Kinetic and isotherm studies on the adsorption–desorption of technical-grade endosulfan in loamy soils under Theobroma cacao L cultivation, Southwestern Nigeria
Received
11th May 2022
, Accepted 28th November 2022
First published on 29th November 2022
Abstract
The fate of pesticides in soils is dependent on the adsorption–desorption kinetics, isotherms and soil types. Interactions of technical-grade endosulfan with top soils (top 0–15 cm) from CRIN, Igba and Sore Bale Theobroma cacao L farms in Southwestern Nigeria were studied using the OECD batch isotherm method. The soils were predominately basic (pH 7.1–8.33), while the orders of the total organic carbon (1.32–2.03%) and clay content (10.92–19.11%) were CRIN > Igba > Sore Bale and Igba > CRIN > Sore Bale, respectively. The adsorption of endosulfan was bi-continuous: initially rapid, followed by a slow process, with pseudo-equilibria and plateaus formed between 120 and 240 min. Endosulfan adsorption by soils was due to their greater affinity for organic matter than clay. The adsorption rates fitted better into a pseudo-second-order model (PSOM) than a pseudo-first-order model (PFOM), with the adsorption (kads) and desorption (kdes) rate constants for both isomers ranging from 7.60 × 10−3 to 11.52 × 10−3 min−1 and 1.39 × 10−3 to 3.42 × 10−3 min−1, respectively (i.e. kads > kdes), while kdes (β-isomer) > kdes (α-isomer) for PFOM, but k2_ads < k2_des for the two isomers in PSOM. Additionally, α-endosulfan was adsorptive, with the β-isomer more prone to leaching; both isomers were moderately leachable according to their FAO mobility rankings. The adsorption model did not fit well into a Langmuir isotherm (R2 ≤ 0.948); however, the desorption model did (R2 ≥ 0.991). Freundlich isotherm plots fitted better (R2 ≥ 0.992) and exhibited non-linear curves of types L and S for the adsorption and desorption processes, respectively. The adsorption/desorption coefficients (Kfads and Kfdes) and strengths of adsorption/desorption (1/nads and 1/ndes) for both isomers were from 1.33 ± 0.10 to 4.81 ± 0.18 μg1−1/n (mL)1/n g−1 and 0.503 to 1.402, respectively, in all soils, with Kfads < Kfdes and 1/nads < 1/ndes. Positive hysteresis was observed. CRIN exhibited the highest hysteresis index. The Kfom values were ≤127.14 ± 6.23 mL g−1, while the values of the standard free energy were ΔG0 = −5.11 to −14.05 kJ mol−1 K−1, depicting a spontaneous physisorption process, driven by van der Waals forces, among others. Endosulfan could easily be leached and contaminate the surface and groundwater owing to its faster PSOM desorption rate constant, but TOM and clay could be used as mitigants to reduce its mobility in soils as they have significant affinity for the pesticide.
Environmental significance
The misapplication of pesticides due to inappropriate and unregulated use by cocoa farmers in West African sub-region is amongst the main cause for their persistence and contamination of farm soils. Therefore the interactions of commercial grade endosulfan pesticide with three pristine Theobroma cacao L farm soils from Nigeria were assessed to decipher its fate and mobility after application without amendment using adsorption-desorption batch model. The studies reveal that soils with high organic matter and high clay inhibited mobility of the pesticides and thus would mitigate their runoff and/or leaching to impact ground water and surface water.
|
1 Introduction
The use of organochlorine pesticides (OCPs) and other agrochemicals in agriculture to control pests in order to enhance productivity has achieved its purpose tremendously. However, their application has brought myriad environmental challenges owing to their persistence, bio-accumulation and toxicity.1,2 OCP contamination of agricultural soils and its impact on groundwater, surface water, aquatic biota and the atmosphere is caused by their leaching, run-off, uptake, volatilization and precipitation after application.3,4
Endosulfan (6,7,8,9,10,10-hexachloro-1,5,5a,6,9,9a-hexahydro-6,9-methano-2,3,4-benzo(e)dioxathiepin-3-oxide) (Fig. 1) is an organochlorine pesticide (OCP) of the cyclodiene subgroup that is used extensively for the control of numerous insects and viruses in both food and non-food crops.5,6 It is a pesticide of choice for Theobroma cacao farmers in West African countries;7 however, it has been listed by the Stockholm Convention as a persistent organic pollutant (POP) and marked for global elimination.8,9 It exists in two stereoisomers: α- and β-endosulfan, making up of 70% and 30% of the technical grade, respectively.10,11 Although, both isomers have been reported to exhibit similar insecticidal properties, they show some different physicochemical properties.12 Endosulfan is ubiquitous and has a propensity to undergo long-range transport.13 This has made it one of the most frequently detected pesticides in the environment and it is often reported in places where it has not been applied.14 It is a persistent organic pollutant (POP) and has a high potential for bioaccumulation in biota.8,10 In the environment, some of its metabolites are endosulfan hydroxyl, lactone, and sulfates.7,15
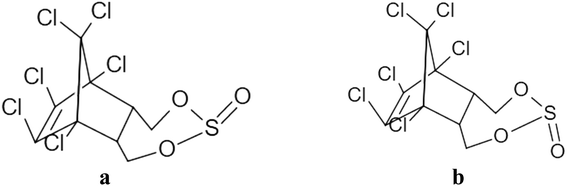 |
| Fig. 1 Chemical structures of (a) α-endosulfan and (b) β-endosulfan. | |
Soil, as that part of the earth that support plants growth, is a ready sink for the used pesticides16 and when they are applied to plants (such as Theobroma cacao trees) through spraying, most of them get on the surrounding matrixes, which consist of fell dried leaves and soils – where the un-volatilized pesticides are eventually washed into the soil.7 The volatilized portion may later precipitate and drop on soil and/or surface water. One of the major processes governing the fate of pesticides in the environment is the retention of pesticides by soils. Pesticide retention in soils is dependent on the mechanism of adsorption and desorption. Adsorption is the adherence of adsorbate (pesticide) molecules to adsorbent (e.g. soil) surfaces through physical interaction (physisorption) and/or through the formation of chemical bonds (chemisorption). A variety of mechanisms are involved when pesticides bind to soils, including: London–van der Waals forces, cation and water bridging, hydrogen bonding, protonation, ligand exchange, covalent bonding, cation and anion exchange, and physical trapping.17–19 Desorption is the reserve process of adsorption and is dependent on the soil–pesticide binding strength. Adsorbates that are initially adsorbed on an adsorbent are then leached into the surrounding medium – usually liquid.20 The phenomenon of soil sorption involving pesticides is of tremendous importance from both an agricultural and environmental perspective. Pesticide sorption influences other processes too, like transport, degradation, volatilization, persistence, bioaccumulation, and biomagnification; these in turn determine the final fate of the pesticide in the soil environment.7,21–23 In addition, soils are heterogeneous mixtures of several components, comprising organic and inorganic compounds with varying compositions and surface activities and when bonded to pesticides, their bioavailability is generally reduced. Thus, a knowledge of the pesticide adsorption–desorption characteristics of soil is necessary for predicting their mobility (leaching and migration), retention, and disappearance, as well as to understand the soil–pesticide dynamics, and whether bioremediation is a feasible option or not for the clean-up of contaminated soils.
Sorption is usually described through isotherm models. Some of the most frequently observed and sited mathematical models in the literature are: the Freundlich isotherm, Langmuir isotherm, BET (Brunauer, Emmett, and Teller) isotherm, and Gibb's isotherm. The Freundlich and Langmuir isotherms are the most commonly used models for solid–aqueous systems, like for activated carbon/natural sorbent (solid) in water/wastewater treatment (aqueous) containing organic compounds.24 However, of the two models, the Freundlich isotherm is the most frequently applied for the adsorption processes that are typical of a heterogeneous surface, which are often observed in soils.25–27
There are numerous reports available on the reaction of pesticides (carbamates, organophosphates, organochlorines) with soil.6,21,28,29 Other hydrophobic organics, like PAHs,16,30 and herbicides, like atrazine, acetochlor, and phenoxyalkanoic acids,31–33 have been reported. Kumar and Philip,6 Atasoy et al.,18 and Qian et al.34 studied the behaviour of endosulfan in four Indian soils: vertisol soils under cotton in a southeast region of Turkey and two typical agricultural soils (latosol and lateritic red soil) in Southwest China, respectively. They investigated the sorption phenomenon of endosulfan in soils and its properties, and also reported on the relationships between the total organic carbon/organic matter, soil pH, soil types, and particle size (clay, loam, silt, sandy) and endosulfan. These researchers asserted that the adsorption and desorption of endosulfan in soils is dependent on the presence and levels of the aforementioned soil properties. In addition these properties are fundamental to the assessment of the quantity of endosulfan that can be transported or leached and also offers guidance for appropriate remediation and mitigation techniques.6 There are also several studies on the sorption of OCPs and other pesticides; however, most studies tend to address only adsorption properties and were conducted under different experimental conditions, thus making a comparison of the adsorption behaviour and properties difficult.29
The application of uncontrolled and large quantities of pesticides may impact the quality of the surrounding soils, surface water, and groundwater. The fate of sprayed endosulfan as a pesticide of choice by Theobroma cacao farmers in the West African sub-region has not yet been extensively examined,7 but would be worth doing so as this sub-region accounts for over 70% of the world production of cocoa beans, with Nigeria presently in fourth position.35 Consequently, this study aimed to (i) investigate the adsorption and desorption behaviours of α-endosulfan and β-endosulfan in commercial grade endosulfan pesticide using three selected farm soils under Theobroma cacao vegetation; (ii) use the Freundlich and Langmuir sorption isotherms and pseudo-first- and pseudo-second-order kinetic models for the isotherm mechanism and kinetic studies to determine the residence time of endosulfan in the soils and to predict it environmental mobility and fate; (iii) attempt to evaluate the sorption–desorption mechanisms of α- and β-endosulfan and find whether they are influenced by their physical or chemical properties in soils. It is pertinent to mention that the adsorption–desorption phenomenon of hydrophobic and non-ionic organic like endosulfan is best assessed by applying kinetic and isotherm models.33
2 Materials and methods
2.1 Soil samples
Representative top soil samples, i.e. from a depth of 0–15 cm, from three (3) geo-referenced Theobroma cacao farms located in Oyo (Cocoa Research Institute of Nigeria (CRIN), Ibadan), Ogun (Sore Bale Cocoa Farming Community, Sore Bale) and Ondo (Igba Cocoa farm, Ondo) States, Southwestern Nigeria were selected for the study. Fig. 2, shows the sampling locations: CRIN – N07°12′ E003°51′; Sore Bale – N07°08′ E003°43′; and Igba – N07°07′E004°53′. Samples were collected randomly at five points within each location. Samples were later composited and allowed to age for a period of 24 months; then, prior to the sorption studies, the samples were air-dried, crushed gently, and sieved through a sieve with a mesh size of 2 mm. The fraction of the soils ≤ 2 mm were collected and used for the study.
 |
| Fig. 2 Sampling locations: CRIN (N07°12′ E003°51); Sore Bale (N07°08′ E003°43′); and Igba (N07°07′ E004°53′) (Source: Google Maps, April, 2022). | |
Table 1 shows a summary of the physicochemical properties of the soil samples used for the study – obtained from previous report by Vaikosen et al.7
Table 1 Physicochemical properties of the tested farm soilsa
Parameters |
CRIN |
Sore Bale |
Igba |
0–15 cm |
0–15 cm |
0–15 cm |
Previously published by Vaikosen et al.7
|
pH |
8.26 |
8.31 |
7.1 |
Total organic carbon (%, w/w) |
2.03 |
1.32 |
1.72 |
Total nitrogen (%, w/w) |
0.14 |
0.11 |
0.14 |
C : N ratio |
15 : 1 |
12 : 1 |
12 : 1 |
Total organic matter (%, w/w) |
3.61 |
2.35 |
3.06 |
C. E. C (meq/100 g) |
25.27 |
17.04 |
23.23 |
![[thin space (1/6-em)]](https://www.rsc.org/images/entities/char_2009.gif) |
Particle-size distribution
|
% Clay |
13.65 |
10.92 |
19.11 |
% Silt |
16.84 |
14.87 |
25.53 |
% Sand |
69.51 |
74.21 |
55.36 |
2.2 Chemicals
Endosulfan standards (α-endosulfan (99.6%) and β-endosulfan (99.9%)) and n-hexane and dichloromethane (DCM) solvents for the extraction were purchased from Sigma-Aldrich (St Louis, USA). Sodium sulfate (anhydrous), calcium chloride (CaCl2), and silica gel 60 extra-pure (60–120 mesh) for the column chromatography were obtained from BDH Limited (Poole, UK). Thiodan EC 35 (technical-grade endosulfan, manufactured by Bessen Chemical Co., Ltd, Nanjing, Jiangsu, China) was purchased from an agro-chemical vendor at Dugbe market, Ibadan, Southwestern Nigeria. The technical-grade endosulfan was composed of α- and β-isomers in a ratio of 70
:
30%, respectively.36
A stock solution of 2500 mg L−1 (i.e. 1750 mg L−1 α-endosulfan and 750 mg L−1 β-endosulfan) was prepared by transferring 143 μL of technical-grade endosulfan (Thiodan 35 EC) into 5 mL of ethanol in a 20 mL volumetric flask. This was made to mark with distilled water.
2.3 Adsorption–desorption studies
OECD 106 and USEPA guidelines for the testing of chemicals (adsorption–desorption using a batch equilibrium) were adopted for the studies.26,27 Studies were conducted separately for three loamy soils cultivated under Theobroma cacao farms in Southwestern Nigeria, namely, CRIN (Cocoa Research Institute of Nigeria), Sore Bale, and Igba cocoa farms.
2.3.1 Experimental design.
Kinetic studies of these soils were carried out with 5 μg mL−1 endosulfan at an equilibrium time of 720 min using the parallel method, while the working concentrations for the isotherm studies for the technical-grade endosulfan ranged from 0.25 (0.175, 0.075) μg mL−1 to 25 (17.5, 7.5) μg mL−1, with the concentrations of α- and β-endosulfan isomers given in the parentheses. Data obtained for the endosulfan isomers were subjected to Freundlich and Langmuir model equations to understand the mechanism of their adsorption–desorption. The adsorption–desorption isotherm curves and values of important variables in the linearized Freundlich and Langmuir equations for both isomers in the soil samples were also determined.
2.3.1.1 Screening for an appropriate soil/test solution ratio, pH, and equilibrium time.
Prior to the more definitive studies, a preliminary investigation was carried out (at 24.0 ± 1.0 °C) on two soil types [i.e. soils with the highest and least organic carbon (OC) content] to ascertain the appropriate soil
:
test solution ratio and equilibrium time to be adopted for the sorption studies. Based on the OC levels in the soils, the following three soil
:
solution ratios (w/v) 1/1, 1/5, and 1/25 were prepared in 25 mL calibrated glass centrifuge tubes and the tests were carried adopting OECD 106 and USEPA procedures.
The preliminary investigation showed a soil to solution (0.01 M CaCl2) ratio of 1/25 was the most appropriate for all the samples. Subsequent experiments were carried out using this ratio.
2.3.2 Adsorption–desorption kinetics.
2.3.2.1 Adsorption kinetic study.
Soil samples (1 g) were weighed into eleven (11) calibrated 25 mL glass centrifuge tubes. To each tube, 22.5 mL of 0.01 M CaCl2 solution and 0.25 mL 0.02% (w/v) NaN3 were added and shaken overnight (12 h) to equilibrate. To the tubes, 50 μL of 2500 mg L−1 technical-grade endosulfan emulsion (stock solution) was added and adjusted with 0.01 M CaCl2 solution to 25 mL to give a concentration of 5000 μg L−1 endosulfan (i.e. 3500 μg L−1 and 1500 μg L−1 of α- and β-endosulfan, respectively). The mixtures were shaken for 12 h with the aid of a GFL 3040 (end-to-end) mechanical shaker at a rate of 22–24 rpm. At intervals of 15, 30, 45, 60, 90, 120, 180, 240, 360, 480, and 720 min, the interactions between the endosulfan and soil samples were stopped by centrifuging at 4500 rpm (3375 g) for 5 min with a Beckmen Coulter Allegra 21 digital centrifuge. The supernatant was gently decanted into a clean flask for extraction and clean-up, while the residue in the tubes were left for the desorption kinetic studies. Table 2 shows a summary of the experimental set-up.
Table 2 Tabulated sorption kinetics experimental set-up for the samples, blank and control with initial concentrations of α- and β-endosulfan in 0.01 M CaCl2 aqueous solution
Experiment/farm soil |
Weight of soil (g) |
Final volume of 0.01 M CaCl2 in tube (mL)a |
Concentration of endosulfan (μg mL−1) |
α-Isomer |
β-Isomer |
The weight per milliliter (wt/mL) of 0.01 M CaCl2 was 1.00 g mL−1 (or specific gravity = 1.00); i.e. endosulfan was partitioned into 25 mL or 25 g 0.01 M CaCl2.
|
CRIN |
1.0 |
25.00 |
87.5 |
37.5 |
Igba |
1.0 |
25.00 |
87.5 |
37.5 |
Sore Bale |
1.0 |
25.00 |
87.5 |
37.5 |
Blank |
1.0 |
25.00 |
— |
— |
Control |
— |
25.00 |
87.5 |
37.5 |
2.3.2.2 Extraction and clean-up.
A 5 mL aliquot (in triplicate) of the supernatant was transferred into a 25 mL separatory funnel, and then extracted first with 10 mL DCM and then twice with 5 mL of DCM. Combined extracts was concentrated to 1 mL under a gentle stream of N2 gas in a hood and transferred into SPE cartridges with silica gel. This was eluted with 10 mL of hexane
:
DCM (3
:
1) at a flow rate of 1.2 mL min−1 and the eluate was evaporated to dryness under a gentle stream of N2 gas. The cleaned residue was redissolved with 1 mL hexane and the amount of pesticide in the aqueous phase was quantified by GC-MS analysis (the amount adsorbed on the soil was computed by the difference).
2.3.2.3 Control and blank.
The control and blank experiments were carried out as described above, with the test medium (0.01 M CaCl2), 50 μL of 2500 mg L−1 of technical-grade endosulfan, and NaN3 without soil samples for the control; while the blank was made with a soil sample (1 g), test medium (0.01 M CaCl2), and NaN3 without the test sample (endosulfan). The extracts were subjected to the same test procedure.
2.3.2.4 Desorption kinetic study.
The parallel method was adopted for the desorption kinetics.26,27 The procedure described for the adsorption kinetics was repeated using the same tubes, except that the decanted supernatants were replaced with an equal volume of 0.01 M CaCl2 and the new mixtures were agitated at the same speed with the end-to-end shaker. The reaction was quenched for each tube corresponding to time intervals of 15, 30, 45, 60, 90, 120, 180, 240, 360, 480, and 720 min (in the adsorption kinetics) by centrifuging. The amount of pesticide in each supernatant was then extracted, cleaned, and quantified by GC-MS.
2.3.3 Adsorption–desorption isotherm studies.
2.3.3.1 Adsorption isotherms/equilibrium studies.
The adsorption isotherm experiments were set up in ten (10) calibrated 25 mL glass centrifuge tubes, each containing 1 g of pre-equilibrated soil samples in 20 mL of 0.01 M CaCl2 solution. Working concentrations of 0.25, 0.50, 1.0, 1.50, 2.5, 5.0, 10.0, 15.0, 20.0, and 25.0 μg mL−1 of endosulfan were prepared by adding an appropriate amount from the endosulfan stock solution (technical grade), and thereafter the tubes were adjusted to 25 mL with 0.01 M CaCl2 solution. Controls and blanks were also set up (as described for the adsorption kinetics). The sample tubes were shaken for 12 h to attain equilibrium. The phases were separated by centrifuging the mixtures at 4500 rpm or (3375g) for 5 min and the aqueous phase was decanted gently. The amount of endosulfan in each supernatant was extracted, cleaned, and quantified by GC-MS.
2.3.3.2 Desorption isotherms/equilibrium studies.
After the completion of the procedure for adsorption isotherm/equilibrium, the aqueous phases were carefully drained off completely from the tubes (0.25, 0.50, 1.0, 1.50, 2.5, 5.0, 10.0, 15.0, 20.0, and 25.0 μg mL−1 endosulfan) and replaced by equal volume of 0.01 M CaCl2 (without test substance) to the 25 mL mark. The new mixtures were agitated again for 12 h and thereafter centrifuged at 4500 rpm for 5 min to separate both phases. The aqueous phase was gently decanted into a glass flask for extraction and clean-up. This was followed by GC-MS quantification of both isomers.
2.4 Gas chromatography mass spectroscopy analysis
Residual levels of α- and β-endosulfan in the test media were quantified using a Thermo-Finnigan Trace GC Ultra system (Waltham, MA, USA) coupled to an ion trap mass spectrometer (MS) (Polaris Q), equipped with an AS-2000 Tray Auto-sampler (Thermoquest), split-less injector, and HP-5MS capillary column (30 m length × 0.25 mm i.d. × 0.25 μm film thickness). The data were processed by Xcalibur software. The operating conditions were as follows: the initial temperature of the oven was held at 80 °C for 5 min, then increased to 200 °C at a rate of 20 °C min−1, and then held there for 5 min – this was followed by an increase to 280 °C at 10 °C min−1, finally holding it there for 2 min. The flow rate of the carrier gas helium (99.99% purity) was kept constant at 1.18 mL min−1. The split-less injection mode was done at 79.5 kPa at a temperature of 250 °C, while the total flow and linear velocity were 32.7 mL min−1 and 10.0 cm s−1, respectively. The interface line and ion source temperatures were 260 °C and 250 °C, respectively.
2.5 Calibration and validation of the analytical method
2.5.1 Calibration curve.
A five-point calibration plot for α- and β-endosulfan in the hexane and isopropyl alcohol mixture (1
:
1) was carried out. The working concentrations of the reference standards used for the calibration ranged from 0.020 to 1.200 μg mL−1. The calibration curves for the α- and β-endosulfan standards were linear over the concentration range applied. The linearity was good, as indicated by the regression coefficients (R2) of 0.9989 and 0.9976 obtained for α- and β-endosulfan, respectively, with corresponding retention times (RTs) at 18.42 and 20.07 min.
2.5.2 Quality assurance.
To validate the method, the standard addition technique was applied for the recovery studies. Solutions of technical-grade endosulfan (containing 3.5 and 1.5 μg mL−1 of α- and β-endosulfan, respectively) were fortified at three concentration levels. Spiking was done using the α- and β-endosulfan standards as follows: 4.0 and 6.0 μg mL−1; 6.0 and 8.50 μg mL−1; 11.50 and 13.50 μg mL−1 respectively. Control and blank experiments were also assayed. The control tube was filled with 0.01 M CaCl2 solution without endosulfan, while the blank was made up of the technical grade endosulfan (3.5 and 1.5 μg mL−1 of α- and β-endosulfan, respectively) in 0.01 M CaCl2 solution without spiking. The method sensitivity was determined by the limit of detection (LoD) and the limit of quantification (LoQ). The LoD and LoQ were obtained by evaluating the lowest concentrations of the analyte that could be detected and measured respectively. They were calculated using the expressions: LoD = 3.3Sa/b and LoQ = 10Sa/b (where Sa is the standard deviation of the intercept of the regression line, and b is the slope of the regression line).37,38 The identified GC-MS peaks for α- and β-endosulfan extracted from aqueous phase were confirmed by selected molecular ion peaks at m/z values using the National Institute of Standards and Technology (NIST) search library.7,39
2.6 Data evaluation
2.6.1 Quantification of the adsorbed endosulfan.
The amount of endosulfan adsorbed onto the soil surface was evaluated from the difference between the initial concentration of endosulfan in solution (C0) and the amount remaining in the aqueous solution at the end of the experiment (Ce). The quantity of endosulfan adsorbed at equilibrium (qe) or at any time t (qt) was calculated in ug g−1 from eqn (1) and (2) as follows: |  | (1) |
|  | (2) |
where qe and qt are the amounts of endosulfan adsorbed onto the soil surface at equilibrium and at time t, respectively; C0 (mg L−1) is the initial concentration of endosulfan in solution, while Ce and Ct (mg L−1) represent the final concentration of endosulfan in the aqueous phase at equilibrium and at time t; v is the volume of solution in mL, and m is the soil mass in g.
2.6.2 Kinetic study and sorption rate constants.
The adsorption (kads) and desorption (kdes) kinetic rate constants for α- and β-endosulfan in the soils were evaluated by the Lagergren pseudo-first-order model40 (PFOM), as expressed in eqn (3) and (4): | 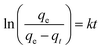 | (3) |
| ln(qe − qt) = ln(qe) − kt | (4) |
Values for In (qe − qt) were calculated for each time amplitude for both isomers at a temperature of 25.0 ± 1 °C, where qe and qt represent the quantity of adsorbate adsorbed at equilibrium and time t, respectively, and k represents the rate constants for the adsorption (kads) and desorption (kdes) processes. The pseudo-second-order kinetics model (PSOM) was evaluated using eqn (5):
|  | (5) |
2.6.3 Isotherm/equilibrium study.
The Langmuir41 and Freundlich42 isotherms have been used for the description of soil/sediment or solid phases with other materials. However, the adsorption–desorption of pesticides with soils and sediments are best described by the non-linear Freundlich isotherm.16,27 The Freundlich adsorption isotherm is generally expressed as follows: | 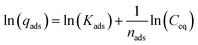 | (7) |
Eqn (6) and (7) are the normal and linearized forms of the Freundlich adsorption isotherm respectively, where qe, is the amount of pesticide adsorbed on the adsorbent (μg g−1), Ce is the equilibrium concentration of pesticide in the solution (μg mL−1), and Kf [(μg g−1)/(μg mL−1)n] and 1/n are empirical constants, referred to as the Freundlich adsorption coefficient and adsorption constant, respectively. Kf and n are obtained by linear regression and characterize the adsorbent and the pesticides adsorbed.
The Langmuir isotherm is expressed as follows:
| 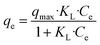 | (8) |
| 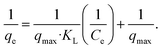 | (9) |
where
qe is the amount of pesticide adsorbed on the adsorbent (μg g
−1),
Ce is the equilibrium concentration of pesticide in the solution (μg mL
−1),
KL is the Langmuir constant, and
qmax is the maximum adsorption capacity (μg g
−1). It is pertinent to mention that the Langmuir isotherm is based on the assumption that there is chemisorption of the adsorbate. It is therefore appropriate for the interaction between an adsorbate and adsorbent leading to a monolayer adsorption.
41
2.7 Leaching potential
Pesticide leachability (or mobility) in the soil profile and the risk of groundwater contamination are often assessed by FAO mobility ranking based on log
Koc values43 and the Groundwater Ubiquity Score, GUS index,44 expressed as: | GUS index = (log t1/2) × (4 − log(Koc)) | (10) |
where t1/2 is the half-life of pesticide and Koc (organic carbon adsorption coefficient) is an empirical value obtained from the Kd and % OC. (The t1/2 of α-endosulfan, β-endosulfan in CRIN soil are 12.16 and 16.75 d, respectively; as earlier published by Vaikosen et al.7).
2.8 Thermodynamic parameter of adsorption: the standard gibbs free energy (ΔG°)
The thermodynamic properties aid describing the energy changes observed in adsorption processes.45 To clarify the sorption mechanism of the endosulfan isomers in the three farm soils, the resultant free energy changes due to the reactants (soil and endosulfan) and products (soil–endosulfan) were evaluated based on the equilibrium adsorption coefficient Kd and the standard Gibbs free energy (ΔG°), as expressed in eqn (10): | ΔG° = −RT In Kd | (11) |
where ΔG° is the standard Gibbs free energy change, R is the gas molar constant (8.314 J mol−1 K−1), Kd if the equilibrium adsorption coefficient, and T is the absolute temperature – at 298 K. Note: in order to have a dimensionless Kd, the volume (mL) of the aqueous phase used was converted to weight (g) by determining its specific gravity (or weight per millilitre, wt/mL). The wt/mL of 0.01 M CaCl2 was approximately 1.00 g mL−1.
3 Results and discussions
3.1 Quality assurance and validation of the analytical method
The analytical performance for the quantification of α- and β-endosulfan was assessed and validated in accordance with ICH guidelines.46 The calibration curves for α- and β-endosulfan standards were linear over the concentration range applied. The linearity was good, as indicated by the regression coefficients (R2) of 0.9989 and 0.9976 obtained for α- and β-endosulfan, respectively. Table 3 shows the values obtained in the recovery studies for both isomers, which ranged from 97.27% ± 1.87% to 100.44% ± 1.16%, with a coefficient of variation (CV) as % RSD ≤ 1.97%, with these values indicating that the method of analysis was highly reliable and reproducible. The LoQ and LoD values were 0.001 μg mL−1 and 0.0003 μg mL−1, respectively; this again showed the good sensitivity of the method applied for quantification of the pesticides.
Table 3 Recovery studies for the adsorption–desorption experiments
Pesticide (isomers) |
Amount of pesticide in solution (μg mL−1) (0.001 M CaCl2) |
Amount of pesticide spiked (μg mL−1) |
Total quantity of pesticide expected (μg mL−1) |
Total quantity of pesticide found (μg mL−1) |
Percentage recovery (%) |
RSD (%) |
SEM |
α-Endosulfan |
3.50 |
4.00 |
7.50 |
7.53 ± 0.06 |
100.44 ± 1.16 |
0.81 |
0.04 |
β-Endosulfan |
1.50 |
6.00 |
7.50 |
7.43 ± 0.04 |
99.02 ± 1.10 |
0.53 |
0.02 |
α-Endosulfan |
3.50 |
6.50 |
10.00 |
9.74 ± 0.19 |
97.37 ± 1.92 |
1.97 |
0.11 |
β-Endosulfan |
1.50 |
8.50 |
10.00 |
9.73 ± 0.07 |
97.27 ± 1.87 |
0.67 |
0.04 |
α-Endosulfan |
3.50 |
11.50 |
15.00 |
14.98 ± 0.06 |
99.84 ± 0.56 |
0.41 |
0.04 |
β-Endosulfan |
1.50 |
13.50 |
15.00 |
14.75 ± 0.07 |
98.33 ± 1.12 |
0.48 |
0.04 |
![[thin space (1/6-em)]](https://www.rsc.org/images/entities/char_2009.gif) |
Control
|
α-Endosulfan |
3.5 |
— |
3.5 |
3.49 ± 0.01 |
99.79 ± 0.26 |
0.01 |
0.01 |
β-Endosulfan |
1.5 |
— |
1.5 |
1.47 ± 0.01 |
97.87 ± 1.22 |
0.03 |
0.01 |
![[thin space (1/6-em)]](https://www.rsc.org/images/entities/char_2009.gif) |
Blank
|
α-Endosulfan |
<0.000 |
— |
<0.000 |
<0.000 |
<0.000 |
<0.000 |
<0.000 |
β-Endosulfan |
<0.000 |
— |
<0.000 |
<0.000 |
<0.000 |
<0.000 |
<0.000 |
3.2 Identification of the endosulfan isomers
Fig. 3 shows the chromatogram for α- and β-endosulfan at retention times of 18.43 and 20.07 min, respectively, with the corresponding m/z spectra below. These m/z spectra patterns were characteristic of endosulfan.15,47 Also, Fig. 4(a–j) present the chromatograms (with peak areas/heights) of both isomers from t0 to t480 − these indicate the amount of residual endosulfan in 0.01 M CaCl2 medium versus the working time amplitude as the soil–endosulfan reaction progressed. The decrease in the amount of residual endosulfan implied an increase in the amount of pesticide adsorbed on the soil samples.
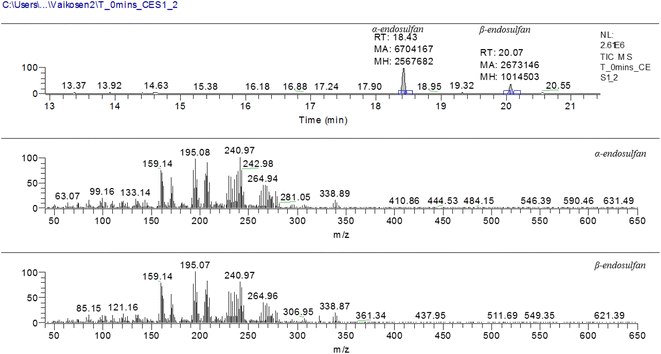 |
| Fig. 3 Chromatograms and mass spectra of α-endosulfan and β-endosulfan. | |
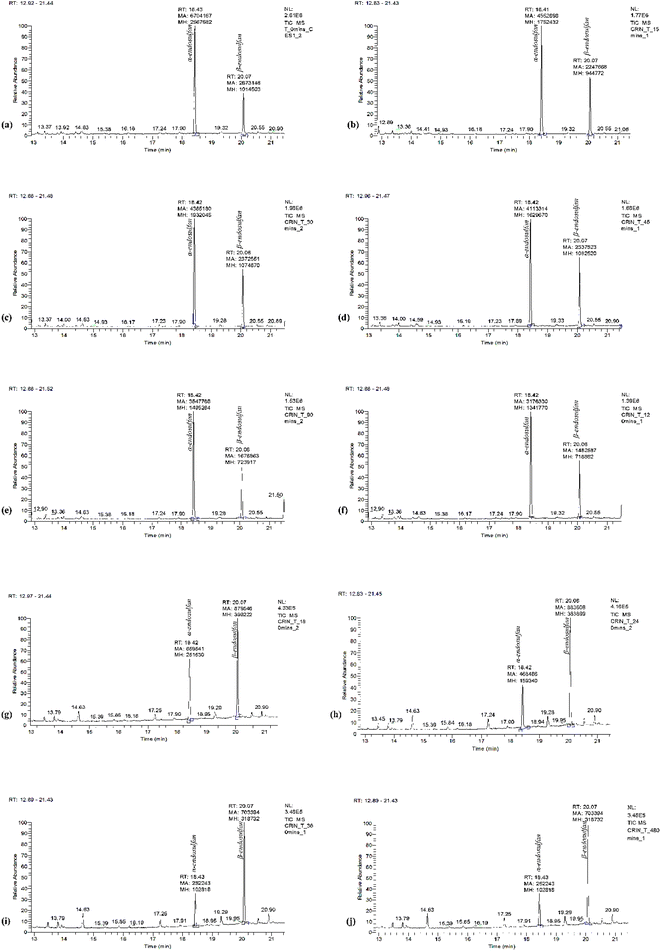 |
| Fig. 4 Chromatograms of the adsorption kinetics for α- and β-endosulfan at (a) T0mins, (b) T15min, (c) T30min, (d) T45min, (e) T90min, (f) T120min, (g) T180min, (h) T240min, (i) T360min, and (j) T480min for CRIN farm soil. | |
3.3 Adsorption–desorption kinetics
Fig. 5–12, show the results obtained for the interaction of endosulfan pesticide with the soils from CRIN, Igba, and Sore Bale Theobroma cacao farms for the PFOM.
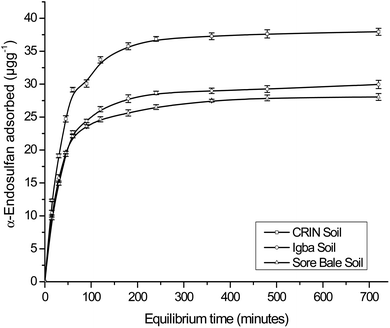 |
| Fig. 5 Adsorption kinetics of α-endosulfan on three different cocoa farm soils. | |
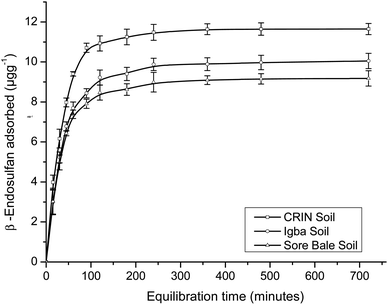 |
| Fig. 6 Adsorption kinetics of β-endosulfan on three different cocoa farm soils. | |
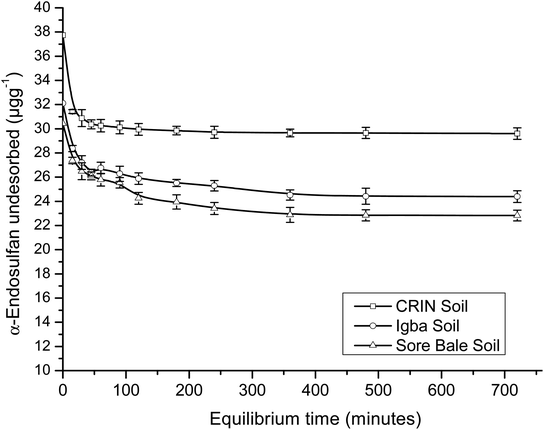 |
| Fig. 7 Desorption kinetics of α-endosulfan for CRIN, Igba and Sore Bale farm soils. | |
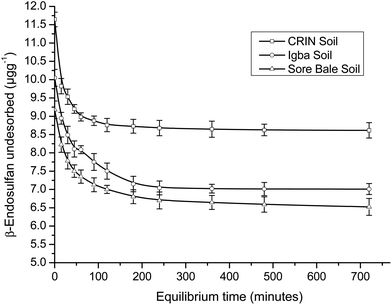 |
| Fig. 8 Desorption kinetics of β-endosulfan for CRIN, Igba and Sore Bale farm soils. | |
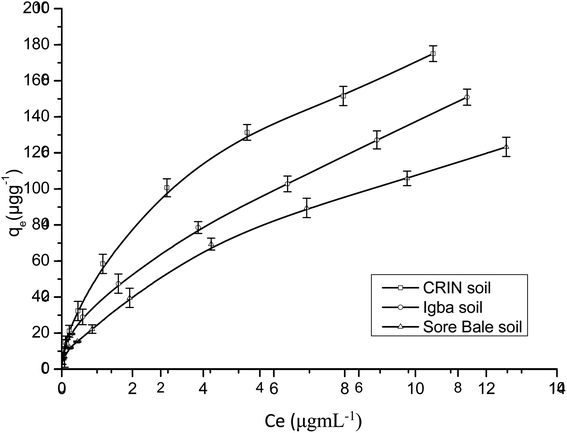 |
| Fig. 9 Adsorption isotherms of α-endosulfan for CRIN, Igba and Sore Bale soils, where: qe = concentration of α-endosulfan adsorbed on soil at equilibrium (μg g−1), Ce = concentration of α-endosulfan remaining in 0.01 M CaCl2 at equilibrium (μg mL−1). | |
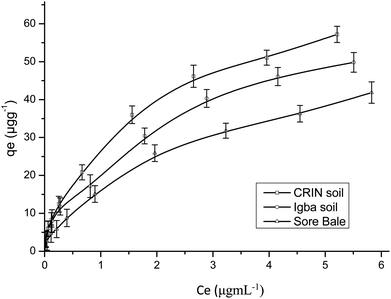 |
| Fig. 10 Adsorption isotherms of β-endosulfan for CRIN, Igba and Sore Bale soils, where: qe = concentration of β-endosulfan adsorbed on soil at equilibrium (μg g−1), Ce = concentration of β-endosulfan remaining into 0.01 M CaCl2 at equilibrium (μg cm−3). | |
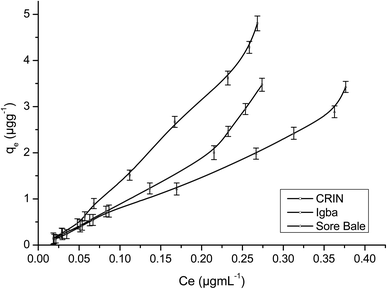 |
| Fig. 11 Desorption isotherms of α-endosulfan for CRIN, Igba and Sore Bale farm soils, where qe = concentration of α-endosulfan undesorbed at equilibrium (μg g−1), Ce = concentration of α-endosulfan desorbed into 0.01 M CaCl2 at equilibrium (μg mL−1). | |
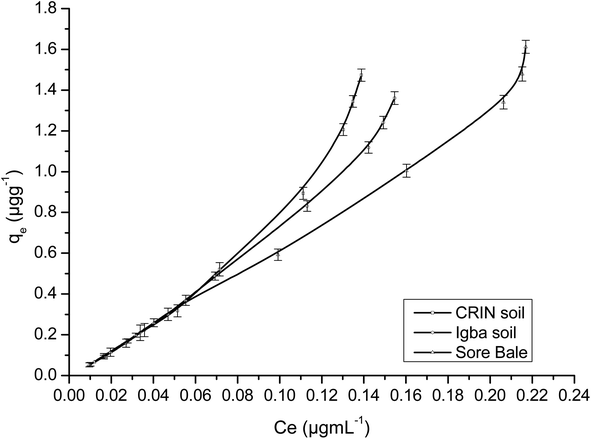 |
| Fig. 12 Desorption isotherms of β-endosulfan for CRIN, Igba and Sore Bale farm soils, where: qe = concentration of β-endosulfan undesorbed at equilibrium (μg g−1), Ce = concentration of β-endosulfan desorbed into 0.01 M CaCl2 at equilibrium (μg mL−1). | |
3.3.1 Adsorption kinetics.
Fig. 5 and 6 show the adsorption kinetics for a nominal equilibration concentration of 5 μg mL−1 technical-grade endosulfan [α- and β-isomers (7
:
3) ratio], which exhibited a rapid and immediate adsorption, with 23.94–43.36% of the equilibration concentration of both isomers adsorbed by the soils under investigation. The percentage adsorption obtained with respect to the equilibration concentration of the pesticides and three soils, in this study were comparable with the range reported for the sorption studies of seven pesticides with sediment from a pond in Teufelseiher, Germany.21 However, this range was significantly different from the values reported by Atasoy et al.18 The incomparable ranges with the latter may have been due to the large differences in the working concentrations and soil profile.
The maximum adsorption capacities for α- and β-endosulfan ranged from 75.64%–78.78% and 76.53–82.21%, respectively, for all the soils in the Theobroma cacao farms within 60 min. This phase was rapid, and it was followed by a slow adsorption process of the adsorbate isomers to the farm soils – this trend depicted a bi-continuous phenomenon.48,49 The aforementioned rapid adsorption was a surface phenomenon, in which vacant sites in the soil were quickly occupied by the non-ionic and hydrophobic endosulfan molecules.6,49 The sudden decrease in the rate of adsorption exhibited in the second phase by the endosulfan isomers was a result of their gradual migration and diffusion into the organic matter matrix and clay mineral interlayers and surfaces.18,21 The same trend was reported by Parkpian et al.50 while studying Rangsit lowland and Phrabat upland soils in Thailand; and also by Kumar and Philip6 and Atasoy et al.18 for four Indian soils and vertisol soils from Turkey, respectively.
The α-isomer attained pseudo-equilibria, with the formation of plateaus after 120, 180, and 240 min with the Sore Bale, Igba, and CRIN farm soils, respectively, while for the β-isomer, plateaus were formed after 120 min with Sore Bale soil and 240 min with CRIN and Igba soils. The pseudo-equilibrium time range obtained for each farm soils in this study (120–240 minutes) was comparable with the time reported by Parkpian et al.50 and Atasoy et al.,18 for both isomers in topsoils. At pseudo-equilibrium, less than a 3% variation of the residual adsorbate concentrations (α- and β-endosulfan) in the aqueous phase was observed between the two successive time amplitudes, even at the terminal time of 720 min. The variation in percentage adsorption was comparable with the values reported for four Indian soils.6
The order of attainment of pseudo-equilibria and the formation of plateaus were as follows: Sore Bale > Igba > CRIN. The relative ease of attaining pseudo-equilibrium exhibited by the Sore Bale soil was due to its relatively low adsorption capacity and percentage adsorption caused by the presence of fewer vacant sites for the α- and β-endosulfan isomers to occupy.
The partition coefficient (Kd) at equilibrium ranged from 13.33 ± 0.57 to 19.51 ± 0.97 mL g−1 and 8.10 ± 0.34 to 11.25 ± 0.23 mL g−1 for α- and β-endosulfan, respectively (Table 4). The order of partitioning was CRIN > Igba > Sore Bale; thus exhibiting the same trend with respect to the percentage adsorption. A high equilibrium Kd value signifies a greater adsorption tendency of the adsorbate to the adsorbent and a lower tendency of the adsorbate to remain in the aqueous phase.
Table 4 Adsorption–desorption pseudo-first-order model (PFOM) and pseudo-second-order model (PSOM) kinetics parameter of α- and β-endosulfan for the CRIN, Igba and Sore Bale farm soilsab
Adsorption kinetics – parameters |
PFOM |
PSOM |
|
A
cp (μg g−1) |
A
t
% |
K
d
(mL g−1) |
K
om (mL g−1) |
k
ads (min−1) |
R
2
|
k
2_ads (g μg−1 min−1) |
R
2
|
Where; Acp = adsorption capacity at 720 min equilibrium time.; A% = adsorption percentage at equilibrium; D% = desorption percentage at equilibrium; Kd = distribution coefficient at adsorption equilibrium; Kdes = distribution coefficient at desorption equilibrium; Koc = distribution coefficient due to organic carbon; kads=adsorption first-order rate constant; kdes = desorption first-order rate constant; k2_ads = adsorption second-order rate constant; k2_des = desorption second-order rate constant; R2 = correlation coefficient. Note: Kd was calculated using the expression from OECD (2000)26 and USEPA (2008).27
where; Cs = concentration in soil; Caq = concentration in aqueous medium.
Partitioning of endosulfan (μg) between soil (1 g) and 0.01 M CaCl2 (25 g) since wt/mL of 0.01 M CaCl2 was approx. 1.00 g mL−1. Unit for Cs and Caq the same (μg g−1) will result in a unit-less Kd and ΔG° could be calculated.
|
CRIN farm soil
|
α-Endosulfan |
37.94 ± 1.09 |
43.36 |
19.15 ± 0.57 |
547.19 ± 23.31 |
9.67 × 10−3 |
0.933 |
71.59 ± 1.88 |
0.997 |
β-Endosulfan |
11.94 ± 0.17 |
31.85 |
11.25 ± 0.23 |
321.45 ± 19.57 |
12.36 × 10−3 |
0.895 |
6.92 ± 0.57 |
0.999 |
![[thin space (1/6-em)]](https://www.rsc.org/images/entities/char_2009.gif) |
Igba farm soil
|
α-Endosulfan |
29.96 ± 0.29 |
34.24 |
14.5 ± 0.12 |
488.99 ± 31.91 |
7.60 × 10−3 |
0.974 |
43.47 ± 1.41 |
0.999 |
β-Endosulfan |
10.05 ± 0.11 |
26.80 |
9.15 ± 0.06 |
308.57 ± 21.46 |
9.67 × 10−3 |
0.931 |
5.03 ± 0.49 |
0.998 |
![[thin space (1/6-em)]](https://www.rsc.org/images/entities/char_2009.gif) |
Sore bale farm soil
|
α-Endosulfan |
28.04 ± 0.30 |
32.05 |
13.33 ± 0.05 |
585.54 ± 35.34 |
9.91 × 10−3 |
0.990 |
44.57 ± 1.55 |
0.999 |
β-Endosulfan |
8.98 ± 0.08 |
23.94 |
8.10 ± 0.04 |
355.94 ± 23.34 |
11.52 × 10−3 |
0.958 |
5.47 ± 0.59 |
0.999 |
Desorption kinetics – parameters |
|
D
cp (μg mL−1) |
D
t
% |
K
des (mL g−1) |
K
om (mL g−1) |
k
des (min−1) |
R
2
|
k
2_des (g μg−1 min−1) |
R
2
|
![[thin space (1/6-em)]](https://www.rsc.org/images/entities/char_2009.gif) |
CRIN farm soil
|
α-Endosulfan |
0.12 ± 0.01 |
7.92 |
209.7 ± 10.56 |
2600.21 ± 54.24 |
1.39 × 10−3 |
0.821 |
754.6 ± 25.5 |
1.000 |
β-Endosulfan |
0.06 ± 0.00 |
14.89 |
142.9 ± 8.78 |
2028.73 ± 51.19 |
2.69 × 10−3 |
0.922 |
32.94 ± 3.83 |
1.000 |
![[thin space (1/6-em)]](https://www.rsc.org/images/entities/char_2009.gif) |
Igba farm soil
|
α-Endosulfan |
0.12 ± 0.01 |
10.15 |
221.2 ± 12.02 |
2664.17 ± 61.52 |
1.79 × 10−3 |
0.959 |
449.8 ± 16.8 |
1.000 |
β-Endosulfan |
0.07 ± 0.00 |
16.32 |
128.2 ± 9.98 |
2141.45 ± 41.21 |
2.98 × 10−3 |
0.906 |
31.18 ± 2.72 |
1.000 |
![[thin space (1/6-em)]](https://www.rsc.org/images/entities/char_2009.gif) |
Sore Bale farm soil
|
α-Endosulfan |
0.14 ± 0.01 |
11.25 |
197.2 ± 13.83 |
3295.72 ± 67.09 |
2.04 × 10−3 |
0.939 |
450.4 ± 16.7 |
1.000 |
β-Endosulfan |
0.07 ± 0.00 |
18.53 |
109.99 ± 8.87 |
2702.49 ± 69.17 |
3.42 × 10−3 |
0.957 |
33.01 ± 4.08 |
1.000 |
A comparison of the R2 values of the PFOM with that of the PSOM (Table 4) showed a higher value, which indicated the better fitness for the latter. The adsorption and desorption kinetics fitted perfectly into PSOM, which may imply that the rate was dependent on the concentration of both the adsorbent and the adsorbate.
3.3.2 Desorption kinetics of α- and β-endosulfan.
The degree of mobility of a chemical compound in soil can be assessed by desorption studies. Fig. 7 and 8 show the kinetic desorption of both isomers of endosulfan from the soils. The α- and β-endosulfan exhibited a rapid desorption kinetics, with apparent desorption capacities of α-endosulfan at the terminal equilibrium time (720 min) for CRIN, Igba, and Sore Bale soils of 0.12 ± 0.01 (7.92%), 0.12 ± 0.01 (10.15%), and 0.14 ± 0.01 μg mL−1 (11.25%), respectively (with the percentage desorption given in the parentheses); while the values for the β-isomer were 0.06 ± 0.00 (14.89%), 0.07 ± 0.01 (16.32%), and 0.07 ± 0.01 μg mL (18.53%), respectively. The relative ease of endosulfan desorption from the soils at this initial stage was in the order: Sore Bale > Igba > CRIN. This trend was in reverse with respect to the adsorption kinetics. The loss of both isomers was immediate and rapid. This may have been due to the particle-size distribution of the farm soils. Krishna and Philip29 reported a 50% desorption of pesticides (lindane, carbofuran, and methyl parathion) within 15 min from a sandy soil. The percentage of sand content in the farm soils here were 71.21%, 69.51%, and 55.36% for Sore Bale, CRIN, and Igba, respectively. The rapid release of the endosulfan isomers from the soils continued until pseudo-equilibrium was reached, followed by a slow desorption process of the two adsorbate isomers. Pseudo desorption equilibria in CRIN, Igba, and Sore Bale soils for α- and β-endosulfan were attained within 180–240 min and 120–180 min, respectively. The β-isomer showed a greater desorption tendency than the α-isomer, hence exhibiting a faster desorption rate. This relative ease of being leached from the farm soils may be one of the reasons the β-isomer is predominant in the aquatic environment.51,52
3.3.3 Sorption rate constants.
The adsorption (kads) and desorption (kdes) rate constants for both PFOM and PSOM are presented in Table 4. The adsorption rate constants (kads) for α- and β-endosulfan evaluated from the Lagergren plot varied from 7.60 × 10−3 to 9.91 × 10−3 min−1 and 9.67 × 10−3 to 11.52 × 10−3 min−1, respectively. The regression coefficients (R2) for all the plots ranged between 0.895 and 0.990. The order for the kads values were Igba < CRIN < Sore Bale and CRIN < Igba < Sore Bale for α- and β-endosulfan, respectively. This trend implied that the Sore Bale soil would attain adsorption equilibrium fastest due to its relatively low adsorption capacity because of its low percentage clay and organic carbon content.6,21 Also, the kdes for α- and β-endosulfan ranged from 1.39–1.70 × 10−4 min−1 and 2.24–2.85 × 10−4 min−1, respectively, the order being CRIN < Igba < Sore Bale for both isomers. The calculated desorption rate constants (kdes) were generally higher for the β-isomer than those of the α-isomer in all the soils. This implied that the β-isomer would have a greater tendency to be leached from soil during run-off and also would migrate to contaminate both surface and ground water.53 These rate constants were comparable with the values reported for endosulfan isomers in four Indian soils.6 Also, this study showed that kads > kdes for both isomers, which indicated that the adsorption of endosulfan proceeded much faster than in the desorption exercise, and thus it would likely be retained.
Conversely, for PSOM, the obtained rate constants (Table 4) showed that k2_des > k2_ads. This implied that when the concentrations of the adsorbate and adsorbent were factored in, the rate of desorption was faster than the rate of adsorption. This was the inverse of the trend obtained in the PFOM. Since the kinetics fitted better into the PSOM than the PFOM, it may deduced that there was high mobility and a leaching rate tendency of the endosulfan owing to the hydrophilicity of the soil. This thus implied that the pesticide may not persist for long in farm soils, which may indicate a short term impact but no potential immediate contamination risk unless degraded. The α-endosulfan was observed to adsorb and desorb faster than the β-endosulfan, which was opposite of the trend observed in PFOM. CRIN soil had faster adsorption and desorption kinetics, while the Igba and Sore Bale soil kinetics had comparable rate constants (Table 4) for both adsorption and desorption studies of both isomers.
3.4 Adsorption–desorption isotherms
3.4.1 Adsorption isotherm.
The data obtained in the adsorption equilibrium study for α- and β-isomers at the temperature of 25 ± 1 °C fitted the Freundlich isotherm better (R2 ≥ 0.992) for the isomers and soils (Table 5), but the adsorption data were considered not to fit as well into the Langmuir isotherm (R2 ≤ 0.948) as they did for the Freundlich. The desorption data also fitted well into the Freundlich isotherm. All the Freundlich isotherm plots were non-linear, while the isotherm curves were type L.54 Type L is typical for adsorbents with a high affinity for adsorbates.18 Endosulfan is hydrophobic by nature and would be strongly attracted to soil surfaces that are highly heterogeneous in an aqueous environment.
Table 5 Adsorption–desorption isotherm constants for α- and β-endosulfan in the cocoa farm soilsa
Freundlich constants for the adsorption isotherm and standard Gibbs free-energy change* |
|
K
fads (μg1−1/n (mL)1/n g−1) |
1/nads |
K
foc (mL g−1) |
K
fom (mL g−1) |
ΔG° (kJ mol−1) |
R
2
|
Where; Kfads = Freundlich adsorption coefficient; Kfdes = Freundlich desorption coefficient; KL_ads = Langmuir adsorption coefficient; KL_des = Langmuir desorption coefficient; qmax_ads = maximum adsorption capacity; qmax_des = maximum desorption capacity; 1/nads = Freundlich exponent for adsorption (nads – adsorption intensity); 1/ndes = Freundlich exponent for desorption (ndes – desorption intensity); Kfaoc = organic carbon normalized adsorption coefficient; Kfaom = organic matter normalized distribution coefficient; log Koc = logarithm of Freundlich coefficient due to organic carbon; log Kf = logarithm of Freundlich adsorption/desorption coefficient or capacity; ΔG° = standard Gibbs energy (kJ mol−1); R2 = coefficient of determination. ΔG° calculated from Kd in Table 4.
|
CRIN farm soil
|
α-Endosulfan |
4.50 ± 0.22 |
0.535 ± 0.02 |
221.47 ± 10.83 |
127.14 ± 6.23 |
−7.31 |
0.998 |
β-Endosulfan |
2.33 ± 0.19 |
0.524 ± 0.01 |
114.74 ± 9.36 |
65.87 ± 4.01 |
−6.09 |
0.998 |
![[thin space (1/6-em)]](https://www.rsc.org/images/entities/char_2009.gif) |
Igba farm soil
|
α-Endosulfan |
3.64 ± 0.13 |
0.519 ± 0.02 |
211.62 ± 7.56 |
121.48 ± 7.32 |
−6.36 |
0.999 |
β-Endosulfan |
2.13 ± 0.11 |
0.503 ± 0.02 |
123.95 ± 5.42 |
71.1 ± 5.74 |
−5.49 |
0.997 |
![[thin space (1/6-em)]](https://www.rsc.org/images/entities/char_2009.gif) |
Sore Bale farm soil
|
α-Endosulfan |
2.31 ± 0.08 |
0.557 ± 0.01 |
175.24 ± 6.06 |
100.60 ± 6.11 |
−6.11 |
0.992 |
β-Endosulfan |
1.33 ± 0.10 |
0.547 ± 0.02 |
101.06 ± 7.57 |
58.012 ± 4.88 |
−5.11 |
0.994 |
Freundlich constants for the desorption isotherm |
|
K
fdes (μg1−1/n (mL)1/n g−1) |
1/ndes |
K
foc (mL g−1) |
K
fom (mL g−1) |
ΔG° (kJ mol−1) |
R
2
|
CRIN farm soil
|
α-Endosulfan |
4.81 ± 0.18 |
1.299 ± 0.03 |
237.14 ± 8.87 |
136.13 ± 8.72 |
−14.05 |
0.998 |
β-Endosulfan |
3.35 ± 0.09 |
1.335 ± 0.05 |
165.17 ± 4.43 |
94.82 ± 5.35 |
−12.29 |
0.993 |
![[thin space (1/6-em)]](https://www.rsc.org/images/entities/char_2009.gif) |
Igba farm soil
|
α-Endosulfan |
3.84 ± 0.15 |
1.176 ± 0.01 |
223.21 ± 8.72 |
128.13 ± 6.15 |
−13.38 |
0.995 |
β-Endosulfan |
3.16 ± 0.09 |
1.239 ± 0.06 |
183.52 ± 5.23 |
105.35 ± 5.25 |
−12.02 |
0.999 |
![[thin space (1/6-em)]](https://www.rsc.org/images/entities/char_2009.gif) |
Sore Bale farm soil
|
α-Endosulfan |
3.49 ± 0.12 |
1.171 ± 0.03 |
264.16 ± 12.09 |
151.64 ± 6.32 |
−13.09 |
0.994 |
β-Endosulfan |
2.51 ± 0.13 |
1.076 ± 0.04 |
190.08 ± 9.85 |
109.12 ± 4.68 |
−11.65 |
0.997 |
Langmuir constants |
Adsorption isotherm |
Desorption isotherm |
K
L_ads (L mg−1) |
q
max_ads (μg g−1) |
R
2
|
K
L_des (L mg−1) |
q
max_des (μg g−1) |
R
2
|
CRIN farm soil
|
α-Endosulfan |
0.021 ± 0.001 |
0.093 ± 0.006 |
0.905 |
0.017 ± 0.005 |
0.283 ± 0.011 |
0.991 |
β-Endosulfan |
0.052 ± 0.007 |
0.064 ± 0.008 |
0.928 |
0.020 ± 0.004 |
0.319 ± 0.015 |
0.999 |
![[thin space (1/6-em)]](https://www.rsc.org/images/entities/char_2009.gif) |
Igba farm soil
|
α-Endosulfan |
0.023 ± 0.005 |
0.126 ± 0.010 |
0.922 |
0.010 ± 0.002 |
0.509 ± 0.012 |
0.994 |
β-Endosulfan |
0.053 ± 0.008 |
0.075 ± 0.008 |
0.948 |
0.017 ± 0.006 |
0.370 ± 0.023 |
1.000 |
![[thin space (1/6-em)]](https://www.rsc.org/images/entities/char_2009.gif) |
Sore Bale farm soil
|
α-Endosulfan |
0.027 ± 0.001 |
0.202 ± 0.012 |
0.888 |
0.006 ± 0.001 |
0.886 ± 0.032 |
0.992 |
β-Endosulfan |
0.069 ± 0.004 |
0.109 ± 0.010 |
0.890 |
0.013 ± 0.004 |
0.561 ± 0.026 |
0.997 |
The 1/nads Freundlich exponent of sorption for both isomers ranged from 0.503 to 0.557 (i.e. < 1.0) for all soils, with this range depicting non-linearity. The results showed that 1/nads (α-isomer) > 1/nads (β-isomer) for all three soils. The low 1/nads values in this study also indicated that endosulfan adsorption was normal and not cooperative.55 Small 1/nads values are associated with adsorbents that have greater heterogeneity, with some good examples being soils and sediments with high OM.51,56 The OM content in the three loamy farm soils were significantly high [2.35–3.61% (w/w)], with the values for nads – the strength of the adsorption (reciprocal of 1/nads) – ranging from 1.097 to 1.402 for both isomers. The calculated nads values between 1.0 and 10.0 suggested a good adsorption process;57 hence all the soils were good adsorbents.
The Langmuir maximum adsorption capacities (qmax) of the soils were obtained in the range of 0.064–0.202 μg g−1 for adsorption and 0.282–0.886 μg g−1 for desorption. β-Endosulfan had a lower qmax than α-endosulfan in all instances, except in the desorption data of CRIN. The Langmuir isotherm equilibrium constant (KL) showed low values of 0.021–0.070 L mg−1 for the adsorption capacity. The equilibrium constants for the desorption of endosulfan were found to be 0.006–0.020 L mg−1. The value of KL < 1 indicated that the rate of adsorption was lower than the rate of desorption, which supported the trend of results obtained in the PSOM.
Fig. 9 and 10 are separate plots for α- and β-endosulfan of the adsorbed concentration (μg g−1) versus the equilibrium concentration (μg mL−1), which showed a steep rise at low concentrations (0.175–1.050 μg mL−1 for α-endosulfan; 0.075–0.450 μg mL−1 for β-endosulfan), followed by a parabolic increase at higher concentrations (1.750–17.500 μg mL−1 for α-endosulfan; 0.750–7.500 μg mL−1 for β-endosulfan) in all the soil samples investigated. The initial steep rise in the low concentration range may have been due to the availability of abundant vacant sites in the adsorbent material (i.e. the soil samples), competed for by small quantities of the adsorbate (i.e. α- and β-endosulfan) in the aqueous phase; while the parabolic curve may have resulted from the gradual reduction in the number of vacant sites available for adsorption by the adsorbate as its concentration increased. The behaviour of both isomers were almost similar in all the soils, except for β-endosulfan, which exhibited a more ‘parabolic shape’ in the high concentration range, which was likely due to the more competitive nature of the α-isomer over the β-isomer. The α-isomer may have been favoured because of its rapid rate of adsorption6,34 and relatively higher proportion in the commercial endosulfan36 that was used in this study. The aforementioned may also be one of the reasons why the β-isomer is more predominant in the aquatic environment. The Freundlich adsorption coefficients (Kfads) for α-endosulfan were 4.50 ± 0.22 (CRIN), 3.64 ± 0.13; (Igba), and 2.31 ± 0.08 (μg1−1/n (mL)1/n g−1) (Sore Bale), while the same trend was exhibited by the β-isomer, with values ranging from 2.51 ± 0.13 to 3.35 ± 0.09 (Table 5).
3.4.1.1 Effect of the organic matter and clay content on adsorption.
Endosulfan is non-ionic and hydrophobic and would absorb strongly on the organic components present in soil.50 The total organic matter (TOM) content in the soils were: Sore Bale – 2.35% (w/w), Igba – 3.06% (w/w), and CRIN – 3.61% (w/w) (Table 1). Soils with a heavy texture and containing not less than 1% (w/w) organic matter have been reported to have been used to predict their adsorption capacity.58 The order of Freundlich adsorption capacity (Kads) was CRIN > Igba > Sore Bale (Table 5) – this trend corroborates the earlier assertion by Parkpian et al.50 The Kads values of a chemical with different sorbents are directly proportional to their organic matter or organic carbon content.20 The adsorption capacities exhibited by α- and β-endosulfan in this study would retard their downward migration and leaching within these soil profiles. It is therefore expected that the order of ease of mobility and leaching will be in the reverse order, i.e.: CRIN < Igba < Sore Bale. Si et al.59 reported that the leaching potential of a pesticide is inversely related to adsorption, while its mobility in soil is controlled by sorption. In heterogenous adsorbents like soil, the sorption of non-ionic and hydrophobic organics are dependent on the level of organic matter and, to some extent, on the clay mineralogy of each soil,16,60 and these both influence the mobility.61,62
The order of clay content was: Igba > CRIN > Sore Bale, while the order of organic matter was: CRIN > Igba > Sore Bale. The latter trend was observed when the adsorption capacities of the three cocoa farm soils for α- and β-endosulfan were considered, thus suggesting a greater affinity of endosulfan to TOM than to clay. Although, Torrents and Jayasundera63 in their sorption study of non-ionic pesticides reported that the intensity of sorption was a function of herbicide and clay content, the differentials in the clay and organic matter components between the Igba (19.11%, 3.06%) and CRIN (13.65%, 3.61%) top soils were 5.46% and 0.55% for clay and organic matter content respectively. Despite the minimal difference of 0.55% (w/w) TOM content in favour of the CRIN soil, a much greater adsorption capacity was recorded by the CRIN soil compared to the Igba soil, with 5.46% (w/w) more clay. This affirmed the findings of Krishna and Philip,29 who reported that organic matter seems to have a high affinity to lindane, carbofuran, and methyl parathion pesticides compared to clay. In addition, Huang et al.64 reported that if the organic matter of sediment and soil is less than 0.1% (w/w), the clay and silt content will be mainly responsible for the adsorption of pesticides. Therefore, the contribution of TOM to the sorption of endosulfan in this study was much more relevant than the contributions of the clay and other components.
The Kfom values of α- and β-endosulfan, calculated from the Freundlich adsorption coefficient (Kfads),27,65 were 127.14 ± 6.23 and 65.87 ± 4.01 mL g−1; 121.48 ± 7.32 and 71.1 ± 5.74 mL g−1; 100.60 ± 6.11 and 58.012 ± 4.88 mL g−1 for the CRIN, Igba, and Sore Bale soils, respectively. The Kfom values for the α-isomer were higher than those for the β-isomer for all three soils. The order was CRIN > Igba > Sore Bale for α-endosulfan, while for β-endosulfan, it was Igba > CRIN > Sore Bale.
The Kfom values of pesticides with soils have been used to predict their mobility, and according to Swann et al.,66 pesticides with Kfom values < 500 are considered as mobile with respect to leaching. The highest Kfom value obtained in this study was 127.14 ± 6.23 mL g−1 (α-isomer, CRIN); this indicated that endosulfan had a very high potential to contaminate surface and ground water in the three cocoa farm soils and the β-isomer could be expected to have a greater contamination potential over the α-isomer. Again, this corroborated why the β-isomer is more predominant in the aquatic environment. In addition, it also implied that high levels of organic content in soils could be used to mitigate the migration and mobility of endosulfan in the contamination of surface water and groundwater.67,68 According to the FAO criteria for leachability assessment,43 both isomers were ranked moderately mobile in the soils under investigation; however, the GUS index classified them as nonleachers.44
The calculated ΔG° values ranged from −5.11 to −14.05 kJ mol−1 K−1 for both isomers and the adsorption–desorption processes (Table 5). Yu et al.33 reported that values between 0 and −20 kJ mol−1 K−1 imply that physical adsorption was occurring, with van der Waals forces playing the dominant role among others, which may result in the adsorption effect being small and desorption occurring readily. The aforementioned trend was observed in this study. In addition, the composition of the technical-grade endosulfan (70
:
30) favoured the α-isomer, thus making its molecules more available for a fixed number of binding sites in the soil samples.
3.4.2 Desorption isotherm.
The Freundlich desorption parameters obtained for α- and β-endosulfan are shown in Table 5, where all the isotherm equilibrium plots of the undesorbed concentrations (Cqeuds; sorbed pesticides after the desorption exercise) versus desorbed concentrations (Cedes; in the aqueous phase) of both isomers were non-linear and could be fitted to the Freundlich model (Fig. 11 and 12). The coefficients of determination, R2, for the Freundlich equation were ≥ 0.992. The desorption isotherm curves for both isomers had an S-shape (subgroup 1)54 for all the farm soils, with a slight concavity in the middle stage of the isotherm. The concavity was more pronounced with the β-isomer, which exhibited a greater rate of desorption than the α-isomer. This significant difference may be due to β-endosulfan being slightly more soluble than α-endosulfan in aqueous media,69 and at a pH and temperature of 7.2 and 22 °C, respectively. Also, as the equilibration concentrations used for the adsorption process increased, the amount of desorbed pesticide did not follow a proportional increase, but rather there was retardation and disproportionate increase in the desorbed endosulfan. The Freundlich isotherm model with such a graphical concave curvature has been reported to have 1/ndes > 1.70,71 The values for 1/ndes (i.e. Freundlich exponent of desorption) for α- and β-endosulfan were 1.299 and 1.335 for the CRIN soil, 1.176 and 1.239 for the Igba soil, and 1.171 and 1.076 for the Sore Bale soil, respectively; these values depicted a non-linearity of the desorption data and indeed the nature of the isotherm curve. These values tended to agree with those reported by Atasoy et al.18 for both isomers in vertisol soil from a southeast region of Turkey.
3.5 Soil sorption hysteresis
3.5.2 Endosulfan reversibility in soils and the hysteresis index (HI).
Desorption is critical in assessing the extent to which pesticides are released from soil; that is, the reversibility of the adsorbed pesticide. The reversibility of adsorbed pesticides could be evaluated from the Freundlich adsorption coefficient (Kfads) and desorption coefficient (Kfdes) or Freundlich adsorption constant (1/nads) and desorption constant (1/ndes). Hysteresis is a normal phenomenon in pesticide sorption studies.21,72–74 Positive hysteresis occurred for α- and β-endosulfan in all three soils (i.e. Kfads < Kfdes and 1/nads < 1/ndes). The differences between the Freundlich adsorption and desorption coefficients were remarkable for both isomers (Table 5). Hysteresis is exhibited when there is an increase in the difference between the adsorption and desorption isotherm slopes.75–77 The magnitude or degree of hysteresis (ω) is often expressed as the hysteresis index (HI).65,78 According to Seybold and Mersie,65 this is the ratio of the Freundlich adsorption and desorption exponents 1/nads and 1/ndes. | ω = {[1/nads : 1/ndes] × 100} | (12) |
The HI for α-endosulfan ranged between 38.16% and 47.04%, while it was between 39.02% and 49.84% for β-endosulfan (Table 7). Among the three soils, CRIN had the lowest HI for both isomers, with the order being: Sore Bale > Igba > CRIN. However, Huang et al.79 defined HI as residual–concentration–specific relative sorption–desorption and expressed it as:
|  | (13) |
where
qseandqde are the solid-phase solute (pesticide) concentration for single-cycle sorption and desorption, respectively, while
T and
Ce are the temperature and residual solution-phase concentration. The HI values ranged from 0.2129 to 0.2558 and 0.1335 to 0.2257 for α- and β-endosulfan, respectively. The Sore Bale soil exhibited the least hysteresis for both isomers. There was poor correlation between the HI values of the aforementioned definitions with respect to α-endosulfan (
R2 = 0.4100), while the relationship was very strong for β-endosulfan (
R2 = 0.9991). Langaro
et al.,
80 Bao
et al.,
81 and Adeola
et al.82 all determined HI using Freundlich exponents for a single-step desorption. However, the latter expression is believed to be the most appropriate, acceptable, and valid for soil HI quantification for one-step desorption.
79,83 The Freundlich exponents are used when a desorption experiment is carried out in multiple steps.
83 Besides, these two approaches, three other empirical indices for hysteresis quantification have been reported.
83 The desorption of the sorbed endosulfan from the soil samples was most difficult with the CRIN soil, followed by the Igba and Sore Bale soils. This order was reflective of the differences observed in the type of isotherm curves and slopes between adsorption and desorption processes in this study (
Fig. 11 and
12) (
Table 5). The varying isotherms exhibited by the adsorbate (endosulfan) may be due to the following: the extent of its binding with OM, hydrogen-bond formation, charge transfer, ionic bonds, cation bridges, hydrophobic interactions, and physical diffusion into humic substances in the different soils.
60,62,75 The hysteresis order was directly proportional to the level of organic matter in these three soils; thus making organic matter the prime factor in the hysteresis of endosulfan over the clay content and other factors. Low HI values are associated with adsorbates with a poor desorption property.
84,85 The difference in HI between the α- and β-isomers for each of the three soils was almost insignificant or minimal. However, β-endosulfan exhibited a higher hysteresis over α-endosulfan in CRIN, while the reverse was observed for the Sore Bale and Igba soils, where α-endosulfan exhibited a slightly higher hysteresis than β-endosulfan.
Table 7 Hysteresis index (HI) for the CRIN, Igba and Sore Bale soils
Soil/pesticide |
1/nads : 1/ndesa |
[1/nads : 1/ndes] × 100b |
q
de − qse/qseT,Cec |
HI was calculated according to O'Connor et al.,78
HI was calculated according to Seybold & Mersie,65
HI was calculated according to Huang et al.79
|
α-isomer
|
CRIN |
0.3816 |
38.16 |
0.2131 |
Igba |
0.3918 |
39.16 |
0.2558 |
Sore Bale |
0.4704 |
47.04 |
0.2129 |
![[thin space (1/6-em)]](https://www.rsc.org/images/entities/char_2009.gif) |
β-isomer
|
CRIN |
0.3922 |
39.22 |
0.2257 |
Igba |
0.4060 |
40.60 |
0.2099 |
Sore Bale |
0.4984 |
49.84 |
0.1335 |
4 Conclusion
The adsorption–desorption of technical-grade endosulfan (α- and β-isomers (70
:
30)) on top soils from three Theobroma cacao L farms in Southwestern Nigeria were studied using an OECD/USEPA batch equilibrium isotherm method. Both isomers attained pseudo-equilibria, with the formation of plateaus within an equilibrium time range of 120 and 240 min for all the farm soils. There was a very rapid initial adsorption phase, followed by a slow process as equilibrium was approached. The order of attainment of the pseudo-equilibria and plateau formation were as follows: Sore Bale > Igba > CRIN. The order of partitioning was CRIN > Igba > Sore Bale, with the CRIN soil possessing more vacant sites for the adsorption of endosulfan. Study reaffirmed that organic matter does have higher affinity and predominance over clay in the adsorption–desorption of hydrophobic pesticides such as endosulfans. High levels of TOM and clay in soils could be used to mitigate the migration and mobility of endosulfan to contaminate surface water and groundwater. There was no direct proportionality between the amounts of desorbed pesticide and increasing equilibration concentrations; rather, a retardation and disproportionate increase in the desorbed endosulfan was observed. The study showed that the rate of adsorption assuming a PFOM was greater than the rate of desorption (kads > kdes), while the reverse was the case for PSOM (k2_des > k2_ads). This implied that there will be higher mobility and a higher leaching rate of the endosulfan in applying the latter. The Freundlich isotherm model was the most appropriate model for soil compared to the Langmuir, due to its heterogeneous nature. The data obtained in the adsorption equilibrium study at the temperature of 25 ± 1 °C fitted the Freundlich isotherm (R2 ≥ 0.992) better than the Langmuir isotherm (R2 ≤ 0.948) for both the isomers and soils, while the desorption data fitted well for both isotherms (R2 ≥ 0.991). All the plots were non-linear; the curves were of types L and S for the adsorption and desorption processes, with the β-isomer exhibited greater concavity. Again, Kom (α-isomer) > Kom (β-isomer) – this and the aforementioned may have contributed to its predominance in the aquatic environment. Positive hysteresis was observed, with the CRIN soil exhibiting the highest hysteresis index. The values for the standard free energy, ΔG° = −5.11 to −14.05 kJ mol−1 K−1, depicted spontaneity and a physisorption process; driven by van der Waals force among the other soils' physicochemical properties: TOM and clay. Both α- and β-endosulfan showed a weak adsorption capacity in all the soils, with the β-isomer was more desorptive in the soil–aqueous environment. Endosulfan can easily be leached to contaminate surface and groundwater since PSOM revealed faster desorption than adsorption. However, the TOM and the clay could be used as mitigants to reduce its mobility in soils, since they have significant measures of affinities for the pesticide.
Abbreviations
BDH | British drug house |
C | Carbon |
CRIN | Cocoa Research Institute of Nigeria |
CEC | Cation exchange capacity |
CEC | Cation exchange capacity |
DDW | Double distilled water |
DCM | Dichloromethane |
GC-MS | Gas chromatography-mass spectrophotometer |
GC | Gas chromatography |
HI | Hysteresis index |
MA | Massachusetts |
MS | Mass spectrometer |
N | Nitrogen |
OCPs | Organochlorine pesticides |
OM | Organic matter |
OECD | Organisation for Economic Co-operation and Development |
PBT | Persistence Bio-accumulative, and Toxicological |
POP | Persistent organic pollutant |
PAHs | Polyaromatic hydrocarbons |
PFOM | Pseudo-first-order model |
PSOM | Pseudo-second-order model |
PH | Positive hysteresis |
RTs | Retention times |
TOM | Total organic matter |
TOC | Total organic carbon |
TN | Total nitrogen |
TETFUND | Tertiary Education Trust Funds |
USA | United States of America |
USEPA | United States Environmental Protection Agency |
Ethical statement
The study does not involve the use of animals or human participant by any of the authors.
Data availability
All data obtained in this study are included in the present manuscript.
Author contributions
Edebi N. Vaikosen: conceptualization, methodology, software; data curation; formal analysis; investigation; methodology; validation; visualization; writing – original draft. Christine M. Davidson: methodology; resources; formal analysis; visualization; validation. Bamidele I. Olu-Owolabi: supervision; formal analysis; investigation; writing – review & editing. Lorraine T. Gibson: methodology; resources; formal analysis; visualization. Foluso O. Agunbiade: formal analysis; visualization; writing – review & editing. Adesegun J. Kashimawo: formal analysis; visualization; writing – review & editing. Kayode O. Adebowale: supervision; formal analysis; visualization; investigation.
Conflicts of interest
The authors declare that there are no known competing financial interests or personal relationships that could have appeared to influence the work reported in this paper.
Acknowledgements
We sincerely acknowledge the Tertiary Education Trust Funds (TETFUND) for partial funding of this research through the Niger Delta University and also the University of Strathclyde, Glasgow, UK, for the study fellowship and full access to their research laboratories granted to Vaikosen, E. N. We also appreciate the immeasurable assistance by Ms. P. Keating of the Research Laboratory of the University of Strathclyde, Glasgow, UK. The research did not receive any specific grant from funding agency in the public, commercial or not-for-profit sector.
References
- M. Matthies, K. R. Solomon, M. Vighi, A. Gilman and J. Tarazona, The origin and evolution of assessment criteria for persistent, bioaccumulative and toxic (PBT) chemicals and persistent organic pollutants (POPs), Environ. Sci.: Processes Impacts, 2016, 18(9), 1114–1128, 10.1039/C6EM00311G
.
-
FAO/UNEP, Global assessment of soil pollution – Summary for policy makers, Food and Agriculture Organization of the United Nations, Rome, 2021, DOI:10.4060/cb4827en, accessed 27 July 2022
.
- K. S. B. Miglioranza, M. A. G. Sagrario, J. E. Aizpun de Moreno, V. J. Moreno, A. H. Escalante and M. Osterrieth, Agricultural soil as a potential source of input of organochlorine pesticides into a nearby pond, Environ. Sci. Pollut. Res., 2002, 9(4), 250–256, DOI:10.1007/BF02987499
.
-
M. Gonzalez, K. S. B. Miglioranza, J. E. Aizpun de Moreno and V. J. Moreno. Occurrence of Organochlorine Pesticides in Vegetables Grown on Untreated Soils from an Agricultural Watershed, in Water Encyclopedia, 2005, DOI:10.1002/047147844X.aw1503
.
-
R. Bateman, International Cocoa Organization (ICCO), A guide to pesticide use in cocoa, http://www.icco.org, accessed 12/02/22 Search PubMed.
- M. Kumar and L. Philip, Adsorption and desorption characteristics of hydrophobic pesticide endosulfan in four Indian soils, Chemosphere, 2006, 62, 1064–1077, DOI:10.1016/j.chemosphere.2005.05.009
.
- E. N. Vaikosen, B. I. Olu-Owolabi, L. T. Gibson, K. O. Adebowale, C. M. Davidson and U. Asogwa, Kinetic field dissipation and fate of endosulfan after application on Theobroma cacao farm in tropical Southwestern Nigeria, Environ. Monit. Assess., 2019, 191, 1–18, DOI:10.1007/s10661-019-7293-7
.
-
UNEP, Stockholm Convention on persistent organic pollutants, 2011, UNEP-POPS-Treaty-Notif-CN703-2011 on endosulfan, http://chm.pops.int/, accessed 12/10/21 Search PubMed
.
-
L. Fitzgerald and D. S. Wikoff, Persistent Organic Pollutants, in Encyclopedia of Toxicology, 3rd edn, 2014, Provisions of the Convention Search PubMed
.
- R. H. Da Cuna, F. L. Lo Nostro, V. Shimabukuro, P. M. Ondarza and K. S. B. Miglioranza, Bioaccumulation and Distribution Behavior of Endosulfan on Cichlid Fish: Differences Between Exposure to the Active Ingredient and a Commercial Formulation, Environ. Toxicol. Chem., 2020, 39(3), 604–611, DOI:10.1002/etc.4
.
- K. M. Weir, T. D. Sutherland, I. Horne, J. R. Russell and J. G. Oakeshott, A single monooxygenase, ese, is involved in the metabolism of the organochlorides endosulfan and endosulfan sulphate in an Arthrobacter sp, Appl. Environ. Microbiol., 2006, 72(5), 3524–3530, DOI:10.1128/AEM.72.5.3524-3530.2006
.
- W. F. Schmidt, S. Bilboulian, P. C. Rice, C. J. Fettinger, L. L. McConnell and J. C. Hapeman, Thermodynamic, spectroscopic and computational evidence for the irreversible conversion of beta- to alpha-endosulfan, J. Agric. Food Chem., 2001, 49, 5372–5376, DOI:10.1021/jf0102214
.
- C. J. Halsall, Investigating the occurrence of persistent organic pollutants (POPs) in the Arctic: their atmospheric behaviour and interaction with the seasonal snow pack, Environ. Pollut., 2004, 128, 163–175, DOI:10.1016/j.envpol.2003.08.026
.
-
S. M. Khan and M. S. Rahman, Pesticide Residue in Foods: Sources, Management, and Control, Springer International Publishing, 1st edn, 2017 Search PubMed
.
- E. N. Vaikosen, L. T. Gibson, C. M. Davidson, B. I. Olu-Owolabi, K. O. Adebowale, B. U. Ebeshi and P. E. N. Diagboya, GC-MS fragmentation patterns of sprayed endosulfan and its sulphate metabolite within samples of Theobroma cacao L in field kinetic study, Eur. J. Mass Spectrom., 2019b, 4, 362–371, DOI:10.1177/1469066718817690
.
- B. I. Olu-Owolabi, P. N. Diagboya and K. O. Adebowale, Evaluation of Pyrene sorption – desorption on tropical soils, J. Environ. Manage., 2014, 137, 1–9, DOI:10.1016/j.jenvman.2014.01.048
.
-
V. Chaplain, L. Mamy, L. Vieuble, C. Mougin, P. Benoit, E. Barriuso and S. Nelieu, Fate of pesticides in soils: Toward an integrated approach of influential factors, Pesticides in the modern world – Risks and benefits, Intech, 2011, pp. 535–560, DOI:10.5772/17035
.
- A. D. Atasoy, A. R. Mermut, H. Kumbur, F. Ince, H. Arslan and E. D. Avci, Sorption of alpha and beta hydrophobic endosulfan in a Vertisol from southeast region of Turkey, Chemosphere, 2009, 74, 1450–1456, DOI:10.1016/j.chemosphere.2008.12.015
.
- F. Moyo, R. Tandlich, B. S. Wilhelmi and S. Balaz, Sorption of Hydrophobic Organic Compounds on Natural Sorbents and Organoclays from Aqueous and Non-Aqueous Solutions: A Mini-Review, Int. J. Environ. Res. Public Health, 2014, 11, 5020–5048, DOI:10.3390/ijerph110505020
.
- A. D. Site, Factors affecting sorption of organic compounds in natural sorbent/water systems and sorption coefficients for selected pollutants: A review, J. Phys. Chem., 2001, 30(1), 187–439, DOI:10.1063/1.1347984
.
- J. P. Gao, J. Maguhn, P. Spitzauer and A. Kettrup, Sorption of pesticides in the sediment of the Teufelesweiher Pond (Southern Germany). I: equilibrium assessments, effect of organic carbon content and pH, Water Res., 1998, 32(5), 1662–1672 CrossRef CAS
.
-
T. Langenbach, Persistence and bioaccumulation of persistent organic pollutants (POPs), Intech, 2013, DOI:10.5772/56418
.
- I. V. Lushchaka, M. T. Matviishyna, V. V. Husaka, M. J. Storey and B. J. Storey, Pesticide Toxicity: A mechanistic approach, EXCLI J., 2018, 17, 1101–1136, DOI:10.17179/excli2018-1710
.
- S. T. Khayyun and H. A. Mseer, Comparison of the experimental results with the Langmuir and Freundlich models for copper removal on limestone adsorbent, Appl. Water Sci., 2019, 9(170), 1–8, DOI:10.1007/s13201-019-1061-2
.
- N. D. Hutson and R. T. Yang, Theoretical basis for the Dubinin-Radushkevitch (D-R) adsorption isotherm equation, Adsorption, 1997, 3(3), 189–195 CrossRef CAS
.
-
OECD (Organization for Economic Co-operation and Development), Guidelines for Testing Chemical, Guideline 106: Soil Adsorption/Desorption, Using a Batch Equilibrium Method, 2000 Search PubMed
.
-
USEPA, Fate, Transport and Transformation Test Guidelines, OPPTS 835.1230 Adsorption/Desorption (Batch Equilibrium), 2008 Search PubMed
.
- L. Cox, M. C. Hermosín and J. Cornejo, Adsorption of methomyl by soils of southern Spain and soil components, Chemosphere, 1993, 837–849, DOI:10.1016/0045-6535(93)90015-W
.
- K. R. Krishna and L. Philip, Adsorption and desorption characteristics of lindane, carbofuran and methyl parathion on various Indian soils, J. Hazard. Mater., 2008, 160, 559–567, DOI:10.1016/j.jhazmat.2008.03.107
.
- B. Wang, J. Huang, Y. Lu and S. Arai, The pollution and ecological risk of endosulfan in soil of Huai'an city, China, Environ. Monit. Assess., 2012, 184(12), 7093–7101, DOI:10.1007/s10661-011-2482-z
.
- I. M. Meftaul, K. Venkateswarlu, R. Dharmarajan, P. Annamalai and M. Megharaj, Movement and Fate of 2,4-D in Urban Soils: A Potential Environmental Health Concern, ACS Omega, 2020, 5, 13287–13295, DOI:10.1021/acsomega.0c01330
.
- T. Paszko, P. Muszynski, M. Materska, M. Bojanowska, M. Kostecka and I. Jackowska, Adsorption and degradation of phenoxyalkanoic acid herbicides in soils: A review, Environ. Toxicol. Chem., 2016, 35(2), 271–286, DOI:10.1002/etc.3212
.
- H. Yu, Y. Liu, X. Shu, H. Fang, X. Sun, Y. Pan and L. Ma, Equilibrium, kinetic and thermodynamic studies on the adsorption of atrazine in soils of the water fluctuation zone in the Three-Gorges Reservoir, Environ. Sci. Eur., 2020, 32, 27, DOI:10.1186/s12302-020-00303-y
.
- S. Qian, H. Zhu, B. Xiong, G. Zheng, J. Zhang and W. Xu, Adsorption and desorption characteristics of endosulfan in two typical agricultural soils in Southwest China, Environ. Sci. Pollut. Res., 2017, 24, 11493–11503, DOI:10.1007/s11356-017-8800-4
.
- C. E. Merem, A. Y. Twumasi, J. Wesley, D. Olagbegi, M. Crisler, C. Romorno, M. Alsarari, P. Isokpehi, A. Hines, S. G. Ochai, E. Nwagboso, S. Fageir and S. Leggett, Exploring cocoa farm land use in the West African Region, Int. J. Agric. For., 2020, 10(1), 19–39, DOI:10.5923/j.ijaf.20201001.03
.
- K. M. Weir, T. D. Sutherland, I. Horne, R. J. Russell and J. G. Oakeshott, A single monooxygenase, ese, is involved in the metabolism of the organochlorides endosulfan and endosulfan sulphate in an Arthrobacter sp, Appl. Environ. Microbiol., 2006, 72(5), 3524–3530, DOI:10.1128/AEM.72.5.3524-3530.2006
.
- D. A. Bohm, C. S. Stachel and P. Gowik, Confirmatory method for the determination of streptomycin in apples by LC-MS/MS, Anal. Chim. Acta, 2010, 672(1–2), 103–106, DOI:10.1016/j.aca.2010.03.056
.
- A. Shrivastava and V. B. Gupta, Methods for the determination of limit of detection and limit of quantitation of the analytical methods, Chron. Young Sci., 2011, 2, 21–25 CrossRef
.
- S. E. Stein, Chemical Substructure Identification by Mass Spectral Library Searching, J. Am. Soc. Mass Spectrom., 1995, 6(8), 644–655, DOI:10.1016/1044-0305(95)00291-K
.
- S. Lagergren, Zur theorie der sogenannten adsorption gelöster stoffe. Kungliga svenska vetenskapsakademiens, Handlingar, 1898, 24, 1–39 Search PubMed
.
- I. Langmuir, The constitution and fundamental properties of solids and liquids. Parts 1. Solids, J. Am. Chem. Soc., 1916, 38, 2221–2295, DOI:10.1021/ja02268a002
.
- H. M. F. Freundlich, Über die adsorption in lösungen, Z. Phys. Chem., 1906, 57A, 385–470 Search PubMed
.
-
Food and Agriculture Organization (FAO), Parameters of pesticides that influence processes in the soil, https://www.fao.org/3/x2570e/X2570E06.htm#ch8, accessed 28 July 2022 Search PubMed.
- D. I. Gustafson, Groundwater ubiquity score: A simple method for assessing pesticide leachability, Environ. Toxicol. Chem., 1989, 8(4), 339–357, DOI:10.1002/etc.5620080411
.
- J. Zhang, C. Wu, A. Jia and B. Hu, Kinetics, equilibrium and thermodynamics of the sorption of p-nitrophenol on two variable charge soils of Southern China, Appl. Surf. Sci., 2014, 298, 95–101, DOI:10.1016/j.apsusc.2014.01.130
.
-
National Institute of Standards and Technology, (NIST) search library software, 2014 Search PubMed
.
-
ICH, International Conference on Harmonization of Technical Requirements for Registration of Pharmaceuticals for Human use, ICH Harmonized Tripartite Guideline, ICH Q2A. Text on validation of analytical procedures, 2005, accessed 22 July 2022 Search PubMed
.
- M. A. Locke, Sorption-desorption kinetics of alachlor in surface soil from two soyabean tillage systems, J. Environ. Qual., 1992, 21, 558–566, DOI:10.2134/jeq1992.00472425002100040006x
.
- B. von Oepen, W. Kordel and W. Klein, Sorption of nonpolar compounds to soils: processes, measurements and experience with the applicability of the modified OECD- guideline 106, Chemosphere, 1991, 22, 285–305, DOI:10.1016/0045-6535(91)90318-8
.
- P. Parkpian, P. Anurakpongsatorn, P. Pakkong and W. H. Patrick, Adsorption, desorption and degradation of endosulfan in tropical soils of Thailand, J. Environ. Sci. Health, Part B, 1998, 33, 211–233, DOI:10.1080/03601239809373140
.
- J. Weber, J. C. Halsall, D. Muir, C. Teixeira, J. Small, K. Solomon, M. Hermanson, H. Hung and T. Bidleman, Endosulfan, a global pesticide: A review of its fate in the environment and occurrence in the Arctic, Sci. Total Environ., 2010, 408, 2966–2984, DOI:10.1016/j.scitotenv.2009.10.077
.
- C. Chan, D. Williams, M. Neilson, B. Harrison and M. Archer, Spatial and temporal trends in the concentrations of selected organochlorine pesticides (OCs) and polynuclear aromatic hydrocarbons (PAHs) in Great Lakes Basin precipitation, 1986 to 1999, Atmos. Environ., 2003, 40, 1563–1578, DOI:10.1016/S0380-1330(03)70450-X
.
- M. A. Dalvie, E. Cairncross, A. Solomon and L. London, Contamination of rural surface and ground water by endosulfan in farming areas of the Western Cape, South Africa, Environ. Health, 2003, 2(1) DOI:10.1186/1476-069X-2-1
.
- H. C. Giles, H. T. MacEwan, N. S. Nakhwa and D. Smith, Studies in Adsorption. Part XI. A system of classification of solution adsorption isotherms and its use in diagnosis of adsorption mechanisms and in measurement of specific surface
areas of solids, J. Chem. Soc., 1960, 3, 3973–3993, 10.1039/jr9600003973
.
- S. Mohan and J. Karthikeyan, Removal of lignin and tannin color from aqueous solution by adsorption onto activated charcoal, Environ. Pollut., 1997, 97, 183–187, DOI:10.1016/S0269-7491(97)00025-0
.
- R. Singh and Y. Saxena, Adsorption – desorption behaviour of selected pesticides in some Western Australian soils, Aust. J. Soil Res., 1986, 28, 227–243, DOI:10.1071/SR9900227
.
- V. Chantawong, N. W. Harvey and V. N. Bashkin, Comparison of heavy metal adsorptions by Thai kaolin and ball clay, Water, Air, Soil Pollut., 2003, 148, 111–125, DOI:10.1023/A:1025401927023
.
- F. Gao, J. Jia and X. Wang, Occurrence and ordination of dichlorodiphenyltrichloroethane and hexachlorocyclohexane in agricultural soils from Guangzhou, China, Arch. Environ. Contam. Toxicol., 2008, 54(2), 155–166, DOI:10.1007/s00244-007-9023-3
.
- Y. Si, J. Zhang, S. Wang, L. Zhang and D. Zhou, Influence of organic amendment on the adsorption and leaching of ethametsulfuron-methyl in acidic soils in China, Geoderma, 2006, 130, 66–76, DOI:10.1016/j.geoderma.2005.01.009
.
- H. Barchanska, M. Czaplicka and J. Kyziol-Komosinska, Interaction of selected pesticides with mineral and organic soil components, Arch. Environ. Prot., 2020, 46(3), 80–91, DOI:10.24425/aep.2020.134538
.
- S. Chianese, A. Fenti, P. Iovino, D. Musmarra and S. Salvestrini, Sorption of Organic Pollutants by Humic Acids: A Review, Molecules, 2020, 25(918), 1–17, DOI:10.3390/molecules25040918
.
- I. Cwielag-Piasecka, M. Debica and A. Medynska-Juraszek, Effectiveness of carbaryl, carbofuran and metolachlor retention in soils under the influence of different colloid, Minerals, 2021, 11, 924, DOI:10.3390/min11090924
.
- A. Torrents and S. Jayasundera, The sorption of non-ionic pesticides onto clays and the influence of natural organic carbon, Chemosphere, 1997, 35(7), 1549–1565, DOI:10.1016/S0045-6535(97)00206-3
.
- W. Huang, P. Peng, Z. Yu and J. Fu, Effects of organic matter heterogeneity on sorption and desorption of organic contaminants by soils and sediments, Appl. Geochem., 2003, 18, 955–972, DOI:10.1016/S0883-2927(02)00205-6
.
- C. A. Seybold and W. Mersie, Adsorption and desorption of atrazine, deethylatrazine, deisopropylatrazine, hydroxyatrazine, and metolachlor in two soils from Virginia, J. Environ. Qual., 1996, 25(6), 1179–1185, DOI:10.1021/jf950370k
.
- R. I. Swann, D. A. Laskowski, P. J. Mc Call and K. V. Kuy, A rapid method for estimation of the environmental parameters octanol/water parting coefficient, soil sorption constant water to air ratio and water solubility, Res. Rev., 1983, 85, 17–28 CAS
.
- R. P. Schwarzenbach and J. Westall, Transport of nonpolar organic compounds from surface water to groundwater. Laboratory sorption studies, Environ. Sci. Technol., 1981, 15, 1360–1367, DOI:10.1021/es00093a009
.
- P. Banerjee, D. M. Piwoni and K. Ebeid, Sorption of organic compounds to a low carbon subsurface core, Chemosphere, 1985, 14, 1057–1067, DOI:10.1016/0045-6535(85)90026-8
.
-
C. Tomlin, The pesticide manual: Incorporating the agrochemicals handbook, British Crop Protection Council Publications and the Royal Society of Chemistry, 10th edn, 1994, pp. 407–408 Search PubMed
.
-
W. J. Weber Jr, Physicochemical processes for water quality control, Wiley-Interscience, New York. 1972 Search PubMed
.
-
W. A. Adamson, Physical Chemistry of Surfaces, Wiley, New York, 3rd edn, 1976 Search PubMed
.
- T. B. Bowman and W. W. Sans, Partitioning behavior of insecticide in soil-water system: desorption hysteresis effect, J. Environ. Qual., 1985, 14, 270–273, DOI:10.2134/jeq1985.00472425001400020023x
.
-
W. C. Koskinen and S. S. Harper, The retention processes: mechanisms, in Pesticides in the Soil Environment: Processes, Impacts and Modelling, ed. H. H. Cheng, Soil Sci. Soc. Am. Book Series, No. 2, Madison, Wisconsin, 1990 Search PubMed
.
- W. R. Roy and I. G. Krapac, Adsorption and desorption of atrazine and deethylatrazine by low organic carbon geological materials, J. Environ. Qual., 1994, 23, 549–556, DOI:10.2134/jeq1994.00472425002300030021x
.
- T. G. K. Stolze and K. U. Totsche, Pathways of biogenically excreted organic matter into soil aggregates, Soil Biol. Biochem., 2022, 164, 108483, DOI:10.1016/j.soilbio.2021.108483
.
- W. Yang, J. Zhang, C. Zhang, L. Zhu and W. M. Chen, Sorption and resistant desorption of atrazine in typical Chinese soils, J. Environ. Qual., 2009, 38, 171–179, DOI:10.2134/jeq2007.0674
.
- D. L. D. Lima, J. R. Schneider, W. H. Scherer, C. A. Duarte, H. B. E. Santos and I. V. Esteves, Sorption-desorption of atrazine on soils subjected to different organic long-term amendment, J. Agric. Food Chem., 2010, 58, 3101–3106, DOI:10.1021/jf903937d
.
- A. O'Connor, J. P. Wierenga, H. H. Cheng and G. K. Doxtader, Movement of 2,4,5T through large soil columns, Soil Sci., 1980, 130, 157–162 CrossRef
.
- W. Huang, H. Yu and W. J. Weber, Hysteresis in the sorption and desorption of hydrophobic organic contaminants by soils and sediments: 1. A comparative analysis of experimental protocols, J. Contam. Hydrol., 1998, 31(1–2), 129–148, DOI:10.1016/S0169-7722(97)00059-4
.
- A. C. Langaro, M. F. Souza, G. A. M. Pereira, J. P. A. Barros, A. A. da Silva, D. V. Silva, A. B. R. Passos and V. Mendonça, Influence of Glyphosate Formulations on the Behavior of Sulfentrazone in Soil in Mixed Applications, Toxics, 2020, 8(4), 123–137, DOI:10.3390/toxics8040123
.
- Y. Bao, Q. Zhou, Y. Wan, Q. Yu and X. Xie, Effects of soil/solution ratios and cation types on adsorption and desorption of tetracycline in soils, Soil Sci. Soc. Am. J., 2010, 74(5), 1553–1561, DOI:10.2136/sssaj2009.0402
.
- A. O. Adeola, J. de Lange and P. B. C. Forbes, Adsorption of antiretroviral drugs, efavirenz and nevirapine from aqueous solution by graphene wool: Kinetic, equilibrium, thermodynamic and computational studies, App. Sur. Sci. Adv., 2021, 6(1), 100157, DOI:10.1016/j.apsadv.2021.100157
.
- M. Sander, Y. Lu and J. J. Pignatello, A thermodynamically based method to quantify true sorption hysteresis, J. Environ. Qual., 2005, 34, 1063–1072, DOI:10.2134/jeq2004.0301
.
- B. Chefetz, I. Y. Bilkis and T. Polubesova, Sorption – desorption of atrazine and phenylurea herbicides in Kishon river sediments, Water Res., 2004, 38, 4383–4394, DOI:10.1016/j.watres.2004.08.023
.
- Y. Drori, Z. Aizenshtat and B. Chefetz, Sorption-desorption behaviour of atrazine in soils irrigated with reclaimed wastewater, Soil Sci. Soc. Am. J., 2005, 69(6), 1703–1710, DOI:10.2136/sssaj2004.0345
.
|
This journal is © The Royal Society of Chemistry 2023 |
Click here to see how this site uses Cookies. View our privacy policy here.