DOI:
10.1039/D0BM01155J
(Paper)
Biomater. Sci., 2021,
9, 108-115
In vivo vocal fold augmentation using an injectable polyethylene glycol hydrogel based on click chemistry†
Received
13th July 2020
, Accepted 12th October 2020
First published on 15th October 2020
Abstract
It is important to focus on urgent needs in clinics and develop optimal materials. For successful augmentation of vocal folds, the ideal filler should be injectable through a syringe, and should stably maintain its volume for a long time without toxicity. To achieve these criteria, a click chemistry-based PEG (polyethylene glycol) hydrogel was developed and applied for vocal fold augmentation in vivo. The PEG hydrogel enables fast gelation in vivo after injection and provides long-term stability. Azide- and dibenzocyclooctyne (DBCO)-modified 4-arm PEG were cross-linked by chemical conjugation via click chemistry and yielded gelation within several minutes. After subcutaneous injection into mice and rats, the PEG hydrogel showed higher stability after 1 month compared to the traditionally used calcium hydroxyapatite–carboxymethyl cellulose (CaHA-CMC) filler. In rabbit models with vocal fold paralysis, the PEG hydrogel stably fixed the paralyzed vocal fold in 4 months and minimized the glottic gap. It was an improved therapeutic result compared to CaHA-CMC, demonstrating the potential of a click chemistry-based PEG hydrogel for vocal fold therapy.
Introduction
The main functions of the larynx are voice production and airway protection, which can be fully achieved by the vocal fold. Several pathologic conditions, such as vocal cord paralysis, paresis, atrophy, and presbylarynx, cause glottal insufficiency. Voice therapy has been the first step for treatment of glottal insufficiency, but in many cases, additional treatment is needed.1–4 Injection laryngoplasty is one of the most popular procedures because this procedure can be easily performed in the office and has benefits in terms of cost and morbidity compared to surgical methods.5,6 Different types of materials have been introduced for injection laryngoplasty. Hyaluronic acid is commonly used for short period augmentation, and calcium hydroxyapatite (CaHA) for long period augmentation.7,8 CaHA is a biomaterial that has been approved by the Food and Drug Administration (FDA) and has been used for more than 20 years for this purpose. Many studies have reported that the injection of CaHA could be effective in treating glottal insufficiency for more than 12 months.9,10 However, complications such as migration, inflammation, and early volume decrease have been reported.11
For successful vocal fold augmentation, the ideal filler needs to have several properties. First, it should be injectable through a syringe with a thin needle. Second, it should stably maintain its volume during the intended period of curing. Third, the material should be non-toxic to humans. Finally, the material needs to contain at most only a small amount of foreign materials. A hydrogel is a mass of polymers that can maintain its shape and that contains a considerable amount of water.12 Various kinds of hydrogels have been developed and applied to biomedical fields for tissue engineering and drug delivery.13,14 Owing to their thermosensitive gelation and biocompatibility, Pluronic F127 hydrogels were used as fillers in vocal folds by Kwon et al. in 2014, but a large amount of polymers (25 wt%) was required for gelation in their work.15 As a gelation mechanism of hydrogels, chemical cross-linking between polymers has been studied for a long time.16,17 In particular, if the cross-linking reaction can occur in vivo, it is useful for in situ gelation and enables the hydrogel to be injectable, with the hydrogel changing from a liquid into a gel after injection into the body.18 A hydrogel based on cross-linking can form a stable network structure with a relatively less amount of polymers. Recently, click chemistry has been regarded as an attractive technique for cross-linking polymers to obtain hydrogels.19 Click chemistry is a term for chemical reactions that are simple to use, fast and orthogonal and provide easily purifiable products in high yields.20 In particular, copper-free click chemistry, such as strain-promoted cycloaddition and the inverse-electron-demand Diels–Alder reaction, can conjugate two molecules without a catalyst, which has enabled chemical conjugation in vivo and demonstrated its utility in many studies.21,22
Herein, we used a click chemistry-based hydrogel for vocal fold augmentation. For the polymer backbone, we used polyethylene glycol (PEG), a representative non-toxic polymer approved for human usage by the FDA. Two modified forms of 4-arm PEG polymers were made, with the end groups of the 4-arm PEG polymers functionalized with azide (N3) and dibenzocyclooctyne (DBCO) for subsequent click chemistry. The resulting 4-arm PEG-N3 and 4-arm PEG-DBCO reacted with each other to form stable hydrogels via click chemistry by simple mixing together without a catalyst (Fig. 1). The gelation process and mechanical properties of the click-based polymer hydrogel were observed in vitro. Then, the click-based hydrogel was injected into a rat model to observe its biocompatibility. Finally, the hydrogel was injected into a rabbit model with the right inferior thyroid nerve cut and the therapeutic ability for vocal fold augmentation was evaluated for 4 months. To the best of our knowledge, this is the first time that cross-linked polymer hydrogels have been applied for vocal fold augmentation in vivo.
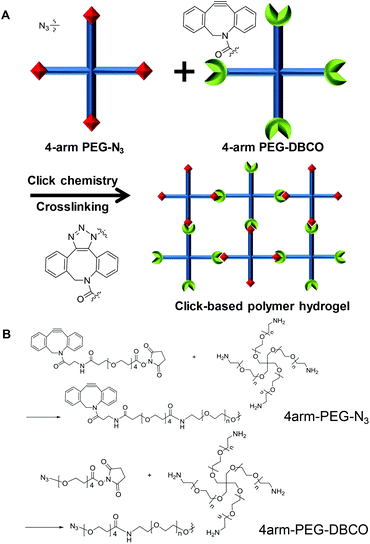 |
| Fig. 1 Schematic illustration of the click chemistry-based PEG hydrogel. | |
Experimental
Materials
4-Arm PEG-NH2 (MW: 10 kDa) was purchased from Laysan Bio (Arab, AL, USA). DBCO-PEG5-NHS and azide-PEG4-NHS were purchased from Click Chemistry Tools (Scottsdale, AZ, USA). N,N-Diisopropylethylamine (DIPEA) and fluorescamine were purchased from Sigma-Aldrich (St Louis, MO, USA). Ethyl alcohol, 99.9%, was purchased from Duksan (Seongnam, Gyeonggi-do, Korea). Radiesse (Merz North America, Inc., USA) was used as a control filler containing calcium hydroxyapatite (CaHA) microspheres (35 ± 10 μm) and carboxymethylcellulose (CMC) gel as a carrier.
Synthesis of 4-arm PEG-N3 and 4-arm PEG-DBCO
The amine groups of 4-arm PEG-NH2 were conjugated with the NHS ester groups of DBCO-PEG5-NHS ester by amine-NHS coupling. 4-Arm PEG-NH2 (20 mg) was dissolved in 1 ml of distilled water and DBCO-PEG5-NHS ester (8.33 mg) was dissolved in 1 ml of ethyl alcohol. The two solutions were mixed with 6.96 μl of DIPEA. The resulting solution was stirred at room temperature for 2 h, forming 4-arm PEG-DBCO. Unreacted DBCO-PEG5-NHS ester was eliminated by dialysis in distilled water, 50% ethanol, and 100% ethanol for one day. The final solution was fully lyophilized to yield a powder. 4-Arm PEG-N3 was synthesized similarly using azide-PEG4-NHS. 1H NMR was performed on a Delta (600 MHz) spectrometer in CDCl3. Chemical shifts were reported in parts per million (δ) and calibrated using the internal tetramethylsilane (TMS) standard or residual undeuterated solvent for 1H NMR spectra (CDCl3 7.26 ppm). For fluorescamine assay, after incubation with fluorescamine for 15 minutes, the fluorescence of the solutions was measured using a synergy H1 Hybrid Multi-Mode Reader (Biotek Instruments, Inc., Winooski, VT, USA) at 365/470 nm.
Cell viability test
The 3-(4,5-dimethylthiazol-2-yl)-2,5-diphenyltetrazolium bromide (MTT) assay was implemented for measuring NIH3T3 cell viability. 8 × 103 cells per well were placed in a 96-well plate and incubated for 24 h. After a washing step with DPBS (pH 7.4), either 4-arm PEG-N3 or 4-arm PEG-DBCO was dissolved in culture media and was used to treat the cells for 24 h. After the washing step, the MTT solution was placed in all the wells of the plate and incubated for 2 h. After removing the solution, 100 μl of DMSO was poured into the wells. The absorbance of the solution was measured at 570 nm using a synergy H1 Hybrid Multi-Mode Reader. For viability testing of cells in the hydrogel, 4-arm PEG-N3 solution containing NIH 3T3 cells (5 × 105) was mixed with 4-arm PEG-DBCO solution in a confocal dish. After 100 μl of 4 wt% hydrogel was formed, growth medium was added until the hydrogel was submerged. The hydrogel was incubated for 24 hours, washed with DPBS, and stained with a LIVE/DEAD Viability/Cytotoxicity Kit (Invitrogen, USA). Confocal images were obtained at AF488 and rhodamine wavelengths using an LSM800 confocal laser scanning microscope (Carl Zeiss, Germany).
Gelation and rheological analysis of the hydrogel
The 4-arm PEG-DBCO and 4-arm PEG-N3 powders were dissolved in distilled water to a specific weight percent. After one solution was added to the other solution, the mixed solution reacted to form a PEG hydrogel within several minutes. A strain-controlled rheometer (ARES G2, TA Instruments) with 25 mm diameter parallel plates was used to analyze the rheological properties of the PEG hydrogel. G′ and G′′ were measured with oscillatory frequency sweeps from 0.1 to 100 Hz, at a shear strain of 1%, a temperature of 25 °C, and an axial force of 1 N. The volume of the sample used for the measurement was 250 μl. For the adhesion test, 4 wt% hydrogel (200 μl) was formed on a glass slide. Various substrates such as polypropylene, rubber, glass, steel, mouse liver, and mercury batteries were placed onto the hydrogel for a few minutes to adhere. Then, the slide was inverted, and adhesion was observed for more than 5 minutes.
Stability test of hydrogels in mice and rats
This study was approved by the Animal Ethics Committee of Catholic University of Korea (Permit No. CMCDJ-AP-2018-010). The animals were cared for in accordance with our established institutional guidelines. Twenty-eight male C57BL/6 mice weighing 22–24 g (Damul Science, Daejeon, Korea) were used for the stability test of the hydrogels. The mice were kept at room temperature (23–25 °C) and 40–60% relative humidity on a 12/12 h light/dark cycle, and fed with a standard pellet diet and water ad libitum. A 200 μL volume of the 4 wt% PEG hydrogel or CaHA-CMC was injected subcutaneously on the dorsal side of the mice with 26G needles (n = 14). One month after injection, the mice were euthanized with cervical dislocation. The hair on the injected site was epilated to observe macroscopically. Then the injected sites were removed for histological examination. The volume of the injected materials was measured using water displacement.
A total of four Sprague-Dawley rats (Damul Science, Daejeon, Korea) were used to evaluate the stability of the hydrogel in the larynx. All rats were anesthetized via intraperitoneal injection of ketamine hydrochloride (100 mg kg−1) and xylazine hydrochloride (10 mg kg−1). Each animal was placed in a semi-vertical position on a custom-made platform. The larynx was visualized with a pediatric endoscope. A total volume of 0.05 ml PEG hydrogels with 4 wt% polymer concentration was injected in the lateral side of the arytenoid cartilage with a 26G needle. One month after injection, the larynxes were harvested for histological examination.
Vocal fold augmentation in rabbit models
Sixteen New Zealand white rabbits (Damul Science, Daejeon, Korea) weighing 3.0 kg were used for the vocal fold augmentation study. All animals were divided into two groups for PEG hydrogel and CaHA-CMC treatment. After premedication with xylazine (Rompun 10 mg kg−1; Bayer, Berlin, Germany), all rabbits were anesthetized via intramuscular administration of 50 mg kg−1 of ketamine. Each rabbit was then placed in dorsal recumbency. The neck area was shaved. A vertical incision was made at the midline of the neck. The strap muscles were dissected until the thyroid gland and trachea were encountered. The isthmus of the thyroid gland was divided. The right inferior thyroid nerve was identified around the inferior thyroid artery at the medial side of the thyroid gland. The right inferior thyroid nerve was cut, and endoscopic examination was performed in all rabbits after anesthesia. A 150 μL volume of the PEG hydrogel or CaHA-CMC was injected into the right vocal fold with a 23G long needle under direct vision employing a 0° endoscope (n = 8). The correct injection site was confirmed by observing medializing and bulging of the vocal fold. At 0, 1, 2, and 4 months after the injection, photographs of the rabbit vocal folds were taken with a rigid endoscope. We outlined the injected material and plotted the distance from the vocal fold free edge to the contour of the injected material at the end point of the vocal process.
Four months after injection, four rabbit larynges from each group were harvested for functional analysis after euthanasia. For precise observation of the vocal fold, the supraglottic structure was removed. To measure the degree of medialization of the injected vocal fold during phonation, artificial vocal fold vibration was induced by supplying air from the bottom of the resected trachea toward the vocal fold. Vibration images were recorded with a high-speed video camera (X9PRO, Megaspeed, Canada, 5000 fps). The acquired image data were analyzed using TEMA Automotive (Image Systems, Sweden). An imaginary midline between the anterior commissure and the mid-point of the posterior commissure was drawn. The distances of the midline to the left vocal fold (normal) and right vocal fold (injected side) were measured. ‘Max’ and ‘Min’ were the maximum and minimum distances from the midline to each vocal fold during the vibration cycle.
The larynges of the four rabbits were harvested from each group for histological examination 4 months after injection. The specimens were embedded in paraffin blocks and sectioned with a thickness of 4 μm along the coronal axis using a microtome. The specimens were stained with hematoxylin and eosin (H&E), using standard pathology department protocols. The stained samples were observed under a light microscope (Eclipse TE300; Nikon, Tokyo, Japan).
Results and discussion
Development and characterization of a click chemistry-based PEG hydrogel
As a filler for vocal fold augmentation, we fabricated a PEG hydrogel, which was cross-linked by click chemistry. The end groups of 4-arm PEG were modified by either DBCO or N3 (Fig. 1B). Conjugation of azide and DBCO groups to 4-arm PEG-amine was characterized by 1H NMR, as shown in Fig. S1.† We also confirmed the loss of primary amine groups after conjugation in both cases by fluorescamine assay, which means successful conjugation of azide and DBCO groups. When the resulting 4-arm PEG-N3 and 4-arm PEG-DBCO solutions were simply mixed together, we observed hydrogel formation within 5 min (Fig. 2A). To optimize the properties of the hydrogels, we prepared PEG hydrogels with 2 and 4 wt% polymer concentrations. Both polymers (4-arm PEG-N3 and 4-arm PEG-DBCO) before gelation were soluble in water, and they showed negligible toxicity until 20 mg ml−1 in a cell viability test with NIH3T3 fibroblast cells (Fig. 2B). In the rheological analysis, the values of the storage modulus (G′) and loss modulus (G′′) for the 2 and 4 wt% hydrogels were constant, and the G′ values were higher than the G′′ values in all ranges with increasing frequency and time, indicating that both hydrogels were successfully formed (Fig. 2C and D). After mixing, gelation proceeded very fast, within several minutes, with G′ higher than G′′ from the onset. The 4 wt% hydrogel had a higher G′ value than the 2 wt% hydrogel, showing that the 4 wt% hydrogel was stiffer. Therefore, we used the 4 wt% hydrogel for further animal studies, which needed high stability in vivo. We tested hydrogel formulations with different ratios of 4arm-PEG-N3 and 4arm-PEG-DBCO while the total weight concentration was maintained at 4 wt%, as shown in Fig. S2.† The hydrogel was successfully formed at ratios from 1
:
2 to 2
:
1 (4arm-PEG-N3 and 4arm-PEG-DBCO), and we selected the median 1
:
1 ratio for further studies. We also tested the adhesive properties of the PEG hydrogel. As shown in Fig. S3,† the hydrogel adhered to various substrates such as polypropylene, rubber, glass, steel, mouse liver, and mercury batteries. An amount of 200 μl of hydrogel could hold 10 mercury batteries (about 19 g), illustrating its adhesivity and stability, which would be useful for vocal fold augmentation. In addition, we tested the viability of cells in the hydrogel by live/dead staining with calcein AM and ethidium homodimer-1. After growing in the hydrogel for one day, most cells showed the green fluorescence of calcein AM, while the red color of ethidium homodimer-1 was sparse, indicating the presence of live cells, as shown in Fig. S4.†
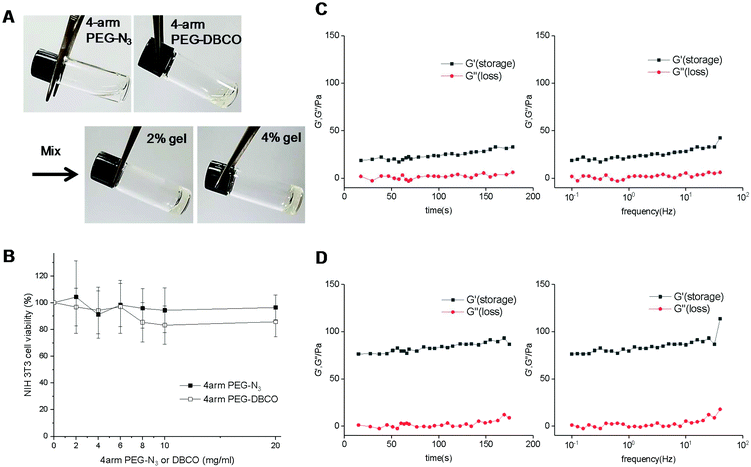 |
| Fig. 2 Characterization of the click chemistry-based PEG hydrogels. (A) Gelation images of 4-arm PEG-N3, 4-arm PEG-DBCO, and click chemistry-based PEG hydrogels (2 or 4 wt%). (B) Cell viability of NIH3T3 cells after treatment with 4-arm PEG-N3 and 4-arm PEG-DBCO. Rheology data (value of G′ and G′′ of the hydrogel according to time and frequency) of the click chemistry-based PEG hydrogels at (C) 2 wt% and (D) 4 wt%. | |
In vivo stability test of the click chemistry-based PEG hydrogel in mice and rats
To test the stability of the PEG hydrogel in vivo, we injected equal volumes of the PEG hydrogel and CaHA-CMC (calcium hydroxyapatite–carboxymethyl cellulose) as a control under the skin of mice and observed them after 1 month. After harvest, the injected PEG hydrogel formed a round shape and did not spread, but CaHA-CMC became flat and easily divided into several lumps with low stability (Fig. 3A). One month after injection under the skin, the measured volume of the PEG hydrogel was significantly larger than that of CaHA-CMC (PEG = 215 ± 13.2 μl vs. CaHA-CMC = 165 ± 11.9 μl; P < 0.05) and the PEG hydrogel showed less volume change than CaHA-CMC, demonstrating the superior in vivo stability of the PEG hydrogel (Fig. 3B). We think that the dense cross-linking resulting from click chemistry endowed the PEG hydrogel with high stability and prevented its migration on the tissue. The volume of the PEG hydrogel increased from the injected volume, suggesting that it absorbs water from the surroundings. H&E staining of the sliced mouse tissue revealed that 1 month after subcutaneous injection there was no foreign body response around the PEG hydrogel (Fig. 3C). Separately, after injection of the PEG hydrogel into the larynx of a rat, we also observed no abnormality of the larynx tissue and no significant foreign body response after H&E staining (Fig. 3D and E).
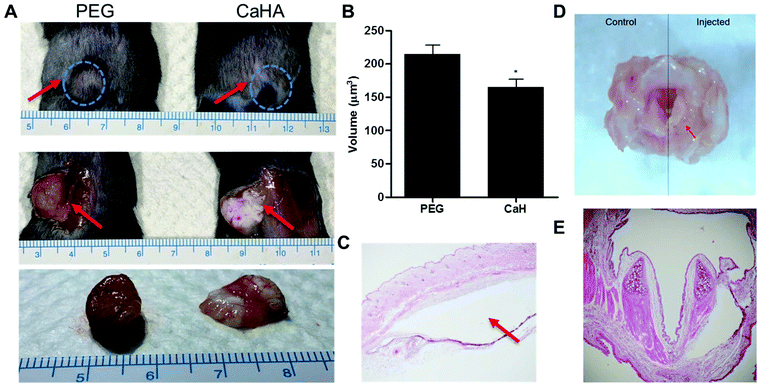 |
| Fig. 3
In vivo stability test of the PEG hydrogel after injection into mice and rats. (A) Images of the PEG hydrogel and CaHA under mice skin after 1 month. (B) Volume comparison between the PEG hydrogel and CaHA-CMC. (C) H&E stained images of the PEG hydrogel under mice skin. The red arrow marks the injection sites. (D) Image of the PEG hydrogel in the rat larynx after 1 month. The red arrow marks the injection sites. (E) H&E stained images of the PEG hydrogel in the rat larynx. | |
Vocal fold augmentation by the click chemistry-based PEG hydrogel in rabbit models
Encouraged by the data from the mice and rat experiments, we tested the therapeutic efficacy of our PEG hydrogel in a rabbit model with right vocal fold paralysis. We injected the PEG hydrogel and CaHA-CMC into the right vocal fold, and conducted endoscopic examinations 0, 1, and 4 months after injection. The distance from the right vocal fold free edge to the contour line of the injected material was shorter in the PEG hydrogel group than in the CaHA-CMC group 1 and 4 months after injection (Fig. 4). These results indicated that the injected PEG hydrogel showed less volume loss compared to CaHA-CMC. In the CaHA-CMC group, a remarkable volume reduction was observed 1 month after injection.
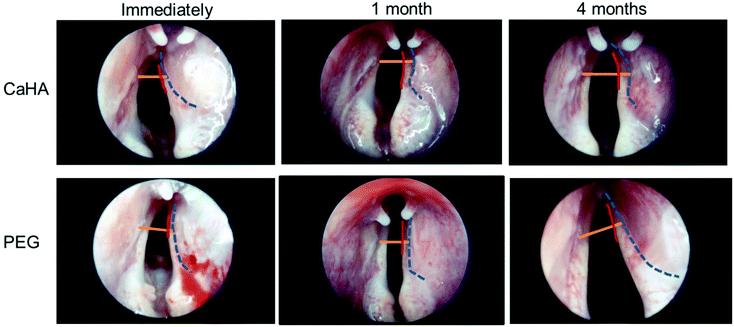 |
| Fig. 4 Endoscopic images conducted immediately and 1 and 4 months after injection with CaHA and PEG. The red, blue, and orange lines indicate the vocal fold free edge, the contour of the injected material, and the connection line of both ends of the vocal process, respectively. | |
The normal vocal fold (the left side vocal fold in this experiment) could perform adduction and abduction movements, so the wide glottic gap was not a problem. However, because the paralyzed vocal fold (right side) had no movement, the normally wide glottic gap was not helpful for vocalization, and it needed to be narrowed. Clinical treatment for vocal fold augmentation is typically required to move the paralyzed vocal fold close to the midline (Fig. 5A).
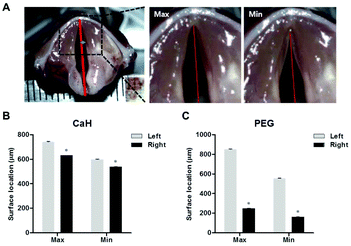 |
| Fig. 5 Functional analysis of the treated vocal fold by measuring the maximum and minimum amplitudes with a high speed video camera. (A) The method to calculate the maximum (Max) and minimum (Min) amplitudes. The amplitude of the vocal folds injected with (B) CaHA and (C) the PEG hydrogel (P < 0.05). | |
The more the amplitude is reduced, the more effective the treatment is. In the CaHA-CMC group, the maximum (Max) and minimum (Min) amplitudes of the right (Rt) side vocal folds were slightly decreased compared to the untreated left (Lt) side vocal folds (Fig. 5B, Rt Max = 630.5 ± 0.705 vs. Lt Max 742.3 ± 1.459; P < 0.05, and Rt Min = 537.6 ± 0.884 vs. Lt Min 598.4 ± 2.840; P < 0.05). However, when the PEG hydrogel was injected, both the Max and Min amplitudes of the right side vocal folds decreased to <300 μm (in the PEG group, Rt Max = 276.5 ± 3.249 vs. Lt Max 663.6 ± 1.632; P < 0.05, and Rt Min = 141.2 ± 2.621 vs. Lt Min 387.2 ± 4.736; P < 0.05). These data showed that the PEG hydrogel stably fixed the paralyzed vocal fold after 4 months, resulting in effective treatment.
Histopathological examination showed that the injected PEG hydrogel remained at the injection site without migration in the thyroarytenoid muscle (Fig. 6A). The cells were demarcated well among muscle bundles and were loculated. Infiltration of the hydrogel to the surrounding tissue was limited and there was no vasculitis. However, the injected CaHA-CMC partially leaked out to the surrounding fat and muscle tissue (Fig. 6B). CaHA-CMC was deposited into adjacent muscle tissues (blue arrow), and vasculitis was observed from the surrounding fat tissue (black arrows). The injected material formed granules that consisted of foreign body giant cells. Both lymphoplasma cells and inflammatory cells, such as neutrophils, were observed.
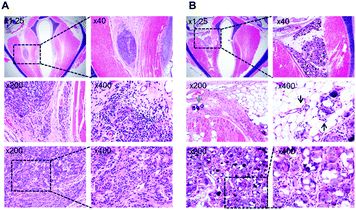 |
| Fig. 6 H&E staining of the vocal fold augmented after 16 weeks: (A) The PEG hydrogel and (B) CaHA-CMC. Blue arrow: Deposited CaHA-CMC in the adjacent muscle tissues. Black arrows: Vasculitis observed in the surrounding fat tissue. | |
Discussion
The development of an ideal injectable and long-lasting material for glottic insufficiency is essential and still ongoing. The vocal fold is a small structure, so the optimal procedure requires precise positioning and capacity. The material should be injected with a fine needle, and there should be no movement or volume change at the injected position.23 Previously tried long-lasting injection materials have many problems. First, the injection materials made by processing autologous biological tissue for augmentation require syringes with thick needles. Autologous tissue transplantation avoids foreign body reaction and rapid absorption. However, the thick needle makes it difficult to inject them into the precise microstructure of the vocal folds. For example, the injection of autologous fat tissue and crushed cartilage requires 19G needles.24–26
Other problems are observed with synthetic injectable materials. Most of these materials consist of a substance for augmentation and a fluid carrier.27,28 However, the carrier solution can be absorbed faster than the augmentation substance. In this situation, the real volume decreases appreciably after injection. Even though the augmentation substances remain in the injected site, a glottic gap can occur due to the volume loss that originates from absorption of the carrier. Additionally, the duration of the filler is dependent upon the absorption rate of the carrier, which cannot be predicted exactly. This makes it difficult to estimate and decide the optimal amount of the injection materials. Therefore, in the clinic, the injection volume has been overcorrected beyond the expected volume, but there has been no standard guideline for the injection volume and clinicians still find it problematic.29 Furthermore, due to the carrier, the augmentation substance can migrate to another site that is not intended. This migration makes the augmentation ineffective even though the materials are precisely injected at the target site and at the optimal dose. Some materials enable gelation in vivo to prevent migration of the augmentation substance, but they require a 20G thick needle for injection.15
We hypothesized that in vivo gelation without a carrier could solve the problems mentioned above. The augmentation substance formed in vivo would not need a thick needle for injection and would avoid problems that originate from the use of a carrier. Because click chemistry enables fast chemical cross-linking of polymers under aqueous conditions, we expected that click chemistry-based gelation in vivo would be suitable for this purpose.30 In this study, we used a 26G needle in animal experiments with PEG hydrogels and with CaHA-CMC. However, our click chemistry-based-PEG hydrogel could be injected through a 30G needle as easily as water. In particular, the PEG hydrogel did not require a special carrier solution for injection, so it could prevent unintended carrier migration from the injection site. After subcutaneous injection in mice and rats, the PEG hydrogel was stably localized at the injection site, but CaHA-CMC spread out into several lumps. Similar findings were observed in the histological examination of rabbit vocal folds. The hydrogels were in close contact with the surrounding tissue due to their good adhesion properties. Several host cells infiltrated at the margin of the hydrogel but not in the center due to the dense cross-linking and the non-degradable hydrogel structure.31 However, such infiltration is not expected to be a major problem for vocal fold augmentation because the injected hydrogel does not need to fill the defect area and degrade but should occupy the space surrounding the tissue for a long time.
The lamina propria of vocal folds is the key structure for vocal fold vibration.32 If the injected material is involved in this structure, there is a possibility that the original viscoelasticity will be changed, which will affect vocal fold vibration. Therefore, the viscoelastic property of the implant material should be similar to that of the vocal folds as far as possible.33,34 However, the injected materials developed so far have a viscoelasticity that differs from the viscoelasticity of the vocal fold. Based on our rheological data and references, the viscoelasticity of the 4 wt% PEG hydrogel is more similar to that of the vocal fold than the viscoelasticity of CaHA-CMC (at 10 Hz, normal vocal fold = about 80 G, CaHA-CMC = 2000 G, 4 wt% PEG = 75 G).35 The viscoelastic similarity is an additional advantage of our click chemistry-based PEG hydrogel.
Conclusions
In summary, we synthesized a click chemistry-based PEG hydrogel and applied it for vocal fold augmentation in vivo. The main advantages of copper-free click chemistry are that it is a fast and specific reaction and does not require a catalyst under aqueous conditions. Therefore, our PEG hydrogel enabled fast gelation in vivo after injection and provided long-term stability, and therefore could be an ideal filler for clinical treatment of vocal fold paralysis. Azide- and DBCO-modified 4-arm PEG molecules were cross-linked with each other by chemical conjugation based on click chemistry, which enabled gelation within several minutes. After subcutaneous injection into mice and rats, the PEG hydrogel showed higher stability after 1 month compared to the traditionally used CaHA-CMC filler. In a rabbit model with vocal fold paralysis, the effects of the PEG hydrogel and CaHA-CMC were also analyzed and compared. Importantly, the PEG hydrogel stably fixed the paralyzed vocal fold close to the midline 4 months after injection and minimized the glottic gap. The PEG hydrogel provided an improved therapeutic result compared to CaHA-CMC, which partially leaked out to the surrounding fat and muscle tissue and had insufficient stability. These overall results demonstrated that click chemistry-based PEG hydrogels show promising potential in the clinical treatment of vocal fold augmentation in vivo.
Conflicts of interest
There are no conflicts of interest to declare.
Acknowledgements
C.-S. K. acknowledges the financial support of the Catholic Medical Center Research Foundation made in the program year of 2019. This work was supported by the Basic Research Program (2016R1C1B3013951 and 2020R1G1A1004280) through the National Research Foundation of Korea (NRF) funded by the Korean government (Ministry of Science, ICT, & Future Planning), a Clinical Research Institute Grant funded by The Catholic University of Korea Daejeon St. Mary's Hospital (CMCDJ-P-2019-003), and The E.N.T. Fund of the Catholic University of Korea made in the program year of 2018.
References
- M. Santos, S. Vaz Freitas, P. Santos, I. Carvalho, M. Coutinho, Á. I. Moreira da Silva and E. S. C. Almeida, Ear, Nose Throat J., 2019, 145561319882116, DOI:10.1177/0145561319882116
.
- P. Krasnodębska, A. Domeracka-Kołodziej, A. Szkiełkowska, A. Panasiewicz, M. Mularzuk, D. Sokołowska-Łazar and K. Wilhelmsen, Otolaryngol. Pol., 2018, 72, 36–44 CrossRef
.
- S. Miller, Otolaryngol. Clin. North Am., 2004, 37, 105–119 CrossRef
.
- E. M. van den Broek, D. E. Vokes and E. B. Dorman, J. Voice, 2016, 30, 221–223 CrossRef
.
- C. A. Rosen, M. R. Amin, L. Sulica, C. B. Simpson, A. L. Merati, M. S. Courey, M. M. Johns 3rd and G. N. Postma, Laryngoscope, 2009, 119(Suppl. 2), S185–S212 CrossRef
.
- P. S. Mallur and C. A. Rosen, Clin. Exp. Otorhinolaryngol., 2010, 3, 177–182 CrossRef
.
- A. A. Halderman, P. C. Bryson, M. S. Benninger and R. Chota, J. Voice, 2014, 28, 631–635 CrossRef
.
- T. K. Kwon, J. E. Lee, W. J. Cha, C. M. Song, M. W. Sung and K. H. Kim, Laryngoscope, 2013, 123, 2491–2496 CrossRef
.
- T. L. Carroll and C. A. Rosen, Laryngoscope, 2011, 121, 313–319 CrossRef CAS
.
- C. A. Rosen, J. Gartner-Schmidt, R. Casiano, T. D. Anderson, F. Johnson, L. Reussner, M. Remacle, R. T. Sataloff, J. Abitbol, G. Shaw, S. Archer and A. McWhorter, Otolaryngol. – Head Neck Surg., 2007, 136, 198–204 CrossRef
.
- R. A. DeFatta, F. R. Chowdhury and R. T. Sataloff, J. Voice, 2012, 26, 614–618 CrossRef
.
- S. R. Caliari and J. A. Burdick, Nat. Methods, 2016, 13, 405–414 CrossRef CAS
.
- O. Z. Fisher, A. Khademhosseini, R. Langer and N. A. Peppas, Acc. Chem. Res., 2010, 43, 419–428 CrossRef CAS
.
- H. Thakar, S. M. Sebastian, S. Mandal, A. Pople, G. Agarwal and A. Srivastava, ACS Biomater. Sci. Eng., 2019, 5, 6320–6341 CrossRef CAS
.
- S. K. Kwon, H. B. Kim, J. J. Song, C. G. Cho, S. W. Park, J. S. Choi, J. Ryu, S. H. Oh and J. H. Lee, PLoS One, 2014, 9, e85512 CrossRef
.
- Z. Huang, P. Delparastan, P. Burch, J. Cheng, Y. Cao and P. B. Messersmith, Biomater. Sci., 2018, 6, 2487–2495 RSC
.
- V. X. Truong, M. L. Hun, F. Li, A. P. Chidgey and J. S. Forsythe, Biomater. Sci., 2016, 4, 1123–1131 RSC
.
- M. G. Raucci, U. D'Amora, A. Ronca and L. Ambrosio, Adv. Healthcare Mater., 2020, e2000349, DOI:10.1002/adhm.202000349
.
- C. A. DeForest and K. S. Anseth, Nat. Chem., 2011, 3, 925–931 CrossRef CAS
.
- H. C. Kolb, M. G. Finn and K. B. Sharpless, Angew. Chem., Int. Ed., 2001, 40, 2004–2021 CrossRef CAS
.
- E. Kim and H. Koo, Chem. Sci., 2019, 10, 7835–7851 RSC
.
- J. C. Jewett and C. R. Bertozzi, Chem. Soc. Rev., 2010, 39, 1272–1279 RSC
.
- M. S. Courey, Otolaryngol. Clin. North Am., 2004, 37, 121–138 CrossRef
.
- J. C. Lee, B. J. Lee, S. G. Wang, C. H. Lee and D. H. Shin, J. Voice, 2012, 26, 515–520 CrossRef
.
- D. P. Hill, A. D. Meyers and J. Harris, Laryngoscope, 1991, 101, 344–348 CrossRef CAS
.
- O. Laccourreye, J. F. Papon, R. Kania, L. Crevier-Buchman, D. Brasnu and S. Hans, Laryngoscope, 2003, 113, 541–545 CrossRef
.
- C. P. Gulka, J. E. Brown, J. E. M. Giordano, J. E. Hickey, M. P. Montero, A. Hoang and T. L. Carroll, Laryngoscope, 2019, 129, 1856–1862 CrossRef CAS
.
- M. R. Homicz and D. Watson, Facial Plast. Surg., 2004, 20, 21–29 CrossRef
.
- L. Sulica, C. A. Rosen, G. N. Postma, B. Simpson, M. Amin, M. Courey and A. Merati, Laryngoscope, 2010, 120, 319–325 CrossRef
.
- N. K. Devaraj, ACS Cent. Sci., 2018, 4, 952–959 CrossRef CAS
.
- Q. Feng, J. Xu, K. Zhang, H. Yao, N. Zheng, L. Zheng, J. Wang, K. Wei, X. Xiao, L. Qin and L. Bian, ACS Cent. Sci., 2019, 5, 440–450 CrossRef CAS
.
- R. W. Chan and M. L. Rodriguez, J. Acoust. Soc. Am., 2008, 124, 1207–1219 CrossRef
.
- T. Caton, S. L. Thibeault, S. Klemuk and M. E. Smith, Laryngoscope, 2007, 117, 516–521 CrossRef CAS
.
- S. A. Klemuk and I. R. Titze, Laryngoscope, 2004, 114, 1597–1603 CrossRef
.
- M. Kimura, T. Mau and R. W. Chan, Laryngoscope, 2010, 120, 764–768 CrossRef CAS
.
Footnotes |
† Electronic supplementary information (ESI) available. See DOI: 10.1039/d0bm01155j |
‡ These authors contributed equally to this work. |
|
This journal is © The Royal Society of Chemistry 2021 |
Click here to see how this site uses Cookies. View our privacy policy here.