DOI:
10.1039/C5RA17182B
(Paper)
RSC Adv., 2015,
5, 90022-90030
Amyloid fibrils as rapid and efficient nano-biosorbents for removal of dye pollutants†
Received
25th August 2015
, Accepted 9th October 2015
First published on 12th October 2015
Abstract
This study demonstrates the promising role of amyloid fibrils as rapid and efficient nano-biosorbents for removal of dye pollutants in water. Amyloid fibrils of hen lysozyme, which are highly ordered protein nanofibers, can be prepared easily in one step under green and mild aqueous conditions. Results of zeta-potential and fluorescence measurements indicate that lysozyme nanofibers bear positive/negative charges and hydrophobic regions along their fibrillar structures. These special structural properties enable lysozyme nanofibers to adsorb the anionic dyes Reactive Black 5 and Acid Blue 29 and the cationic dye Victoria Blue B rapidly and efficiently, presumably through multiple intermolecular interactions (e.g. electrostatic attraction and hydrophobic interaction); the adsorption equilibrium for these dyes can be reached within 15 min, and a dye removal efficiency of over 60% can be achieved in industrial wastewater. Lysozyme nanofibers are also compatible with magnetite nanoparticles to form magnetic nanofibers, which can provide rapid and convenient dye removals through the application of an external magnetic field and maintain high dye removal efficiency (92–99%) after undergoing 20 cycles of desorption.
Introduction
Dyes have been one of the major classes of chemical pollutants in water pollution. Every year, more than 800
000 tons of synthetic dyes are produced.1,2 Artificial dyes have been widely used in textile dyeing, paper printing, colour photography, and in the production of crude oil products. In most industrial activities, about 10–15% dyes are discharged as industrial effluents;3,4 for example, more than 10–200 ppm dyes are found in the wastewater from textile dyeing.5 Such dye-polluted wastewater not only causes eyesores, but also leads to adverse environmental effects; many dyes can reduce sunlight penetration in natural water and hence strongly interfere with the photosynthetic activity of aquatic life. Moreover, many dyes are toxic to aquatic organisms and can bring about health risks to humans (e.g. cancers) through the consumption of polluted water.5,6 Thus, dyes removal in natural water/wastewater is a very important task in the protection of precious natural water and public health.
A variety of biological and chemical methods have been developed for removing dye pollutants. Although bacterial methods are relatively cheap, they are not suitable for many non-biodegradable azo dyes. Moreover, by-products produced from dye degradations may interfere with bacterial growth, thus limiting the dye removal efficiency of bacteria.7,8 Conventional chemical methods, which involve chemical flocculation/coagulation and sedimentation/precipitation, usually require a time-consuming process to remove dye pollutants in wastewater. Fenton oxidation has been developed as a chemical means for decolorizing organic dyes.9–11 This chemical method, however, usually requires the use of dangerous chemicals (e.g. H2O2) and may generate harmful by-products after dye degradations.9,12,13 Membrane-based separation methods, such as reverse osmosis and ultra-filtration, require high pressure conditions and high costs in the removal of dye pollutants.14,15 UV irradiation and ultrasonic irradiation have also been developed as chemical-free methods for dye removals.16
The use of adsorbents is regarded as a promising approach for removing dye pollutants in wastewater because of its direct and efficient dye removal function. Activated carbons have been widely used as adsorbents in removing dye pollutants, but their production usually requires high temperature and hence high energy costs.17 In recent years, extensive research efforts have been directed to the development of synthetic nanomaterials for removing dye pollutants.18–20 While these novel nanomaterials show promising dye removal properties, their preparation methods usually require relatively harsh and/or non-green conditions18–20 and multiple synthetic steps.20 As such, it is highly desirable to further explore other nanomaterials that can be produced by simple methods under green and mild aqueous conditions and have useful structural properties for rapid and efficient removal of dye pollutants.
Herein, we demonstrate the promising function of amyloid fibrils as nano-biosorbents for rapid and efficient removal of dye pollutants, using hen lysozyme fibrils as the adsorbent model and Reactive Black 5, Acid Blue 29 and Victoria Blue B as the dye samples.21 Amyloid fibrils are the hallmarks of amyloid diseases (e.g. Alzheimer's and Parkinson's diseases) in which proteins or peptide fragments aggregate into robust and highly ordered nanofibers with a common cross-β structure formed by the stacking of individual polypeptide main chains through hydrogen bond formations among their peptide groups.22 Amyloid fibrils possess a number of unique advantages in their preparation method and structural properties that render themselves promising nano-biosorbents for rapid and efficient removal of dye pollutants: (1) amyloid fibrils can be easily produced in one step under mild and green aqueous conditions; for example, lysozyme nanofibers can be easily prepared in 20 mM potassium phosphate buffer with 3.0 M guanidine HCl (pH 6.3) at 50 °C;23 (2) amyloid fibrils have nanostructures with pH-dependent +/− charges (from Lys, Arg, Glu and Asp), which allow adsorption of various ionic dyes through electrostatic attraction; for example, our recent study on a colorimetric nanosensor has shown that lysozyme nanofibers carry positive charges in acidic and neutral media and can adsorb negatively charged chromate;24 and (3) amyloid fibrils, including lysozyme nanofibers, usually have exposed hydrophobic regions along their nanostructures, which facilitate the adsorption of dyes with hydrophobic structures (e.g. aromatic scaffolds).25 With these special structural properties (nanostructures with both +/− charges and hydrophobic regions), we reasoned that lysozyme nanofibers can act as rapid and efficient nano-biosorbents for removing ionic dye pollutants by exerting multiple intermolecular interactions (e.g. electrostatic attraction and hydrophobic interaction). This study demonstrates the potential application of amyloid fibrils from various natural/engineered proteins as nano-biosorbents in the removal of dye pollutants in natural water/wastewater.
Experimental
Materials and chemicals
Reactive Black 5, Acid Blue 29 and Victoria Blue B were purchased from International Laboratory (USA). Magnetite [iron(II,III) oxide nanopowder, particle size = 50–100 nm], 8-anilino-1-naphthalenesulfonic acid (ANS), guanidine hydrochloride and hen egg white lysozyme were bought from Sigma-Aldrich (St. Louis, MO). Anhydrous monobasic potassium phosphate was purchased from USB Corporation. All chemicals were of analytical reagent grade and used without further purification. Milli-Q deionized water was used to prepare solution.
Preparation of lysozyme nanofibers
Amyloid fibrils of hen egg white lysozyme were prepared according to the reported method.23 Hen lysozyme (200 mg) was dissolved in 20 mL of 3.0 M guanidine hydrochloride in 20 mM potassium phosphate buffer (pH 6.3). The mixture was stirred for 4 h at 50 °C and then centrifuged at 14
000 rpm for 4 min. Purification of the fibrils was performed by washing with deionized water for 10 cycles. The lysozyme nanofibers were suspended in deionized water and then stored at 4 °C. Transmission electron microscopy (TEM) was performed to characterize the lysozyme nanofibers. A 10 μL portion of the lysozyme nanofibers was placed on a formvar-coated carbon grid, which was then negatively stained with 2% (w/v) phosphotungstic acid (pH 7.0) for 30 s. The grid was then washed with deionized water and air-dried. The lysozyme nanofibers on the carbon were observed using a JEM-2010 transmission electron microscope (JEOL).
Preparation of magnetite-bound lysozyme nanofibers
An aqueous solution of magnetite (10 mg mL−1) was sonicated before mixing with hen lysozyme. The magnetite solution (6 mg) was added to a solution of hen lysozyme (30 mg, volume = 3 mL) with 3.0 M guanidine hydrochloride in 20 mM potassium phosphate buffer (pH 6.3). The mixture was stirred for 4 h at 50 °C and then centrifuged at 14
000 rpm for 4 min. Purification of the magnetite-bound lysozyme nanofibers was performed by washing with deionized water for 10 cycles. The magnetite-bound lysozyme nanofibers were suspended in deionized water and stored at 4 °C.
Characterization of lysozyme nanofibers
The zeta potential of each sample of lysozyme nanofibers was measured by photon correlation spectroscopy using a Malvern Zetasizer 3000 HAS instrument. Lysozyme nanofibers (0.15 mg mL−1) were mixed with 10 mL of deionized water with 1.0 mM NaCl in a 20 mL polypropylene bottle. A series of diluted samples of lysozyme nanofibers (0.15 mg mL−1) at different pH values was then prepared (pH = 3.0, 4.0, 5.0, 6.0, 7.0, 8.0, 9.0, 10.0 and 11.0) by addition of 0.1 M NaOH or 0.1 M HCl. Fluorescence measurements were done on a PerkinElmer LS55B Spectrofluorometer. Excitation and emission wavelengths were 5 nm. A 20 μM solution of 8-anilino-1-naphthalenesulfonic acid (ANS) in 50 mM potassium phosphate buffer (pH 7.0) was first prepared and then mixed with lysozyme nanofibers (0.2 mg mL−1) at pH 7.0. The mixture was excited at 350 nm, and the fluorescence spectrum at the spectral region of 400–650 nm was recorded. For comparison, a solution of 20 μM ANS alone (without lysozyme nanofibers) was also analyzed by the same fluorescence measurement.
Stability studies of lysozyme nanofibers
The stability of lysozyme nanofibers in acidic and alkaline media was studied by incubating lysozyme nanofibers at pH 3.0 and pH 11.0 for 100 days. The structures of the nanofibers were monitored at different time intervals by transmission electron microscopy as described in the section of preparation of lysozyme nanofibers.
Adsorption studies
Determination of adsorption capacities. Adsorption experiments of Reactive Black 5 were conducted by mixing the dye [109 mg L−1 (109 μM), volume = 3.0 mL] with 0.6 mg mL−1 lysozyme nanofibers. The adsorption process was performed at pH 3.0 with stirring at 250 rpm for 15 min at 25 °C. A 1.0 mL portion of the mixture was collected by centrifugation at 14
000 rpm for 2 min. The filtrate was collected and diluted for absorbance measurements at 600 nm using a Varian Cary 4000 UV-Visible spectrophotometer. The concentration of Reactive Black 5 in the filtrate was then determined by making reference to a calibration curve constructed from Reactive Black 5 standards (2.2–10.9 mg L−1). To determine the amount of Reactive Black 5 adsorbed by lysozyme nanofibers, the adsorption capacity (qe) of lysozyme nanofibers (mg g−1) was calculated using the following equation:where V is the volume of the solution (L), Ci is the initial dye concentration (mg L−1), Ce is the dye concentration at equilibrium after adsorption by lysozyme nanofibers (mg L−1), and M is the mass of lysozyme nanofibers used in the dye adsorption (g).Similar experiments were performed on lysozyme nanofibers with Acid Blue 29 and Victoria Blue B to determine the qe values. For Acid Blue 29 [67 mg L−1 (109 μM), pH 3.0, volume = 3.0 mL], absorbance measurements were performed at 600 nm. In the case of Victoria Blue [55 mg L−1 (109 μM), pH 10.7, volume = 3.0 mL], the absorbance signals at 514 nm were recorded.
Langmuir and Freundlich isotherm models. A series of Reactive Black 5 solution was prepared at various initial concentrations (0, 54, 82, 96, 109, 136, 164 and 191 mg L−1, volume = 3.0 mL, pH 3.0). Lysozyme nanofibers (1.8 mg) were added to each solution and stirred for 15 min at 25 °C. The solution fraction of each mixture was collected by centrifugation at 14
000 rpm. The amount of unabsorbed Reactive Black 5 was determined by measuring the absorbance at 600 nm and making reference to a calibration curve constructed from Reactive Black 5 standards (2.2–10.9 mg L−1). The Langmuir isotherm model and the Freundlich isotherm model were used in our dye adsorption studies. The non-linear Langmuir isotherm model is shown below: |
qe = (qmaxKLCe)/(1 + KLCe)
| (2) |
where qe (mg g−1) is the weight of Reactive Black 5 adsorbed per unit weight of lysozyme nanofibers, Ce (mg L−1) is the dye concentration at equilibrium after adsorption, qmax (mg g−1) is the maximum adsorption capacity of lysozyme nanofibers, and KL (L mg−1) is the Langmuir constant related to the affinity of the binding sites.The non-linear Freundlich isotherm model is shown below:
where
n is the Freundlich constant and
KF is the adsorption coefficient.
Similar adsorption experiments were conducted on lysozyme nanofibers with Acid Blue 29 and Victoria Blue B. For Acid Blue 29 (initial concentration = 34, 51, 59, 67, 84, 101 and 118 mg L−1, volume = 3.0 mL, pH 3.0), absorbance measurements were done at 600 nm. In the case of Victoria Blue B, (initial concentration = 28, 41, 48, 55, 69, 83 and 97 mg L−1, volume = 3.0 mL, pH 10.7), the absorbance signals were measured at 514 nm. The qe values at different Ce for both dyes were fitted to the Langmuir isotherm model and the Freundlich isotherm model as described.
Studies of pH effects
A series of Reactive Black 5 solution [109 mg L−1 (109 μM), volume = 3.0 mL] was prepared at different pH values (pH = 3.0, 4.0, 5.0, 6.0, 7.0, 8.0, 9.0, 10.0 and 11.0). Lysozyme nanofibers (1.8 mg) were added to each solution and stirred for 15 min at 25 °C. The solution fraction was then collected by centrifugation at 14
000 rpm for 2 min, and the absorbance at 600 nm measured. The adsorption capacity (qe) of the lysozyme nanofibers for each sample was then determined as described above [eqn (1)]. Similar adsorption experiments were performed on Acid Blue 29 [67 mg L−1 (109 μM), volume = 3.0 mL] and Victoria Blue B [55 mg L−1 (109 μM), volume = 3.0 mL]. For Acid Blue 29, the absorbance signals were recorded at 600 nm. In the case of Victoria Blue B, absorbance measurements were done at 614 nm (pH 3.0–8.0) and 514 nm (pH 9.0–11.0).
Studies of dye removal efficiency
A series of Reactive Black 5 solution [109 mg L−1 (109 μM), volume = 3.0 mL, pH 3.0, T = 25 °C] was added with different amounts of lysozyme nanofibers (final concentration = 0.2, 0.4, 0.6, 0.8, 1.0 and 1.2 mg mL−1). The mixtures were incubated for 15 min and then centrifuged for 2 min at 14
000 rpm. The solution fraction of each mixture was collected, and the concentration of unabsorbed Reactive Black 5 was determined by measuring the absorbance at 600 nm and making reference to a calibration curve constructed from Reactive Black 5 standards (2.2–10.9 mg L−1). The dye removal efficiency was then determined as follows:where Qi is the amount of Reactive Black 5 in solution before adsorption by lysozyme nanofibers (=VCi, in g) and Qe is the amount of Reactive Black 5 in solution after adsorption by lysozyme nanofibers (=VCe, in g).
Similar experiments were conducted on Acid Blue 29 [67 mg L−1 (109 μM), volume = 3.0 mL, pH 3.0, T = 25 °C] and Victoria Blue B [55 mg L−1 (109 μM), volume = 3.0 mL, pH 10.7, T = 25 °C]. The absorbance signals for Acid Blue 29 and Victoria Blue B were recorded at 600 nm and 514 nm, respectively.
Adsorption kinetic studies
A 40 mL sample containing 109 mg L−1 (109 μM) Reactive Black 5 (pH 3.0) was prepared and incubated with 0.6 mg mL−1 lysozyme nanofibers. A 400 μL portion was collected at different time intervals (t = 0, 1, 4, 7, 10, 15, 20, 25, 30, 45, 60, 90, 120 and 180 min) and centrifuged at 14
000 rpm. The concentration of Reactive Black 5 in solution was determined by measuring the absorbance at 600 nm and making reference to a calibration curve constructed from Reactive Black 5 standards (2.2–10.9 mg L−1). The adsorption capacity (qe) at each time interval was determined as described above [eqn (1)]. Similar adsorption kinetic studies were conducted on Acid Blue 29 [67 mg L−1 (109 μM), pH 3.0, volume = 40.0 mL] and Victoria Blue B [55 mg L−1 (109 μM), pH 10.7, volume = 40.0 mL] with absorbance measurements at 600 nm and 514 nm, respectively.
Adsorption/desorption studies of magnetite-bound lysozyme nanofibers
A 3.0 mL solution of Reactive Black 5 [109 mg L−1 (109 μM), pH 3.0] was added with magnetite-bound lysozyme nanofibers (3.0 mg), and the mixture incubated for 10 min at 25 °C. The dye-adsorbed nanofibers were separated from the solution fraction by placing a magnet adjacent to the sample solution. The solution fraction was collected, and the dye-adsorbed nanofibers were added with 3.0 mL of aqueous solution (pH 11.0) for desorption at 25 °C for 10 min. The dye-desorbed nanofibers were then separated from the solution fraction again by placing a magnet next to the reaction vial. The concentration of Reactive Black 5 after adsorption and desorption was determined by absorbance measurements at 600 nm and making reference to a calibration curve prepared from Reactive Black 5 standards (2.2–10.9 mg L−1). Similar adsorption/desorption experiments were conducted for 20 cycles. The dye adsorption efficiency after each desorption was determined by eqn (4) as described in the section of studies of dye removal efficiency.
Similar adsorption/desorption experiments were performed on Acid Blue 29 [67 mg L−1 (109 μM)] with adsorption at pH 3.0 and desorption at pH 11.0 (absorbance measurements at 600 nm) and Victoria Blue B [55 mg L−1 (109 μM)] with adsorption at pH 10.7 and desorption at pH 5.0 (absorbance measurements at 514 nm).
Application studies
Samples from the Dongjiang River and local industrial wastewater were used in our application studies. Each sample (3.0 mL) was added with Reactive Black 5 [final concentration = 109 mg L−1 (109 μM)] and lysozyme nanofibers (final concentration = 0.8 mg mL−1). The whole mixture was incubated at 25 °C. The dye removal efficiency was determined by absorbance measurements at 600 nm as described in the section of studies of dye removal efficiency. Similar dye adsorption studies were also performed on the anionic dye Acid Blue 29 [67 mg L−1 (109 μM), absorbance measurements at 600 nm] and the cationic dye Victoria Blue B [55 mg L−1 (109 μM), absorbance measurements at 514 nm].
Results and discussion
Characterization and stability studies of lysozyme nanofibers
Amyloid fibrils of hen lysozyme were prepared under green and mild aqueous conditions (3.0 M guanidine HCl in 20 mM potassium phosphate buffer, pH 6.3; temperature = 50 °C).23 After incubation, white precipitates were formed. These fine protein aggregates were characterized by transmission electron microscopy (TEM). As shown in Fig. 1, nano-sized fibrillar structures appear. The stability of lysozyme nanofibers in acidic and alkaline media was then studied. To this end, lysozyme nanofibers were incubated at pH 3.0 and pH 11.0 for 100 days, and their structures were monitored by TEM at different time intervals (t = 15 min, 1 day and 100 days). In both cases, the lysozyme nanofibers maintain their fibrillar structures after prolonged incubations in acidic and alkaline media, highlighting the high robustness of lysozyme nanofibers (Fig. S1 in the ESI†).
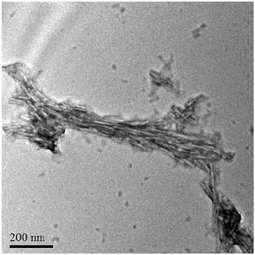 |
| Fig. 1 Transmission electron microscope image of amyloid fibrils of hen lysozyme. Scale bar = 200 nm. | |
We then examined the charge state of lysozyme nanofibers at different pH values by zeta-potential measurements. Lysozyme nanofibers show positive zeta-potential values over the pH range of 3.0–10.0, indicating that they carry positive charges over this wide pH range (Fig. 2). At pH 11.0, lysozyme nanofibers become negatively charged, as indicated by its negative zeta-potential value (Fig. 2). These observations indicate that lysozyme nanofibers are positively charged under acidic and neutral conditions, whereas they become negatively charged in an alkaline medium. The lysozyme nanofibers were further characterized by ANS fluorescence measurements. ANS is a fluorescent molecule which can probe the hydrophobic region of proteins by exhibiting a blue shift in emission wavelength accompanied by enhanced fluorescence.25 Fig. 3 shows the fluorescence spectra of ANS alone and ANS with lysozyme nanofibers. Under aqueous conditions, ANS alone shows a weak fluorescence peak at about 535 nm. In the presence of lysozyme nanofibers, ANS exhibits much stronger fluorescence at 485 nm, indicating that lysozyme nanofibers have exposed hydrophobic regions along their fibrillar structures.
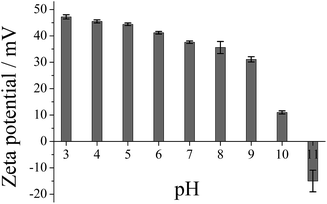 |
| Fig. 2 Zeta potential measurements of lysozyme nanofibers at different pH values. Lysozyme nanofibers (0.15 mg mL−1) were placed in 10 mL of deionized water with 1.0 mM NaCl. Triplicate measurements were performed at each pH value. | |
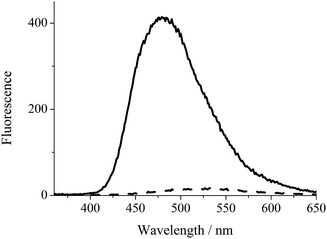 |
| Fig. 3 Fluorescence measurements of ANS in the absence and presence of lysozyme nanofibers. Solid line: fluorescence spectrum of ANS (20 μM) with lysozyme nanofibers (0.2 mg mL−1). Dash line: fluorescence spectrum of ANS alone (20 μM). Excitation and emission slit widths = 5 nm; excitation wavelength = 350 nm. Buffer: 50 mM potassium phosphate buffer (pH 7.0). | |
Dye adsorptions at different pH values
We studied the effect of pH on the adsorption of Reactive Black 5 by lysozyme nanofibers. Fig. 4 shows the chemical structure of the anionic dye Reactive Black 5; Reactive Black 5 consists of an azo-benzyl structure with two –SO4− and two –SO3− groups. Briefly, a series of 109 mg L−1 Reactive Black 5 solution was incubated with 0.6 mg mL−1 lysozyme nanofibers at pH 3.0 to 11.0 for 15 min. After adsorption, the mixtures were centrifuged to pellet down the lysozyme nanofibers, and the solution fractions collected for absorbance measurements at 600 nm to determine the concentration of Reactive Black 5 in each solution. The adsorption capacity (qe) of lysozyme nanofibers towards Reactive Black 5 at each pH was then determined. Fig. 5 shows the adsorption capacities of lysozyme nanofibers over the pH range of 3.0–11.0 towards Reactive Black 5. In general, lysozyme nanofibers have stronger dye adsorption capacity in an acidic medium; lysozyme nanofibers have an adsorption capacity (qe) of 150 mg g−1 at pH 3.0 (Fig. 5), a solution condition under which lysozyme nanofibers become positively charged (Fig. 2). At pH 11.0, lysozyme nanofibers become negatively charged (Fig. 2) and have a much lower dye adsorption capacity (qe = 61 mg g−1, Fig. 5). These observations indicate that electrostatic attraction plays a major role in the adsorption of Reactive Black 5 by lysozyme nanofibers. It is interesting to note that, although lysozyme nanofibers become negatively charged at pH 11.0, they can still adsorb anionic Reactive Black 5 (Fig. 5). This observation implies that other intermolecular interactions may take place in dye adsorption. In this regard, our previous study has shown that lysozyme nanofibers with exposed hydrophobic regions can adsorb the fluorescent dye DTA and cause the dye to exhibit stronger fluorescence accompanied by a blue shift in emission wavelength, a typical observation arising from the hydrophobic interaction between environment-sensitive dyes and hydrophobic structures.25 Thus, when ionic dyes stay in close proximity to fibrillar structures, hydrophobic interaction may become possible and take place in the adsorption process (albeit weaker) in addition to strong electrostatic interaction.
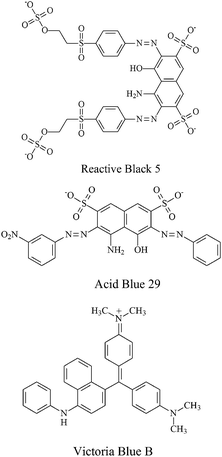 |
| Fig. 4 Chemical structures of the dyes used in this study. | |
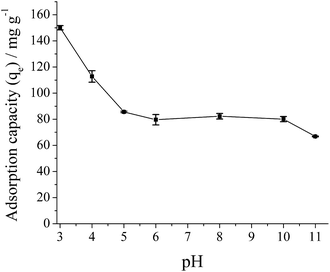 |
| Fig. 5 Adsorption capacities of lysozyme nanofibers with Reactive Black 5 at different pH values. Solution conditions of Reactive Black 5: dye concentration = 109 mg L−1 (109 μM); solution volume = 3.0 mL. Lysozyme nanofibers (1.8 mg) were added to the Reactive Black 5 solution at each pH for dye adsorption. Triplicate measurements were performed at each pH value. | |
Similar adsorption experiments were performed on the anionic dye Acid Blue 29 and the cationic dye Victoria Blue B. For Acid Blue 29, the adsorption of this dye is stronger in an acidic medium (pH 3.0), whereas the adsorption of Victoria Blue B is stronger under alkaline conditions (pH 11.0) (Fig. S2 and S3 in the ESI†).
Rapid dye adsorption by lysozyme nanofibers
To investigate the ability of lysozyme nanofibers to act as rapid adsorbents, we performed an adsorption kinetic experiment for lysozyme nanofibers towards Reactive Black 5. Fig. 6 shows the adsorption capacity (qe) of lysozyme nanofibers with Reactive Black 5 at different time intervals. Surprisingly, lysozyme nanofibers adsorb Reactive Black 5 very quickly (pH 3.0); the qe value reaches 155 mg g−1 at t = 1 min and then remains steady over the time course (t = 1–180 min, Fig. 6). This observation reveals that the dye adsorption equilibrium of lysozyme nanofibers can be reached very quickly. This advantageous property highlights the promising role of lysozyme nanofibers as rapid adsorbents for removing dye pollutants in wastewater treatment. The rapid dye-adsorbing function of lysozyme nanofibers is likely to arise from their electrostatic attraction (and perhaps other intermolecular interactions, such as hydrophobic interaction) towards Reactive Black 5.
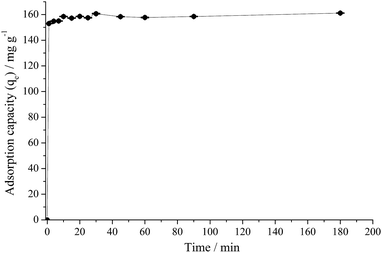 |
| Fig. 6 Kinetic profile of the adsorption of Reactive Black 5 by lysozyme nanofibers. Solution conditions of Reactive Black 5: dye concentration = 109 mg L−1 (109 μM); volume = 40.0 mL; pH 3.0. Lysozyme nanofibers (24 mg) were added to the Reactive Black 5 solution for dye adsorption. The adsorption capacities of lysozyme nanofibers at different time intervals were determined by absorbance measurements on the dye solution before and after dye adsorption. Triplicate measurements were conducted at each time interval. | |
Similar adsorption experiments were also conducted on Acid Blue 29 (pH 3.0). In this case, lysozyme nanofibers can reach their adsorption equilibrium (qe = 101 mg g−1) at t = 4 min (Fig. S4 in the ESI†). For Victoria Blue B, this dye tended to precipitate after a prolonged incubation at high dye concentration at pH 11.0. Thus, we carried out time-course adsorption studies at lower pH (pH = 10.7). Under this condition, lysozyme nanofibers can reach its adsorption equilibrium (qe = 86 mg g−1) at t = 13 min (Fig. S5 in the ESI†).
We tried to further study the dye adsorption kinetics of lysozyme nanofibers using the pseudo-first-order and pseudo-second-order kinetic models. However, the adsorption of Reactive Black 5, Acid Blue 29 and Victoria Blue B by lysozyme nanofibers was so fast that we could not collect enough data in the initial phase of dye adsorption for kinetic studies. Nevertheless, the time-course dye adsorption profile indicates that lysozyme nanofibers can act as rapid nano-biosorbents for removing dyes (Fig. 6, S4 and S5 in the ESI†).
Adsorption studies
We then studied the dye removal efficiency of lysozyme nanofibers by determining the amount of Reactive Black 5 remaining after adsorption using UV-Visible spectrophotometry. Briefly, a fixed amount of Reactive Black 5 (109 mg L−1) was mixed with different concentrations of lysozyme nanofibers (final concentration = 0.2, 0.4, 0.6, 0.8, 1.0 and 1.2 mg mL−1, pH 3.0), and the dye removal efficiency was then determined as described [eqn (4)]. As shown in Fig. 7(a), the dye removal efficiency is about 28% in the presence of 0.2 mg mL−1 lysozyme nanofibers. The dye removal efficiency increases with the amount of lysozyme nanofibers and reaches maximum (∼99%) in the presence of 0.8 mg mL−1 lysozyme nanofibers [Fig. 7(a)]. Beyond this concentration, the dye adsorption efficiency of lysozyme nanofibers remains virtually unchanged [Fig. 7(a)]. Fig. 7(b) and (c) show a sample of Reactive Black 5 before and after adsorption by lysozyme nanofibers. Reactive Black 5 shows intense blue colour before adsorption [Fig. 7(b)]. After adsorption by lysozyme nanofibers, the solution becomes colourless, whereas the lysozyme nanofibers turn blue as a result of the adsorption of Reactive Black 5 [Fig. 7(c)]. These observations highlight the efficient dye-removing function of lysozyme nanofibers.
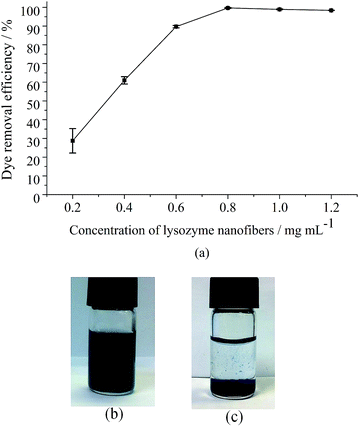 |
| Fig. 7 Adsorption of Reactive Black 5 by lysozyme nanofibers. (a) Removal efficiencies of Reactive Black 5 by different amounts of lysozyme nanofibers (final concentration = 0.2, 0.4, 0.6, 0.8, 1.0 and 1.2 mg mL−1). Lysozyme nanofibers were mixed in 3.0 mL of Reactive Black 5 solution [109 mg L−1 (109 μM)] at pH 3.0. Triplicate measurements were performed at each concentration of lysozyme nanofibers. (b) and (c) show the solution of Reactive Black 5 before and after adsorption by lysozyme nanofibers, respectively. Solution conditions of Reactive Black 5: dye concentration = 109 mg L−1 (109 μM); solution volume = 3.0 mL; pH = 3.0. Concentration of lysozyme nanofibers = 0.8 mg mL−1. | |
To further study the dye adsorption properties of lysozyme nanofibers, we determined the adsorption capacities (qe) of lysozyme nanofibers at different concentrations of Reactive Black 5 and then fitted the qe values and the concentrations of Reactive Black 5 at equilibrium after adsorption (Ce) to the Langmuir isotherm model [eqn (2)] and the Freundlich isotherm model [eqn (3)]. Table S1 in the ESI† shows the results of data fitting from the two isotherm models. The data set of qe versus Ce fits better to the Langmuir isotherm model (Fig. S6 and Table S1 in the ESI†). This observation implies that Reactive Black 5 molecules are likely to be adsorbed onto uniform adsorption sites in a monolayer on lysozyme nanofibers.
Similar adsorption studies were conducted on Acid Blue 29. The dye removal efficiency for Acid Blue 29 (67 mg L−1) increases with the concentration of lysozyme nanofibers (final concentration = 0.2, 0.4, 0.6, 0.8, 1.0 and 1.2 mg mL−1, pH 3.0) and reaches ∼100% with 1.0 mg mL−1 nanofibers (Fig. S7 in the ESI†). The adsorption of Acid Blue 29 by lysozyme nanofibers appears to follow the Langmuir isotherm model, indicating that Acid Blue 29 is adsorbed onto uniform adsorption sites (Fig. S8 and Table S1 in the ESI†).
For Victoria Blue B, the dye removal efficiency increases gradually with the concentration of lysozyme nanofibers (final concentration = 0.2, 0.4, 0.6, 0.8, 1.0, 1.2, 1.4 and 1.6 mg mL−1, pH 10.7) and reaches ∼100% in the presence of 1.4 mg mL−1 nanofibers (Fig. S9 in the ESI†). Lysozyme nanofibers appear to follow the Freundlich isotherm model with Victoria Blue B at pH 10.7 at which lysozyme nanofibers virtually bear zero net charge (pI = 10.8) (Fig. S10 and Table S1 in the ESI†). This observation can be attributed to the fact that lysozyme nanofibers lack electrostatic attraction as the major force for dye adsorption at pH close to their pI, so that Victoria Blue B is adsorbed onto rather heterogeneous adsorption sites on lysozyme nanofibers through non-electrostatic interactions.
The relatively high maximum adsorption capacities (qmax) of lysozyme nanofibers with Reactive Black 5, Acid Blue 29 and Victoria Blue B (>100 mg g−1, Table S1 in the ESI†) indicate that lysozyme nanofibers are strong biosorbents compared to other materials (e.g. activated carbons from Prosopis juliflora bark, activated carbon/Ba/alginate polymer, Aspergillus niger, Penicillium restrictum, acid-treated seaweed Laminaria sp. and Aspergillus foetidus, Table S2 in the ESI†). The strong dye-adsorbing ability of lysozyme nanofibers is presumably due to their nano-sized structures (Fig. 1), which allow efficient dye adsorptions.
Magnetic lysozyme nanofibers as rapid nano-biosorbents
Functionalized magnetic nanoparticles have been widely used to capture target analytes and separate them from sample matrices in various fields (e.g. biochemical studies and removal of water pollutants).26,27 This approach allows target compounds bound to magnetic nanoparticles to be separated rapidly and conveniently by applying an external magnetic field. To prepare magnetic lysozyme nanofibers, we mixed magnetite nanoparticles with hen lysozyme in the preparation of lysozyme nanofibers. To investigate whether magnetite nanoparticles were bound to lysozyme nanofibers, we examined the response of such nanofibers in the presence of an external magnetic field. The responses of lysozyme nanofibers with and without magnetite nanoparticles were monitored by placing a magnet adjacent to the nanofiber samples (Fig. S11 in the ESI†). For lysozyme nanofibers treated with magnetite nanoparticles, they stay close to the magnet, whereas lysozyme nanofibers without magnetite remain suspended in the aqueous solution (Fig. S11 in the ESI†). These results indicate that magnetite nanoparticles can be bound to lysozyme nanofibers to form magnetic lysozyme nanofibers.
The ability of magnetic lysozyme nanofibers to adsorb Reactive Black 5 was then investigated. Briefly, a solution of Reactive Black 5 was mixed with magnetic lysozyme nanofibers for 1 min and then placed adjacent to a magnet. As shown in Fig. 8, the magnetic lysozyme nanofibers turn blue and stay close to the magnet, whereas the aqueous solution becomes clear. This observation highlights the compatibility of lysozyme nanofibers to magnetite and the potential use of magnetite-bound lysozyme nanofibers for rapid and efficient removal of dye pollutants in wastewater treatment. Similar observations were also obtained with Acid Blue 29 and Victoria Blue B. In both cases, magnetite-bound lysozyme nanofibers can adsorb the dyes rapidly, leaving clear solution (Fig. S12 and S13 in the ESI†).
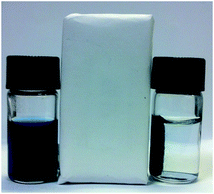 |
| Fig. 8 Adsorption behavior of magnetite-bound lysozyme nanofibers with Reactive Black 5. (Left): Reactive Black 5 solution alone. (Right): Reactive Black 5 solution mixed with magnetite-bound lysozyme nanofibers; the dye-adsorbed lysozyme nanofibers are attracted towards the magnet (white block). Solution conditions of Reactive Black 5: dye concentration = 109 mg L−1 (109 μM); volume = 3.0 mL; pH = 3.0. Amount of lysozyme nanofibers = 3.0 mg. | |
We then investigated the dye removal efficiency of magnetite-bound lysozyme nanofibers after undergoing desorption. To this end, magnetite-bound lysozyme nanofibers underwent 20 adsorption/desorption cycles with Reactive Black 5, Acid Blue 29 and Victoria Blue B, and their dye removal efficiency for each dye at each cycle was determined by absorbance measurements on the dye solution before and after adsorption. Fig. 9 shows the dye removal efficiencies of magnetite-bound lysozyme nanofibers for Reactive Black 5. In this case, the magnetite-bound nanofibers can still maintain high dye removal efficiency (∼99%) after undergoing 20 cycles of desorption. D. Morshedi et al. have reported that protein nanofibrils can remove azo dyes from aqueous solution by coagulation; for example, a high removal efficiency (∼100%) for Reactive Black 5 can be achieved by this approach.28 Dye precipitation can be made faster by addition of NaCl.28 In addition of this chemical method, our work has demonstrated that the incorporation of magnetite nanoparticles can be an alternative physical approach to enabling efficient lysozyme nanofibers to remove dye pollutants from aqueous solution rapidly (Fig. 8 and 9). Similar observations were also obtained with Acid Blue 29 and Victoria Blue B; the dye removal efficiencies of the magnetite-bound nanofibers were found to be 92 and 96% (respectively) after 20 cycles of desorption (Fig. S14 and S15 in the ESI†).
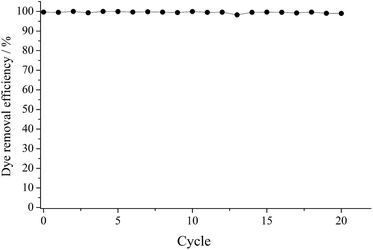 |
| Fig. 9 Dye removal efficiencies of magnetite-bound lysozyme nanofibers with Reactive Black 5 after desorptions. Lysozyme nanofibers (3.0 mg) were mixed with Reactive Black 5 solution [109 mg L−1 (109 μM), volume = 3.0 mL, pH 3.0], separated by an external magnet, desorbed by 3.0 mL of pH 11.0 solution, and then underwent adsorption with Reactive Black 5 [109 mg L−1 (109 μM), volume = 3.0 mL, pH 3.0]. Triplicate experiments were done for each adsorption/desorption cycle. | |
Application studies
To investigate the ability of lysozyme nanofibers to adsorb Reactive Black 5 in real water samples, we conducted dye adsorption studies on river water and industrial wastewater. Briefly, river water and industrial wastewater samples were added with Reactive Black 5 (final concentration = 109 mg L−1) and lysozyme nanofibers (final concentration = 0.8 mg mL−1). The dye removal efficiency of lysozyme nanofibers for each sample was then determined [eqn (4)]. Fig. 10 shows the dye removal efficiencies of lysozyme nanofibers in river water and industrial wastewater; lysozyme nanofibers can directly remove 60 and 66% Reactive Black 5 in river water and industrial wastewater (respectively) without any additional chemical treatment. Lysozyme nanofibers can also directly remove Acid Blue 29 and Victoria Blue B in river water and industrial wastewater with 57–63% and 30–80% dye removal efficiency, respectively (Fig. 10). These observations indicate that lysozyme nanofibers can be used as direct and rapid nano-biosorbents in the removal of dye pollutants in complex natural water/wastewater.
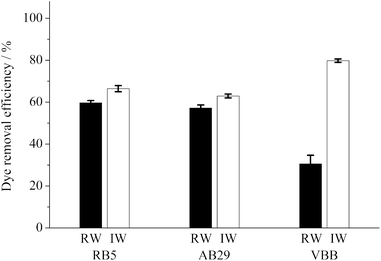 |
| Fig. 10 Dye removal efficiencies of lysozyme nanofibers with Reactive Black 5, Acid Blue 29 and Victoria Blue B in different media. River water (RW) and industrial wastewater (IW) were spiked with each dye and then added with lysozyme nanofibers for dye adsorption. RB5, AB29, and VBB represent Reactive Black 5, Acid Blue 29 and Victoria Blue B, respectively. Dye concentration and volume = 109 μM and 3.0 mL (respectively); amount of lysozyme nanofibers = 2.4 mg. Triplicate experiments were performed for each sample. | |
Conclusions
In conclusion, we have successfully demonstrated with hen lysozyme nanofibers as the model that amyloid fibrils are promising nano-biosorbents for rapid and efficient removal of dye pollutants in water. Unlike other synthetic nanoparticles/nanocomposites that usually require complex and non-green synthetic strategies,18,19 amyloid fibrils can be easily produced in just one step under green and mild aqueous conditions. Moreover, amyloid fibrils are robust protein nanofibers which possess unique structural properties for rapid and efficient removal of dye pollutants; as demonstrated in this study, lysozyme nanofibers carry both positive charges and hydrophobic regions along their nanostructures that enable them to exert multiple intermolecular interactions involving electrostatic attraction and hydrophobic interaction for rapid and efficient dye adsorption. All of these advantageous structural properties enable amyloid fibrils to act as rapid and efficient nano-biosorbents for removing dye pollutants in routine wastewater treatments and immediate removal of dye pollutants in the accidental discharge of dyes to natural water. More importantly, amyloid fibrils have large room to be further developed into “super-nanosorbents” through chemical modifications or protein engineering. In this regards, a possible strategy is to introduce more positively/negatively charged amino acids (e.g. Lys, Arg, Glu or Asp) into individual protein molecules to fabricate highly positively/negatively charged nanofibers. This study has successfully demonstrated an alternative effective way for removing dye pollutants (and perhaps other organic pollutants of environmental concern, such as pesticides) in wastewater/natural water using amyloid fibrils of various natural/engineered proteins.
Acknowledgements
We thank the State Key Laboratory of Chirosciences (4-BBX) and the Research Committee of The Hong Kong Polytechnic University for support to this project.
Notes and references
- K. Z. Elwakeel and M. Rekaby, J. Hazard. Mater., 2011, 188, 10–18 CrossRef CAS PubMed.
- G. Palmieri, G. Cennamo and G. Sannia, Enzyme Microb. Technol., 2005, 36, 17–24 CrossRef CAS PubMed.
- S. Papić, N. Koprivanac, A. Lončarić Božić and A. Meteš, Dyes Pigm., 2004, 62, 291–298 CrossRef.
- K. Selvam, K. Swaminathan and K.-S. Chae, Bioresour. Technol., 2003, 88, 115–119 CrossRef CAS.
- C. O'Neill, F. R. Hawkes, D. L. Hawkes, N. D. Lourenco, H. M. Pinheiro and W. Delee, J. Chem. Technol. Biotechnol., 1999, 74, 1009–1018 CrossRef.
- P. C. Vandevivere, R. Bianchi and W. Verstraete, J. Chem. Technol. Biotechnol., 1998, 72, 289–302 CrossRef CAS.
- M. Işık and D. T. Sponza, Chemosphere, 2004, 55, 119–128 CrossRef PubMed.
- A. K. Golder, N. Hridaya, A. N. Samanta and S. Ray, J. Hazard. Mater., 2005, 127, 134–140 CrossRef CAS PubMed.
- C.-H. Weng, Y.-T. Lin and H.-M. Yuan, Sep. Purif. Technol., 2013, 117, 75–82 CrossRef CAS PubMed.
- M. S. Lucas, M. Algarra, J. Jiménez-Jiménez, E. Rodríguez-Castellón and J. A. Peres, Int. J. Photoenergy, 2013, 2013, 1–6 CrossRef PubMed.
- P. Kajitvichyanukul and N. Suntronvipart, J. Hazard. Mater., 2006, 138, 384–391 CrossRef CAS PubMed.
- M. S. Nawaz and M. Ahsan, Alexandria Eng. J., 2014, 53, 717–722 CrossRef PubMed.
- İ. A. Şengil and M. Özacar, J. Hazard. Mater., 2009, 161, 1369–1376 CrossRef PubMed.
- I. Koyuncu, Desalination, 2002, 143, 243–253 CrossRef CAS.
- I. Petrinić, N. P. R. Andersen, S. Šostar-Turk and A. M. le Marechal, Dyes Pigm., 2007, 74, 512–518 CrossRef PubMed.
- S. Vajnhandl and A. M. le Marechal, J. Hazard. Mater., 2007, 141, 329–335 CrossRef CAS PubMed.
- M. Özacar and İ. A. Şengil, Bioresour. Technol., 2005, 96, 791–795 CrossRef PubMed.
- A. Badruddoza, G. S. S. Hazel, K. Hidajat and M. Uddin, Colloids Surf., A, 2010, 367, 85–95 CrossRef CAS PubMed.
- L. Zhou, C. Gao and W. Xu, ACS Appl. Mater. Interfaces, 2010, 2, 1483–1491 CAS.
- H. Sun, L. Cao and L. Lu, Nano Res., 2011, 4, 550–562 CrossRef CAS.
- K. Hunger, Industrial Dyes: Chemistry, Properties, Applications, 2003 Search PubMed.
- C. M. Dobson, Nature, 2003, 426, 884–890 CrossRef CAS PubMed.
- B. A. Vernaglia, J. Huang and E. D. Clark, Biomacromolecules, 2004, 5, 1362–1370 CrossRef CAS PubMed.
- W.-H. Leung, L. Zou, W.-H. Lo and P.-H. Chan, ChemPlusChem, 2013, 78, 1440–1445 CrossRef CAS PubMed.
- L. Zou, W.-L. Cheong, W.-H. Chung, Y.-C. Leung, K.-Y. Wong, M.-K. Wong and P.-H. Chan, Chem.–Eur. J., 2010, 16, 13367–13371 CrossRef CAS PubMed.
- R. Tietze, S. Lyer, S. Dürr and C. Alexiou, Nanomedicine, 2012, 7, 447–457 CrossRef CAS PubMed.
- J. Gao, H. Gu and B. Xu, Acc. Chem. Res., 2009, 42, 1097–1107 CrossRef CAS PubMed.
- D. Morshedi, Z. Mohammadi, M. M. A. Boojar and F. Aliakbari, Colloids Surf., B, 2013, 112, 245–254 CrossRef CAS PubMed.
Footnote |
† Electronic supplementary information (ESI) available: ESI data of time-course dye adsorption studies, pH effects, adsorption isotherm studies, TEM studies of lysozyme nanofibers in acidic and alkaline media, adsorption/desorption studies of magnetite-bound lysozyme nanofibers. See DOI: 10.1039/c5ra17182b |
|
This journal is © The Royal Society of Chemistry 2015 |
Click here to see how this site uses Cookies. View our privacy policy here.