DOI:
10.1039/D3NH00120B
(Review Article)
Nanoscale Horiz., 2023,
8, 976-990
Nanostructures in Chinese herbal medicines (CHMs) for potential therapy
Received
30th March 2023
, Accepted 15th May 2023
First published on 16th May 2023
Abstract
With its long clinical history, traditional Chinese medicine (TCM) has gained acceptance for its specific efficacy and safety in the treatment of multiple diseases. Nano-sized materials study of Chinese herbal medicines (CHMs) leads to an increased understanding of assessing TCM therapies, which may be a promising way to illustrate the material basis of CHMs through their processing and extraction. In this review, we provide an overview of the nanostructures of natural and engineered CHMs, including extracted CHMs, polymer nanoparticles, liposomes, micelles, and nanofibers. Subsequently, the applications of these CHM-derived nanostructures to particular diseases are summarized and discussed. Additionally, we discuss the advantages of these nanostructures for studying the therapeutic efficacy of CHMs. Finally, the key challenges and opportunities for the development of these nanostructures are outlined.
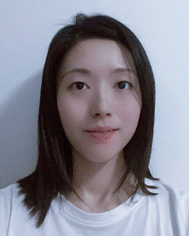
Ya-Li Zhang
| Ya-Li Zhang is currently a postdoctoral fellow at the School of Traditional Chinese Medicine, Beijing University of Chinese Medicine and Key Laboratory for Biomedical Effects of Nanomaterials and Nanosafety, National Center for Nanoscience and Technology of China, Chinese Academy of Sciences. She received her PhD (2021) from Beijing University of Chinese Medicine. Her research focuses on nanomedicine for Chinese herbal medicine, pharmacology and material basis of Chinese herbal medicine. |
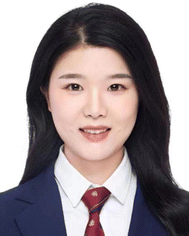
Ya-Lei Wang
| Ya-Lei Wang received her PhD (2022) from Beijing University of Chinese Medicine. She is currently a postdoctoral research fellow at the School of Traditional Chinese Medicine, Beijing University of Chinese Medicine. Her current research interest is focused on cancer treatment through Chinese herbal medicine. |
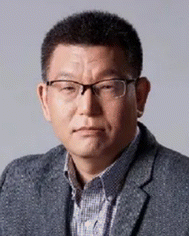
Xing-Jie Liang
| Xing-Jie Liang completed his PhD at the National Key Laboratory of Biomacromolecules, Institute of Biophysics, Chinese Academy of Sciences. He finished his postdoc under the guidance of Dr Michael M. Gottes man, member of NAS and Deputy Director of NIH, for 5 years. Then, he worked as a research fellow at Surgical Neurology Branch, NINDS, NIH. Dr Liang is currently a principal investigator at Center for Excellence in Nanoscience, Chinese Academy of Sciences. He is the current president of the Chinese Association of Nanobiology. His research interests are in elucidating mechanisms to improve drug ability and nanomedicinal bioavailability, and novel strategies to increase therapeutic efficiency. |
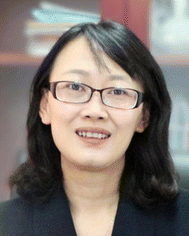
Qian Hua
| Qian Hua completed her PhD at the Institute of Biophysics, Chinese Academy of Sciences and she worked as a postdoctoral fellow at Medical Center, University of California, Davis in 2002–2004. She has been a professor since 2009 and became the Dean of the School of Life Sciences, Beijing University of Chinese Medicine in 2020. Her research focuses on traditional Chinese medicine for prevention and treatment of Alzheimer's disease, nanomedicine for Chinese herbal medicine, pharmacology and material basis of Chinese herbal medicine. |
1. Introduction
The effectiveness of Traditional Chinese medicine (TCM), whose unique theoretical system was established in ancient China some 2000 years ago, for the treatment of multiple diseases has been demonstrated with recent clinical and scientific studies.1–4 The long history of TCM clinical practice has led to plentiful Chinese herbal medicines (CHMs) that exhibit in vivo efficacy and safety, characterized by multiple components acting on multiple targets, some of which are heading to clinics worldwide.5 CHMs are recognized as complementary and alternative remedies for diseases attacking the nervous system, digestive system, endocrine system, and skin.6–8 However, this “multi-component, multi-target” feature of CHMs makes it challenging to assess TCM therapies because the material basis is unclear.
Several attempts have been made to clarify the material basis of CHMs. The pharmacological effect is determined by active fractions of CHMs, which have been widely researched and used in many fields.9 Significant results have been obtained with CHMs developed by implementing the theory of “one target, one drug” associated with Western therapies, through which a single target is bound to a drug with high affinity and specificity.10 Indeed, multiple ingredients derived from CHMs have been developed into medicines that have been approved by the FDA, such as paclitaxel (PTX), camptothecin (CPT), podophyllotoxin, vincristine, vinblastine, and artemisinin.11,12 Benefiting from these successful cases, CHM-derived drugs, especially in the form of nanomedicines, have attracted considerable attention.13 However, due to the “multi-component, multi-target” feature of CHMs, the role of the material in treatment efficacy is not fully reflected. For a better understanding of the CHM effect, high-throughput activity screening, computational methods, network pharmacology, and chinmedomics have been developed, although these techniques have certain disadvantages and application limitations.14
In recent years, with the development of nanobiology, nanomedicine, and supramolecular chemistry15,16 as well as the breakthrough of various electron microscopy and imaging technologies, the characteristics of CHMs have been revealed, greatly expanding the understanding of the material basis of CHMs, which cannot be explained from the perspective of chemical components alone. For example, puerarin, berberine (BBR) and baicalin (BA), the active components of the classic Gegen Qinlian decoction, are characterized by poor solubility and low oral bioavailability. In the presence of licorice, the dissolution and bioavailability of the above components are significantly increased because amphipathic glycyrrhizic acid (GA), the main constituent of licorice, and insoluble components form micelles with increased solubility.17 CHMs are usually taken orally, and boiling CHMs seems to convert bioactive compounds with low bioavailability or dissolution to other component assemblies, which indicate that nanostructures may illustrate the material basis of CHMs.18 Thus, nanotechnology can be applied as an effective way to modernize and internationalize TCM.19 Here, we summarize a representative number of nanostructures that are extracted or engineered from CHMs and discuss the applications of these nanostructures to the treatment of particular diseases (Fig. 1), providing reliable evidence for CHM nanomaterials as potential nanomedicines.
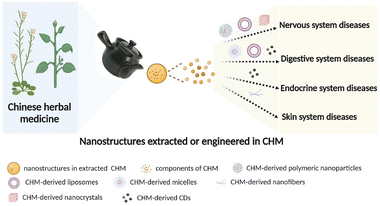 |
| Fig. 1 Schematic of CHM-derived materials with different nanostructures for various diseases (figure was created with Biorender.com). | |
2. Nanostructures in extracted CHMs
Emerging nanotechnologies provide opportunities for the development of CHMs, leading to the discovery of more nanostructures, including nanoaggregates with biological activity, carbon dots (CDs), and self-assembled nanoparticles with clearly defined components,20–23 which benefit from the traditional boiling process. In this part, we introduce the nanostructures of extracted CHMs, which are nanocarrier-free and can be categorized into mixed components and clearly defined components.
2.1 Nanostructures with mixed components in CHM extraction
According to TCM theory, Chinese herbal formulae are typically composed of two or more CHMs in appropriate proportions, which are jointly decocted. The decoction is commonly used in the clinic and is prepared by boiling the CHMs in water for at least 1 h. The CHMs are soaked in cold water before they are heated to ensure sufficient interactions between the components of CHMs for therapeutic efficacy,24 which generates a complicated system consisting of solutes, aggregates, colloids, and precipitates.25,26 The discovery of nanoparticles in decoctions may offer a new perspective for understanding the efficacy of CHMs and even developing a new nanomedicine approach.
A growing body of evidence indicates that biologically active nanometer aggregates exist in CHMs. For example, in a previous study investigating 60 CHMs and 24 Chinese herbal formulae, nanometer aggregates are found in all 84 solution mixtures.27 Moreover, nanometer aggregates with sizes of approximately 100
nm are separated from Bai-Hu-Tang decoction, known to produce an antipyretic effect, and these aggregates are readily taken up by cells.21 Similarly, colloidal nanoparticles with sizes of 50 to 150 nm can be separated from Ma-Xing-Shi-Gan-Tang decoction, and these colloidal nanoparticles are composed of principle ingredient ephedrine (99.7%) and pseudoephedrine (95.5%), which may have a profound impact on the bioavailability and bioactivity of alkaloids in CHMs.23 An investigation into the mechanism by which bioactive molecules in CHMs aggregate into nanoparticles, for example Pueraria lobata (Willd.) Ohwi (PUE), found that four major flavones exist as aggregates in PUE decoction and that the in vivo bioactivity of PUE decoction is related to the aggregates.28 Moreover, a recent study showed that the decocting time of Coptidis Rhizoma—Scutellariae Radix affects the formation of nanostructures and consequently the antibacterial activity.29
Paozhi processing imparts CHMs with the desired properties for medical applications, of which the preparation of charcoal medicines is common.24,30,31 CDs have been found in Artemisiae argyi Folium Carbonisata aqueous extracts, showing a size distribution in the range of 6.0–10.0
nm which has a good therapeutic effect in the treatment of frostbite and thus potential clinical applications.32 Moreover, most CDs derived from CHMs alleviate inflammation, which is a possible mechanism of action of CDs. Additionally, CDs derived from Crinis Carbonisatus (carbonized human hair) (Fig. 2A) provide neuroprotection against cerebral ischemia and reperfusion injury,33 while CDs extracted from Radix Sophorae flavescentis and Glycyrrhizae Radix et Rhizoma Carbonisata exert a protective effect in the treatment of acute gastric ulcers caused by ethanol,34,35 and CDs prepared from Cortex Phellodendri Chinensis exerted a protective effect on imiquimod-induced psoriasis of mouse skin.36 These findings provide novel strategies for studying the active components of charcoal medicines.
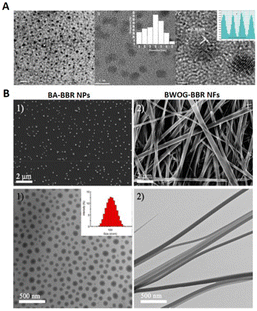 |
| Fig. 2 Nanostructures extracted from CHMs. (A) Transmission electron microscopy (TEM) images of CDs from Crinis Carbonisatus (CrCi-CDs) at different magnifications. CrCi-CDs are spherical and uniformly dispersed, with diameters ranging from 3.2 to 8.8 nanometers with a lattice spacing of 0.203 nanometers. Reproduced from ref. 33 with authorization. Copyright 2022, Springer Nature. (B) Morphologies of self-assembled WOG–BBR (wogonoside-berberine) and self-assembled BA–BBR (baicalin–berberine). The top row shows scanning electron microscopy (SEM) images and the bottom row shows TEM images of BA–BBR and WOG–BBR. The diameters of BA–BBR NPs are approximately 100 nm and those of WOG–BBR NFs range from 50 to 100 nm, and the lengths are beyond tens of micrometers. Reproduced from ref. 38 with authorization. Copyright 2019, American Chemical Society. | |
2.2 Nanostructures of natural small molecules from CHMs
Boiling processing of CHMs makes it possible for two or more natural small molecules to self-assemble. This simple strategy for generating nanostructures reveals several phenomena of boiling CHMs. BBR, the major antibacterial compound of Coptidis rhizoma, is a natural hydrophobic cation that can self-assemble with flavonoid glycoside BA and wogonoside (WOG) derived from Scutellaria baicalensis Georgi. The results show that BA–BBR nanoparticles and BA–WOG nanofibers provide different antibacterial effects, meaning that the different nanostructures of CHM active ingredients play a key role in bioactivity (Fig. 2B).37,38 Similar results are reported for rhein and coptisine (Rhe–Cop) nanofibers and emodin and coptisine (Emo–Cop) nanoparticles, which form through electrostatic attraction, hydrogen bonding, and π–π stacking.39 BBR and aristolochic acid can also self-assemble into linear heterogenous supramolecules through electrostatic attraction and π–π stacking, which decreases the toxicity of aristolochic acid-induced acute kidney injury.40
Single components of CHMs also undergo self-assembly. Licorice, the root and rhizome of Glycyrrhiza uralensis Fisch., is an important CHM that is commonly used to cure multiple diseases in Europe and Asia41 and GA is the major constituent of licorice. Owing to its hydrophobic and hydrophilic moieties, GA shows self-assembly behavior in water to form nanofibers, which can be used to create functional hybrid materials, implying that the solubilizing result of licorice may be attributed to GA self-assembly behavior.42 Compared with the equivalent free drug, rhein hydrogels self-assembled through noncovalent interactions have long-lasting effects and reduced cytotoxicity.43 Moreover, nanoparticles existing in CHMs inspire a more simple formulation, such as dehydrotrametenolic acid (DTA) self-assembled nanoparticles for oral drug delivery18 and betulinic acid (derived from E. ulmoides) nanoparticles for efficient penetration of the blood–brain barrier (BBB).44
Nanocrystals are known to enhance dissolution, and almost 100% of drugs are stabilized by surfactants or polymeric steric stabilizers, which can solve the poor solubility of some CHM compounds.115,116 Baicalein (BE), derived from Radix Scutellariae, has restricted its clinical applications because of the poor solubility, dissolution rate, and oral absorption. To compensate for these disadvantageous properties, high pressure homogenization can be implemented to produce baicalein–nicotinamide (BE–NCT) nano-cocrystals with a dissolution rate and bioavailability that are better than those of BE coarse powder, BE–NCT cocrystals, or BE nanocrystals (Fig. 3A and B).45 Similarly, the solubility and oral bioavailability of BA–BBR complex nanocrystals, also prepared through high pressure homogenization, are improved over those of BA and BBR.46 Oridonin (derived from Rabdosia rubescens) nanocrystals (ORI-NCs), prepared through the anti-solvent precipitation method, have increased solubility, which enables the transportation of ORI-NCs through the Madin-Darby canine kidney monolayer in a complete form.47 Resveratrol nanocrystals (RES-NCs) are similarly prepared, with hydroxypropyl methylcellulose as the stabilizer, to improve the oral absorption and delivery of RES-NCs crossing the BBB for the treatment of Parkinson's disease (PD).48 Andrographolide (AG), derived from Andrographis paniculata (Burm. f.) Nees, is another poorly soluble drug, which can be loaded into a nanocrystal-based solid dispersion (NC-SD) with superdisintegrants through both homogenization and spray-drying to provide a rapidly dissolving, high drug-loaded, surfactant-free NC-SD.49 Moreover, puerarin (derived from Pueraria lobata (Willd.) Ohwi) and BA nanocrystals prepared through high pressure homogenization have increased solubility and bioavailability.50,51
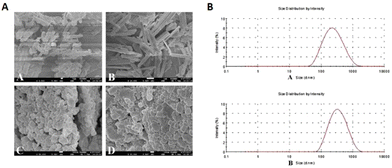 |
| Fig. 3 CHM-derived nanocrystals. (A) BE coarse powder, BE–NCT cocrystals, BE nanocrystals, and BE–NCT nano-cocrystals at various magnifications are shown in SEM images from left to right and top to bottom. BE–NCT cocrystals exhibit a significant change in rod shape as compared to BE coarse powder. BE: baicalein, BE–NCT: baicalein–nicotinamide. (B) BE nanocrystals and BE–NCT nanocrystals have small particle sizes (less than 300 nm). Reproduced from ref. 45 with authorization. Copyright 2018, Elsevier. | |
3. Engineered nanostructures of CHMs
Considering the inherent advantages of CHMs, natural compounds from CHMs have been engineered as nanomaterials to be delivered or be nanocarriers, such as polymer nanoparticles and liposomes, which enhances targeting specificity, bioavailability, and solubility, thus increasing the therapeutic efficacy in the treatment of various diseases.52 Research suggests that these nanostructures are crucial for therapeutic efficacy and drug delivery.53
3.1 CHM-derived polymer nanoparticles
As carriers, polymer nanoparticles can be prepared from biocompatible and biodegradable polymers13,54 to increase therapeutic efficacy while minimizing toxicity.55 Moreover, carriers can increase the distribution of drugs at the target and display synergistic effects.56 This part mainly includes CHM-derived polymers and dendrimers. Micelles will be presented separately.
3.1.1 Increase of targeting distribution.
To enable Ginkgolide B (GB) to cross the BBB for treating PD, biodegradable poly(ethylene glycol)-b-poly(trimethylene carbonate) (PEG–PTMC) nanoparticles (PPNPs) are used to encapsulate GB (GB-PPNPs), which are found in the plasma and brain in high concentrations, thus improving the related indexes of the animal model of PD.57 Curcumin (CUR), derived from Curcuma longa, is a neuroprotective agent with poor brain bioavailability. This problem is solved by preparing CUR-encapsulated PLGA nanoparticles, which are internalized into hippocampal neural stem cells to induce neurogenesis, representing a new strategy for treating neurodegenerative diseases.58 This method is also applied to deliver rhynchophylline, derived from Uncaria rhynchophylla (Miq.) Miq. Ex Havil.59
Local treatment has the advantage of enhancing drug accumulation at the target site. For example, BSP-MNs-QUE@HSF/CDF, the resolvable microneedles are prepared by coating a diphenyl carbonate crosslinking cyclodextrin metal organic framework comprising over 26% quercetin with a hypertrophic scar fibroblast membrane, and then dispersing in Bletilla striata polysaccharide. This biomimetic functional selective drug delivery system strengthening the effects of drugs on hypertrophic scars, together with Bletilla striata polysaccharide, exerts a synergistic effect.60 A carrier is designed to deliver multiple dosages of celastrol (an amphiphilic polymer–celastrol conjugate), which shows efficient mitochondria targeting capacity, thus exerting remarkable anticancer effects against various cancer cells.61 Moreover, shikonin (SK), derived from Lithospermum erythrorhizon, is encapsulated in pH-responsive colitis-targeting nanoparticles, composed by chitosan, hyaluronic acid, and pH-responsive SK-loaded Eudragits S100 polymer, and the resulting system shows significant colitis-targeting ability after oral gavage.62 Ginsenoside Rg3 is incorporated into a reactive oxygen species (ROS)-responsive PEG-b-PPS delivery system, which is administered locally by intramyocardial injection to ameliorate cardiac function and decrease the infarct size (Fig. 4A–E).63 Polysaccharides from CHMs, such as Angelica sinensis polysaccharide,64–66Chinese yam polysaccharide67,68 and Ramulus mori polysaccharide,69 can also be developed into polymer nanoparticles to improve the immune response. Angelica sinensis polysaccharide (ASP)-loaded PLGA nanoparticles are encapsulated by polyethylenimine (PEI) to form the ASP–PLGA–PEI system, which is subsequently used to activate macrophages and thus increase antigen uptake by macrophages.66 Beyond that, PLGA nanoparticles encapsulating Chinese yam polysaccharide can be converted into an oil in water (O/W) Pickering emulsion for high antigen loading.68
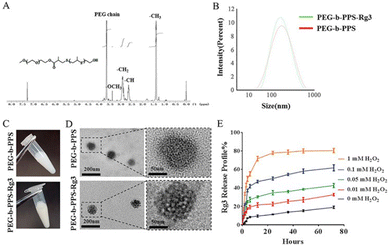 |
| Fig. 4 PEG-b-PPS-Rg3 nanoparticle characterization and release profile. (A) PEG-b-PPS 1H NMR spectrum. 1.30–1.40 ppm (-CH3 in PPS chain), 2.6–2.7 ppm (–CH in PPS chain), 2.80–3.0 ppm (-CH2 in PPS chain), 3.25–3.35 ppm (-OCH3), and 3.5–3.75 ppm (-OCH3) (PEG chain protons). (B–D) Size distribution, appearance and TEM image of PEG-b-PPS and PEG-b-PPS-Rg3. (E) PEG-b-PPS-Rg3 release profiles in the presence of 0, 0.01, 0.05, 0.1, and 1 mM H2O2. Reproduced from ref. 63 with authorization. Copyright 2020, Elsevier. | |
3.1.2 Synergistic effect.
Multi-drug chemotherapy is commonly used to treat malignant tumors. For example, biodegradable PCEC nanoparticles are applied for the co-delivery of PTX and CUR.70 PTX and quercetin are encapsulated in hybrid polymer nanoparticles to decrease the multidrug resistance of tumors,71 and PTX is combined with psoralen-loaded polymer lipid nanoparticles to exert a synergistic effect in the treatment of triple-negative breast cancer.72 Anticancer triptolide and celastrol are isolated from Thunder God Vine and loaded on silk fibroin using a modified desolvation method, which enabled them to exert synergistic effects against human pancreatic cancer cells.73 Moreover, chitosan–gelatin linked EGCG (CGE) is developed as a nanocarrier of siRNA si-TMEM44-AS1, which synergistically reversed 5-FU resistance in gastric cancer.74
3.2 CHM-derived liposomes
Liposomes are biocompatible vesicles composed of lipid bilayers. They have been used to improve the bioavailability of encapsulated agents, target specific sites, and overcome some limitations of CHM applications since the 1980s.13
3.2.1 Increase in bioavailability.
Tripterin (TP), derived from Thunder God Vine, has anti-inflammatory and anti-infective effects but poor solubility, which can be solved by encapsulating TP in liposomes (Fig. 5A–D). The vitro and vivo experiments showed that TP-encapsulated liposomes can mitigate SARS-CoV-2 replication and inflammation.75 Patchouli alcohol, derived from Pogostemon cablin (Blanco) Benth., has the same problem as TP. Because of its potential drug candidate for inflammatory bowel disease (IBD), patchouli alcohol is developed into lactoferrin-modified liposomes, which can bind specifically to LRP-1 that functionalized colonic macrophages, representing an effective and safe therapeutic strategy.76 Similarly, using liposomes as carriers can enhance the oral bioavailability and efficacy of encapsulated liquiritin,77 quercetin,78 and aloe emodin.79
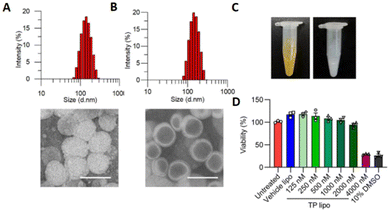 |
| Fig. 5 Characterization of TP lipo. (A and B) TEM images of TP lipo (left) and vehicle lipo (right). The sizes are approximately 134.3 nm for TP lipo and 142.0 nm for vehicle lipo, scale bar = 200 nm. (C) Images of TP lipo and Vehicle lipo. (D) The effects of vehicle lipo, TP lipo at various doses, and 10% DMSO on the viability of Vero E6 cells. TP lipo: tripterin liposomes. Reproduced from ref. 75 with authorization. Copyright 2019, Springer Nature. | |
Increasing the half-life of CHMs is another strategy to improve bioavailability.80 A novel multifunctional liposome system incorporating PTX and ginsenoside Rg2, Rg3, and Rg5, which can function as both a chemotherapy adjuvant and functional membrane, has an increased blood circulation time, thereby improving the stability and half-life of encapsulated drugs for delivery to the tumor site.3 Rhodojaponin III (RJ-III), derived from Rhododendron molle G. Don, is a powerful analgesic that is restricted by its acute toxicity and poor pharmacokinetics. RJ-III loaded into hydroxypropyl trimethyl ammonium chloride chitosan (HACC)-modified solid lipid nanoparticles (RJ-III@HACC-SLNs) has a lower toxicity and higher peak time and half-time than free RJ-III.81
3.2.2 Enhancement of target specificity.
Liposomes have been extensively applied as drug delivery agents, particularly for targeted therapy. Celastrol is an anti-inflammatory ingredient that can inhibit the activation of dendritic cells (DCs) and subsequently suppress the response of T cells.82 To increase its target specificity, celastrol is loaded into mannose-modified liposomes which could target mannose receptors on DCs, thereby improving the symptoms of imiquimod-induced psoriasis on mouse skin.83 Honokiol (HNK), derived from Houpoea officinalis, is encapsulated in a novel nanocarrier, namely liposomes synthesized by hyaluronic acid–phospholipid conjugates (HA-DOPE) (HA-DOPE@Lips/HNK), to enhance its solubility and target therapy owing to the HA impact on CD44 which showed a novel nanocarrier to enhance drug delivery in osteosarcoma treatment.84 Additionally, ginsenoside Rg3-based liposomes are characterized by high in vitro cellular uptake, penetration of glioma spheroids, and in vivo active glioma targeting.85
3.2.3 Co-delivery of drugs.
Ginsenoside Rg3-based liposomes loaded with docetaxel (Rg3-Lp/DTX) can deliver both R3 and docetaxel as well as capture more Glut1 overexpressed in circulating tumor cells and reverse the immunosuppressive microenvironment to inhibit the formation of metastatic niches through the Rg3 effect.86 The poor water solubility and low oral bioavailability of CUR restricts the bioavailability of CUR, and thus a liposomal formulation of pegylated RGDK-lipopeptide is developed to co-encapsulate anti-cancer drugs DOX and CUR, a combination of both hydrophilic and hydrophobic compounds, as anticancer agents.87 The same strategy as Timosaponin AIII (TAIII, derived Anemarrhena asphodeloides Bunge), it could stabilize the phospholipid bilayer and show synergistic antitumor effects with DOX on hepatocellular carcinoma (HCC) cells in a TAIII-based liposomes carrying DOX system.88
3.3 CHM-derived micelles
Micelles, which are typically responsive block copolymers, can self-assemble to form nanospheres with a hydrophilic core and a hydrophobic coating.90,91,94 This common nano drug delivery system has been widely used to carry many CHM-derived small-molecule drugs with a superior safety profile.89
3.3.1 Improvement of solubility.
Many anti-cancer drugs, including CUR and resveratrol, are poorly soluble in water. CUR is loaded into alendronate–hyaluronic acid–octadecanoic acid to form micelles, which can be released in a sustained manner and has a better effect on osteosarcoma bearing mice than free drugs (Fig. 6A–D).90 Besides, rubusoside-based micelles can enhance the solubility of CUR and/or resveratrol, and these insoluble drugs together increase the anti-cancer effect compared with the single drug.91 Supersaturated drug delivery systems (SDDS) are another strategy for increasing drug solubility, such as silybin (derived from Silybum marianum). It is incorporated into a complex system of Soluplus–Copovidone (Soluplus-PVPVA), which can sustain a stable condition of the supersaturated solution and promote oral absorption.92 The well-known anti-cancer drug PTX is insoluble, which limits its application, requiring the development of several formulations, such as multi-functional chitosan polymer micelles,93,94 amphiphilic carboxymethyl chitosan (CMCS)–quercetin conjugate,95 and CMCS–rhein polymer micelles.96,97 Magnolol (derived from Magnolia officinalis) is designed with Soluplus® and Poloxamer 188 to improve its poor solubility and oral bioavailability.98,99
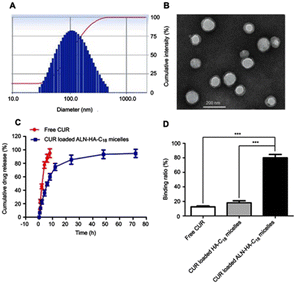 |
| Fig. 6 (A) The distribution of sizes (118 ± 3.6 nm) and (B) the image captured by TEM of micelles loaded with CUR in ALN-HA-C18 formulation. (C) In vitro release patterns of free CUR and loaded CUR ALN-HA-C18 micelles in 1% Tween 80 PBS solution. (D) The binding ratio of CUR, CUR-loaded HA-C18 micelles and CUR-loaded ALN-HA-C18 micelles to HA. ALN-HA-C18: alendronate–hyaluronic acid–octadecanoic acid. Reproduced from ref. 90 with authorization. Copyright 2019, Dove Press. | |
3.3.2 Active targeting to inflammation.
CHM-derived micelles are widely used in liver targeting. Resveratrol is designed as ROS-responsive micelles, which penetrated hepatic stellate cells for delivery into the target organ to treat liver fibrosis, demonstrating the great potential of CHM-derived micelles for targeting liver diseases.100 The same function of ROS-responsive micelles is applied to target hepatoma by loading celastrol into a glycyrrhetinic acid-modified CMCS–thioketal–rhein conjugate101 and to target inflammatory bowel diseases by incorporating aryl boronic ester as a responsive linker of covalently linked quercetin and biocompatible glycol chitosan.102 Vinegar baked Radix Bupleuri (VBRB) has the ability to guide drugs to the liver, and thus it is co-administered with 10-hydroxycamptothecin (HCPT)-loaded polymer micelles to enhance HCPT liver targeting.103 Additionally, Xanthatin, derived from Xanthium strumarium L., is developed into a DC-specific targeting delivery system to form a polymer micelle that could treat the refractory allergic rhinitis.104
3.4 CHM-derived nanofibers
The unique characteristic of nanofibers makes them widely applied in the biomedical and healthcare field.105 Yunnan Baiyao has been popular in China as a CHM for treating wounds for over 100 years and has uniform nanofibers which may have a key role in its function of healing wounds.106 In the current study, many natural small molecules from CHMs have been developed into nanofibers to obtain a better effect.
3.4.1 Control of drug release.
Anemoside B4 (ANE), derived from Pulsatilla, is loaded into a chitosan and polyvinyl alcohol polymer blend (CS–PVA–ANE) by blend-electrospinning for full-thickness diabetic wound repair by controlled drug release.107 To achieve a similar outcome with CUR, CUR nanocrystals are encapsulated in polydopamine (PDA) to form nanofibers (PDA/Cur NFs). Under laser irradiation, encapsulated CUR is released from the PDA shell, and thus PDA/Cur NFs undergo photothermal conversion to exert an antibacterial effect (Fig. 7A–E).108 In another simple strategy, CUR is loaded into a sandwich-like nanofibrous membrane (CSNM) to provide a hemostatic effect.109
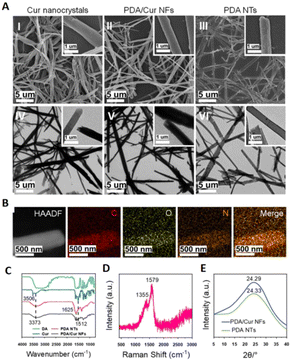 |
| Fig. 7 (A) SEM and TEM images of Cur nanocrystals, PDA/Cur NFs and PDA NTs. (B) PDA/Cur NF elemental mapping images. (C) DA, Cur, PDA NTs, and PDA/Cur NFs FTIR spectra. (D) PDA/Cur NFs Raman spectrum. (E) PDA NTs and PDA/Cur NFs XRD patterns. PDA stands for polydopamine, DA stands for dopamine and NTs stands for nanotubes. Reproduced from ref. 108 with authorization. Copyright 2018, Springer Nature. | |
3.4.2 Increase of efficacy.
The active ingredient of Garcinia hanburyi Hook. f. is gambogic acid, which has an effect on resisting heating-induced damage for cells. It is loaded into pH-responsive PEG-modified Mn2+/indocyanine green (Mn-ICG@pHis-PEG) to realize low-temperature photothermal therapy (PTT), resulting in an outstanding tumor damage effect.110 Electrospun fibrous membranes can be used as an artificial periosteum. For example, poly(ε-caprolactone) (PCL)/gelatin nanofibers can release encapsulated icariin (ICA), derived from Epimedium brevicornum Maxim, to accelerate bone regeneration by stimulating cell attachment, proliferation, and differentiation.111 In addition, celastrol is incorporated into TiO2 nanofibers to enhance immunity and antitumor activity.112 Astragaloside IV (AT) and ferulic acid (FA), derived from Angelicae sinensis radix, are drugs that promote microvessel formation. AT and FA loaded into fragmented nanofibers, an injectable format, have a higher tissue retention than the free drugs after local injection.113
3.5 Others
There are also other CHM-derived nanostructures, such as inorganic nanoparticles and exosomes. TSIIA@SeNPs-APS with sizes of 80 nm consist of Astragalus Polysaccharidesis (APS, derived from Astragali Radix) and tanshinone IIA (TSIIA, derived from Salvia miltiorrhiza Bunge) based on SeNPs, which could improve the retention time and have the synergistic effect of APS and TSIIA.114 A similar strategy as TSIIA@SeNPs-APS, MPE-SeNPs with around 120 nm in particle size showed slow and steady drug release in the simulated digestive fluid, with mulberry leaf and Pueraria Lobata extract-loaded SeNPs.115
CHM-derived exosome-like nanoparticles (ELNs) show both excellent promising biotherapeutics and drug carriers.116 HJT-sRNA-m7 isolated from Hong Jing Tian (HJT, Rhodiola crenulata) effectively decreased the expression of fibrosis marker genes and proteins in vitro in alveoli and in vivo in mouse lung tissues and lipids in HJT decoction may form liposomes with sRNAs to enter human alveolar and gastric cells, which provided an original therapeutic strategy for the oral delivery of sRNA.117 Furthermore, exosome-like nanoparticles extracted from HJT have more therapeutic effects than the decoctions both in vitro and in vivo.118 Ginger exosome-like nanoparticles (GELNs) have the effect of inhibition pathogenicity of Porphyromonas gingivalis and GELNs inhibited bacterial growth by GELN lipids binding to Hemin-Binding Protein 35 from the Outer Membrane of Porphyromonas gingivalis.119 Beyond that, the exosome also could be used as CHM therapeutic drug carriers, for example as exosome encapsulated-CUR,120 baicalin, hederagenin and neferine.121
4. Therapeutic applications of CHM-derived nanostructures
In fact, most bioactive compounds from CHMs have limited bioavailability owing to their poor stability or solubility. Nonetheless, these compounds can exert pharmacological effects following the CHMs boiling process or other processing methods.24 Therefore, to treat various diseases, such as nervous system and digestive system diseases, CHM-derived nanomaterials can be modified to adopt multiple nanostructures, which mainly modulate drug release, increase the distribution of drugs at the target organ, and improve drug transport across barriers (Table 1).52,122
Table 1 Nanostructures of natural and engineered CHMs for the treatment of various diseases
System |
Disease |
Nanostructures |
Drugs |
Effectiveness |
Ref. |
Nervous system |
PD |
Polymer nanoparticles |
Ginkgolide B |
GB-PPNPs |
Prevents neuronal cytotoxicity by promoting sustained release of ginkgolide B. |
57
|
Nanocrystals |
Resveratrol |
RES-NCs |
Improves plasma and brain drug concentrations, alleviates dopamine insufficiency, and raises dopamine and its metabolite levels. |
48
|
|
Paeoniflorin |
PA-NCs |
Increases the PA concentration in the brain through intranasal delivery of PA-NCs. |
126
|
|
Paeoniflorin |
LF-BP-PAE |
Targets PD and crosses the BBB effectively. |
127
|
AD |
Polymer nanoparticles |
Curcumin |
Cur-PLGA-NPs |
Activates the classical Wnt/β-catenin pathway and enhances the self-repair mechanism of the brain. |
58
|
|
|
Rhynchophylline |
T80-NPS-RIN |
Crosses the BBB and provides neuroprotection. |
59
|
|
Liposomes |
Curcumin |
Lipid-PEG-curcumin |
Increases affinity for amyloid deposits and improves uptake in BBB cell models. |
142
|
Spinal cord injury |
Polymer nanoparticles |
APS/TSIIA |
TSIIA@SeNPs-APS |
Elevates the antioxidant properties of APS and TSIIA. |
114
|
Stroke |
Polymer nanoparticles |
Betulinic acid |
Betulinic-acid-NPs |
Penetrates the brain barrier as an antioxidant and reduces ischemia-induced infarction. |
44
|
Brain metastases tumor |
Polymer nanoparticles |
Oleanolic acid |
Oleanolic acid nanoparticles |
Enhances the penetration of chemotherapy drugs into the brain. |
124
|
MCAO |
CDs |
Crinis Carbonisatus |
CrCi-CDs |
Lessens ischemic lesion volume and blood–brain barrier permeability, improves neurological deficits, and diminishes TNF-α and IL-6 levels. |
33
|
Glioma |
Liposomes |
Ginsenoside Rg3 |
Rg3-PTX-LPs |
Increases CD8+ T cell population, increases the M1/M2 ratio, and decreases regulatory T cells and myeloid-derived suppressor cells. |
85
|
Digestive system |
Liver cancer |
Polymer micelles |
Celastrol |
Cela/GCTR PMs |
Improves celastrol bioavailability and has significant HCC targeting activity. |
101
|
|
|
Gambogic acid |
GA-LWMH-PMs |
Improves the solubility of gambogic acid and liver targeting. |
161
|
|
|
Vinegar-baked Radix Bupleuri |
VBRB and HCPT-PMs |
Enhances the liver-targeting efficiency of HCPT-PMs after oral administration of VBRB. |
103
|
|
Liposomes |
Paclitaxel |
PTX-loaded TCR PMs |
Increases PTX intestinal absorption and oral bioavailability. |
93
|
|
|
Curcumin |
CDDP/CUR-LPs |
Prolongs drug retention time, improves the anti-tumor effect, and reduces side effects. |
163
|
Liver fibrosis |
Polymer micelles |
Resveratrol |
CRGD-PMK-MCs |
Alleviates inflammation in fibrotic mice, prevents fibrosis, and protects hepatocytes from injury. |
100
|
|
|
Berberine |
BBR-CTA-Mic |
Improves metabolic profiles and reduces the formation of aortic arch plaques in mice fed with a high-fat diet. |
162
|
IBD |
Liposomes |
Patchouli alcohol |
LF-lipo |
Reduces inflammatory cytokines and ROS levels while suppressing the MAPK/NF-κB pathway and the formation of the NLRP3 inflammasome. |
76
|
|
|
Berberine/baicalin |
BA–BBR NPs |
Treats IBD through regulating three significant components of the microbiota-gut-brain. |
37
|
Gastric carcinoma |
Liposomes |
Aloe emodin |
Nano-AE |
Inhibits the proliferation and remarkably increases the apoptosis of human gastric cancer cells. |
79
|
Acute gastric ulcer |
CDs |
Radix Sophorae flavescentis
|
RSFC-CDs |
Reduces NF-κB, TNF-α, and IL-6 levels for a protective impact, as well as inhibiting ethanol-induced inflammation and oxidative stress. |
35
|
Gastric ulcer |
CDs |
Glycyrrhizae Radix et Rhizoma |
GRR-CDs |
Minimizes the oxidative damage induced by alcohol to the stomach mucosa and tissues. |
34
|
Skin |
Bleeding |
Nanofibers |
—— |
Yunnan Baiyao |
Nanofibers' unique adhesion and structural capabilities may play a role in platelet aggregation. |
106
|
Wound healing |
Nanofibers |
Curcumin |
CSNM |
Boosts the early stages of wound healing by increasing the expression of CD31 and TGF-β. |
109
|
|
|
Aloin |
APP |
Stimulates granulation tissue development, collagen deposition, and epithelial tissue remodeling. |
168
|
4.1 Nanostructures of extracted CHMs for disease treatment
4.1.1 Nervous system diseases.
Pretreatment of a model of middle cerebral artery occlusion (MCAO) with the new species of Crinis Carbonisatus significantly reduced TNF-α and IL-6 levels in MCAO rats, and decreased the BBB permeability and ischemic lesion volume, which improved neurological deficits. Additionally, it inhibited the level of excitatory neurotransmitters aspartic acid and glutamate and increased 5-hydroxytryptamine.33 The active ingredient of Eucommia ulmoides Oliver, betulinic acid, is converted into nanoparticles, which effectively penetrated the brain barrier as an antioxidant and significantly reduced ischemia-induced infarction after intravenous administration.44 Rhein can self-assemble into hydrogels through noncovalent interactions, which can exert long-lasting neuroinflammation relief.43 A naturally occurring pentacyclic triterpene compound known as oleanolic acid exhibits anti-tumor properties.123 It creates nanoparticles that have the ability to efficiently enter the brain and address brain metastases caused by breast cancer. Nanoparticles containing oleanolic acid, which are loaded with PTX, could be employed alongside chemotherapy to treat breast cancer and its associated brain metastases.124
Resveratrol has neuroprotective activity against PD.125 After being orally administered to rats, RES-NCs demonstrated enhanced pharmacokinetics, resulting in increased plasma and brain concentrations compared to other formulations. This leads to an elevation in dopamine and its byproducts, which offer protection to the nervous system.48 Similarly, the pharmacokinetics of paeoniflorin (PA) nanocrystals is better than that of free PA, leading to a higher concentration of PA in the brain after intranasal delivery of PA nanocrystals.126 In addition, paeoniflorin (PAE) is loaded with brain targeting ligand lactoferrin (LF) containing black phosphorus nanosheets (BP) to obtain LF–BP–PAE. Through photothermal effects, these LF–BP–PAE particles crossed the BBB and effectively treated PD.127
4.1.2 Digestive system diseases.
CDs prepared from Sophorae flavescentis Carbonisata (RSFC-CDs) possess the capacity to lower the concentrations of NF-κB, TNF-α, IL-6, catalase, glutathione peroxidase, superoxide dismutase, glutathione, malondialdehyde, and inducible nitric oxide synthase (iNOS). Additionally, they exhibit the ability to hinder ethanol-induced inflammation and oxidative stress. Thus, they exert gastric protective effects.35Glycyrrhizae Radix et Rhizoma (GRR) is one of the 10 core CHMs in the treatment of gastroesophageal reflux disease. Modern pharmacological studies have indicated that GRR has anti-inflammatory, anti-ulcer, anti-cancer, antibacterial, antioxidant and other activities.128–130 Spherical GRR-CDs contain numerous active groups on their surfaces, which can effectively alleviate oxidative damage to gastric mucosa and tissues induced by alcohol, and also help restore the levels of superoxide dismutase, malondialdehyde, and nitric oxide in mice.34 Various components in CHMs can interact with multiple sites and pathways, potentially resulting in synergistic effects and chemical reactions.131 BA–BBR nanoparticles formed by BBR and BA can synergically treat IBD. The synergistic effects may be related to three significant components of the microbiota–gut–brain axis: immune inflammation, brain–gut peptides, and intestinal flora.37
4.1.3 Endocrine system diseases.
Owing to the different active ingredients of CHMs, the biological activities of CHM-derived CDs vary. Most of these CDs are applied to treat inflammatory diseases. Inspired by the carbonization process of CHMs, one-pot pyrolysis is used to isolate and collect a novel species of CDs from Pollen Typhae (PT-CDs). In a rat model of rhabdomyolysis (RM)-induced acute kidney injury (AKI), PT-CDs exhibited significant activity for improving BUN and CRE levels, the urine output and renal index, and histopathological morphology in rats with RM-induced AKI.132 The toxicity of aristolochic acid and the resulting acute kidney injury are reduced significantly through the formation of supramolecular self-assembly by BBR and aristolochic acid in zebrafish and mice during in vivo systemic toxicology experiments.40Phellodendri Chinensis Cortex Carbonisata-CDs significantly inhibited the renal damage and the production of related inflammatory factors induced by intraperitoneal injection of acute Deinagkistrodon acutus venom.133
4.2 Nanostructures of engineered CHMs for disease treatment
4.2.1 Nervous system diseases.
Polymer nanoparticles can facilitate drug transport across the BBB and are often used to study brain diseases.134–137 For the effective treatment of PD, biodegradable polyethylene glycol-B-polytrimethylene carbonate nanoparticles are developed to promote the sustained release of Ginkgolide B for 48 h, and to prevent neuronal cytotoxicity.57 Some studies have found that CUR-encapsulated PLGA nanoparticles (Cur-PLGA-NPs) can induce adult neurogenesis by activating the classical Wnt/β-catenin pathway, and Cur-PLGA-NPs may treat neurodegenerative diseases, such as Alzheimer's disease (AD), by enhancing the self-repair mechanism of the brain.58 Rhynchophylline is a significant tetracyclic oxindole alkaloid found in the stems of certain Uncaria species138 and has the potential to be useful in treating AD.139 However, its bioavailability is low, and it has a low concentration in brain tissue and poor water solubility.140,141 Rhynchophylline loaded mPEG–PLGA nanoparticles coated with Tween 80 (T80-NPS-RIN) can cross the BBB for brain-targeted delivery. T80-NPS-RIN also provides a potent neuroprotective effect.59 In other studies guided by the CHM matching theory, CHM active ingredient-based selenium nanoparticles are surface decorated with APS and loaded with TSIIA. This formulation improves the bioavailability of APS and TSIIA, enhances the antioxidant protection of APS and TSIIA, and is characterized by efficient delivery, high stability, and effective treatment of spinal cord injury.114
Liposomes are also used in brain applications. A lipid-PEG-CUR derivative is synthesized and characterized to target amyloid deposits in the brains of patients with AD. This derivative showed increased affinity for amyloid deposits, with a significantly improved uptake in BBB cell models.142 Rg3-PTX-LPs have a greater inhibitory effect on the proliferation of glioma cells than PTX-loaded cholesterol liposomes, and Rg3-PTX-LPs can transform M2-type tumor-associated macrophages into M1 phenotypes. Rg3-PTX-LPs can activate the immune microenvironment of glioma, increase the CD8+T cell population, promote the T cell immune response, increase the M1/M2 ratio, decrease the levels of regulatory T cells and myeloid-derived suppressor cells, and significantly prolong the median survival time of glioma mice/rats.85 Elemene liposomes can significantly accelerate the apoptosis of glioma cells, prolong the survival time, suppress metastasis and recurrence, and reverse multidrug resistance.143
4.2.2 Digestive system diseases.
Polymer micelles have great advantages for liver targeting, especially in liver cancer treatment. Celastrol is a proteasome inhibitor. It has a significant inhibitory effect on the progression of liver tumors,144 although its systemic toxicity, low solubility, and low bioavailability hinder its clinical application.145–148 A glycyrrhetinic acid-modified CMCS–thioketol–rhein conjugate, developed for the delivery of celastrol (Cela/GCTR PMs), is responsive to ROS in vitro, which improves celastrol bioavailability and HCC targeting. It can effectively inhibit tumor growth and does not damage organs.101 A naturally occurring compound called GA, found in licorice, exhibits a strong attraction towards the liver.149,150 GA has been used in a variety of delivery systems targeting HCC, including GA-modified chitosan nanoparticles, micelles, and liposomes.151–154 A biodegradable micellar system containing glycyrrhetinic acid has been developed, which serves a dual function of targeting liver cancer and releasing drugs intracellularly in response to REDOX signals.155 Carboxymethyl chitosan is a water-soluble derivative of chitosan, and plays a key role in drug delivery owing to its non-toxicity, good biocompatibility, biodegradability, and mucosal adhesion.156,157 The use of D-α-tocopherol polyethylene glycol 1000 succinate-modified carboxymethyl chitosan-rhein (TCR) polymer micelles demonstrates a notable therapeutic potential in addressing liver cancer.93 According to the theory of TCM, targeting is usually achieved by combining the active ingredient with the so-called meridian guide drug, which alters the distribution of the active ingredient in the body. VBRB is commonly used to enhance liver targeting, and its effectiveness along with those of several model drugs, such as rhein,158 oxymatrine,159 and resveratrol,160 has been demonstrated. Co-administration of VBRB with polymer micelles of HCPT can enhance liver targeting.103 Gambogic acid has poor water solubility, while heparin is hydrophilic and provides an anti-angiogenic effect. Therefore, to enhance the solubility and efficacy of gambogic acid against liver cancer, it is conjugated with low-molecular-weight heparin, resulting in the self-assembly of micelles in aqueous solution.161 A reasonably designed micelle (CTA-Mic) has been developed, which is designed with a hydrophobic core of α-tocopherol and a pegylated sulfhydryl shell for detangling on-site. It can enhance the liver-specific delivery of BBR. The metabolic profiles of mice fed a high-fat diet are significantly improved and the formation of aortic arch plaques is reduced following intervention with BBR-CTA-Mic.162 Promising results have been observed in the treatment of liver fibrosis through the use of ROS-sensitive micelles (CRGD-PMK-MCs) that target hepatic stellate cells. These micelles have been shown to reduce inflammation in fibrotic mice, prevent fibrosis, and protect hepatocytes from damage.100
Liposomes contribute to the treatment of digestive system diseases. The utilization of nanoliposomes to encapsulate aloe emodin and deliver it for transfection of the r-caspase-3 gene, along with photodynamic therapy, has demonstrated efficient inhibition of proliferation and induction of apoptosis in human gastric cancer cells.79 Cisplatin and CUR co-loaded liposomes are prepared using reversed-phase microemulsion and thin-film dispersion. Compared with the single drug, the liposomes show prolonged retention time, improve anti-tumor effect, and reduce side effects in mouse hepatoma H22 and human hepatoma HepG2 xenograft models.163 Biomimetic liposomes are utilized to transport Patchouli alcohol to macrophages for the treatment of inflammatory bowel diseases, resulting in decreased levels of inflammatory cytokines and ROS. Furthermore, it is able to enhance colon function by inhibiting the MAPK/NF-κB pathway and preventing the formation of the NLRP3 inflammasome, leading to a decrease in the activation of IL-1β.76 Rats exposed to NaAsO2 are intraperitoneally injected with quercetin liposomes, which have a certain therapeutic effect in the treatment of NaAsO2-induced damage to liver cells and nerve cells in rats.164
4.2.3 Skin diseases.
Nanofibers are often used for hemostasis and wound healing because of their ability to control drug release and enhance treatment efficacy. For instance, Yunnan Baiyao nanofibers have special adhesive and structural properties, associated with platelet aggregation, thereby causing blood clotting and wound closure.106 The significant effects of CUR include its ability to reduce inflammation and oxidative stress, as well as its promotion of wound healing.165,166 CSNM, fabricated by sequential electrospinning, can stop bleeding and accelerate wound healing with antibacterial effects. By promoting the early expression of CD31 and TGF-β, CSNM can hasten the process of wound healing.109 Electrospun polyvinyl alcohol and chitosan nanofiber scaffolds loaded with flaxseed extract can accelerate wound healing, with 48% of the scratch area closed within 100 h.167 A novel nanofiber membrane called Aloin-PVP/Aloin-PVP-PLA/PLA, has been developed for the purpose of achieving biphasic drug release on a timed basis. This technology shows promise for applications in promoting wound healing, providing antibacterial effects, and facilitating hemostasis. The utilization of APP nanofibers in a dorsal skin defect model of mice resulted in notable increases in collagen deposition, growth of granulation tissue, and remodeling of epithelial tissue.168 To create nanofiber felt made of CS-PVA-ANE that can enhance the healing of diabetic wounds, various concentrations of anemoside B4 (ANE) are introduced into the electrospinning solution. The resulting nanofiber felt is found to effectively increase the pace of wound healing, expedite angiogenesis, and facilitate the processes of re-epithelialization and collagen matrix deposition.107
5. Conclusions and perspectives
CHMs, as supplemental or alternative medicines, have been widely applied for treatment of a variety of diseases in Asian countries, although the material basis of CHMs remains unclear. Nonetheless, we are entering an exciting time in which the ancient wisdom of CHM complex interactions can be applied and interpreted,12 which in turn stimulates further development and application of CHMs. Nanotechnology has been used as a reliable tool in CHM research. Natural nanostructures in CHM decoctions generated during the boiling process provide a better understanding of CHM therapies,18 which may be a promising way to illustrate the material basis of CHMs. To formulate nanostructures for CHM therapies, we summarize the future opportunities and major challenges from our own perspective of the field.
First, there are several different approaches to elucidate the material basis of CHMs. From a chemical point of view, for instance, increasing efficacy and reducing toxicity is relevant to the compatibility theory of CHMs, which dictates the material basis of CHMs.169 Combining Astragali radix and Angelicae sinensis radix as the main constituents of Danggui Buxue decoction is a manifestation of the compatibility theory of CHMs. Specifically, ferulic acid (active ingredient of Angelicae sinensis radix) has been shown to increase the solubility of astragaloside IV, calycosin, and formononetin (active ingredients of Astragali radix), which improves the therapeutic effect of the decoction.170 The herb pair of Aconitum carmichaelii Debx and Rhizoma Glycyrrhizae is frequently used in clinical practice because the carboxyl groups of GA can react with multiple toxic alkaloids of Aconitum carmichaelii Debx to form insoluble complexes, which reduces the toxicity and enhances the treatment efficacy.171 Moreover, proanthocyanidins can decrease toxicity and accelerate the elimination of arsenic.172 The Jun–Chen–Zuo–Shi (emperor-minister-assistant-courier) theory of Chinese herbal formulae can be interpreted from a pharmacology and mechanism standpoint.173,174 For example, in the Realgar-Indigo naturalis formula, whose major active constituents are tetraarsenic tetrasulfide, indirubin, and TSIIA, tetraarsenic tetrasulfide is the Jun element, which targets promyelocytic leukemia (PML)-retinoic acid receptor alpha (RARalpha) oncoprotein, and adjuvants indirubin and TSIIA act as Chen and Zuo, respectively.175 However, there are still several phenomena that cannot be explained through these methods. For example, in the Gegen Qinlian decoction, amphipathic GA and insoluble components form the micellar phase to increase solubility,17 while the nanophase of Chinese herbal formulae is the key component that provides treatment efficacy.21,23 Thus, studying CHM therapies with a focus on nanostructures would be a prospective strategy.
Second, nanostructures existing in or derived from CHMs exhibit specific activities toward particular diseases, and function as the active ingredient and/or a drug carrier. CHM- or CHM-related nanomaterials have been used for treating diseases attacking the nervous system, digestive system, endocrine system, and skin. Notably, the nanostructure determines the role of the CHMs in treating these diseases.173,176–179 For instance, polymer nanoparticles, liposomes, and nanocrystals incorporating CHMs can be designed to cross the BBB.44,48,58 In contrast, benefiting from their capacity for co-delivery and targeting owing to their unique lipid bilayers, amphiphilic polymer micelles180 and liposomes181 incorporating CHMs are more extensively used in anti-tumor therapy.3,85,94,101 CDs and nanofibers based on CHMs have strong anti-inflammatory effects and promote wound healing.35,106,167 Therefore, because CHMs have a lower toxicity and a stronger synergistic effect than many synthetic drugs and, furthermore, operate under the premise of “multi-component, multi-target”, oral formulations of CHMs can form the basis of a prospective method for new drug development.122 Moreover, the application of nanotechnology to CHMs has mitigated compound limitations, such as low water solubility, short half-life, and poor bioavailability, enabling the rapid development of CHMs. Accordingly, the FDA has approved several CHM nanotherapeutics, including Abraxane® (PTX, albumin based nanoparticle) for breast cancer, Genexol-PM® (PTX, lyophilized polymer micelles) for breast cancer and non-small cell lung cancer, and Marqibo® (vincristine sulfate, liposome) for Philadelphia chromosome-negative acute lymphoblastic leukemia.182
Third, there are several limitations that need to be noted. Multiple factors influence CHM nanomedicine delivery in vivo, and investigating formulations for oral administration, the most convenient drug delivery route, can provide information about the processes of CHM nanomedicine delivery in vivo.183 Beyond that, CHMs or Chinese herbal formulae are a relatively complex chemical system, with diverse and complex pharmacological effects, which need to be explored comprehensively and systematically to identify other impacts of CHM nanomedicine delivery in vivo.184 Accordingly, the development of CHM nanomedicines should focus on small-molecule compounds, not Chinese herbal formulae.89
Overall, a summary of the nanostructures of CHMs and an analysis of the relationship between these nanostructures and disease treatment can provide evidence for identifying the potential applications of CHM therapeutic effects. Nanostructures existing in Chinese herbal formulae may contain both active ingredients and delivery frameworks to achieve targeted delivery of active ingredients. This advantage is expected to provide new impetus for the development of CHM nanomedicines to prevent and treat diseases.
Conflicts of interest
There are no conflicts to declare.
Acknowledgements
This work was supported by the National Key Research & Development Program of China (grant No. 2021YFA1201000 and 2018YFE0117800), the National Natural Science Foundation of China (NSFC) key project (grant No. 32030060), the NSFC international collaboration key project (grant No. 51861135103), and the NFSC Regional Innovation and Development Joint Fund Project (grant No. U21A20414). The authors also appreciate the support from “the Beijing-Tianjin-Hebei Basic Research Cooperation Project” (19JCZDJC64100) and the Science Fund for Creative Research Groups of Nature Science Foundation of Hebei Province (B2021201038).
References
- J.-L. Tang, B.-Y. Liu and K.-W. Ma, The Lancet, 2008, 372, 1938–1940 CrossRef PubMed
.
- W. Lam, S. Bussom, F. Guan, Z. Jiang, W. Zhang, E. A. Gullen, S.-H. Liu and Y.-C. Cheng, Sci. Transl. Med., 2010, 2, 45ra59 Search PubMed
.
- C. Hong, D. Wang, J. Liang, Y. Guo, Y. Zhu, J. Xia, J. Qin, H. Zhan and J. Wang, Theranostics, 2019, 9, 4437 CrossRef CAS PubMed
.
- S. Li, Z. Wu and W. Le, Alzheimer's Dementia, 2021, 17, 1066–1071 CrossRef PubMed
.
- D. Cyranoski, Nature, 2018, 561, 448–450 CrossRef CAS PubMed
.
- E. Y. Enioutina, E. R. Salis, K. M. Job, M. I. Gubarev, L. V. Krepkova and C. M. Sherwin, Expert Rev. Clin. Pharmacol., 2017, 10, 327–338 CAS
.
- H. T. Phu, D. T. Thuan, T. H. Nguyen, A. M. Posadino, A. H. Eid and G. Pintus, Curr. Vasc. Pharmacol., 2020, 18, 369–393 CrossRef CAS PubMed
.
- Z. Wang, X. Liu, R. L. K. Y. Ho, C. W. K. Lam and M. S. S. Chow, The Road from Nanomedicine to Precision Medicine, 2020, 931–956 CAS
.
- W. Wan-Ying, H. Jin-Jun, L. Hua-Li, Y. Wen-Zhi, J. Liang and G. De-An, Chin. J. Nat. Med., 2014, 12, 241–250 CrossRef PubMed
.
- S. Li, Science, 2015, 350, S72–S74 CrossRef PubMed
.
- Y. Tu, Nat. Med., 2011, 17, 1217–1220 CrossRef CAS PubMed
.
- F.-S. Li and J.-K. Weng, Nat. Plants, 2017, 3, 1–7 Search PubMed
.
- Y. Liu and N. Feng, Adv. Colloid Interface Sci., 2015, 221, 60–76 CrossRef CAS PubMed
.
- Y. Han, H. Sun, A. Zhang, G. Yan and X.-J. Wang, Pharmacol. Ther., 2020, 216, 107680 CrossRef CAS PubMed
.
- I. Severcan, C. Geary, E. Verzemnieks, A. Chworos and L. Jaeger, Nano Lett., 2009, 9, 1270–1277 CrossRef CAS PubMed
.
- J. A. Berrocal, G. H. Heideman, B. F. De Waal, E. Meijer and B. L. Feringa, ACS Nano, 2020, 14, 13865–13875 CrossRef CAS PubMed
.
- D. Lin, Q. Du, H. Wang, G. Gao, J. Zhou, L. Ke, T. Chen, C. Shaw and P. Rao, BioMed Res. Int., 2017, 2017, 9217912 Search PubMed
.
- X. Yang, C. Ma, Z. Chen, J. Liu, F. Liu, R. Xie, H. Zhao, G. Deng, A. T. Chen and N. Gong, Nano Res., 2019, 12, 2468 CrossRef CAS PubMed
.
- F. Shi, G. Yang, J. Ren, T. Guo, Y. Du and N. Feng, Int. J. Nanomed., 2013, 2533–2541 CrossRef PubMed
.
- Y. Zhao, Y. Zhang, H. Kong, M. Zhang, J. Cheng, J. Wu, H. Qu and Y. Zhao, Int. J. Nanomed., 2020, 9049–9059 CrossRef CAS PubMed
.
- S. W. Lu, H. Su, S. Sun, Y. Y. Guo, T. Liu, Y. Ping and Y. J. Li, Sci. Rep., 2018, 8, 12209 CrossRef PubMed
.
- Z. Zhou, X. H. Li, J. X. Liu, L. Dong, Q. Chen, J. L. Liu, H. H. Kong, Q. Y. Zhang, X. Qi, D. X. Hou, L. Zhang, G. Q. Zhang, Y. C. Liu, Y. J. Zhang, J. Li, J. Wang, X. Chen, H. Wang, J. F. Zhang, H. L. Chen, K. Zen and C. Y. Zhang, Cell Res., 2015, 25, 39–49 CrossRef CAS PubMed
.
- J. W. Zhou, G. Z. Gao, Q. P. Chu, H. Q. Wang, P. F. Rao and L. J. Ke, J. Ethnopharmacol., 2014, 151, 1116–1123 CrossRef CAS PubMed
.
- H. Sheridan, B. Kopp, L. Krenn, D. Guo and J. Sendker, Science, 2015, 350, S64–S66 CrossRef PubMed
.
- G. Wang, C. Yang, K. Zhang, J. Hu and W. Pang, Molecules, 2015, 20, 12376–12388 CrossRef CAS PubMed
.
- L. Ke, J. Zhou, W. Lu, G. Gao and P. Rao, Trends Food Sci. Technol., 2011, 22, 492–497 CrossRef CAS
.
- Y. Zhuang, J. Yan, W. Zhu, L. Chen, D. Liang and X. Xu, J. Ethnopharmacol., 2008, 117, 378–384 CrossRef PubMed
.
- J. Hu, Z. S. Wu, J. J. Yan, W. S. Pang, D. H. Liang and X. J. Xu, J. Ethnopharmacol., 2009, 123, 267–274 CrossRef PubMed
.
- X. M. Huang, X. J. Liu, X. Y. Lin, Z. H. Yuan, Y. Z. Zhang, Z. J. Wang, W. M. Pi, H. Q. Zhao, H. M. Lei and P. L. Wang, J. Nanobiotechnol., 2022, 20, 527 CrossRef CAS PubMed
.
- J. Luo, J. Hu, M. L. Zhang, Y. Zhang, J. S. Wu, J. J. Cheng, H. H. Qu, H. Kong and Y. Zhao, Nanomedicine, 2021, 16, 1657–1671 CrossRef CAS PubMed
.
- X. K. Wang, Y. Zhang, H. Kong, J. J. Cheng, M. L. Zhang, Z. W. Sun, S. N. Wang, J. X. Liu, H. H. Qu and Y. Zhao, Artif. Cells, Nanomed., Biotechnol., 2020, 48, 68–76 CrossRef PubMed
.
- H. Kong, Y. S. Zhao, Y. F. Zhu, W. Xiong, J. Luo, J. J. Cheng, Y. Zhang, M. L. Zhang, H. H. Qu and Y. Zhao, Artif. Cells, Nanomed., Biotechnol., 2021, 49, 11–19 CrossRef CAS PubMed
.
- Y. Zhang, S. Wang, F. Lu, M. Zhang, H. Kong, J. Cheng, J. Luo, Y. Zhao and H. Qu, J. Nanobiotechnol., 2021, 19, 257 CrossRef CAS PubMed
.
- Y. H. Liu, M. L. Zhang, J. J. Cheng, Y. Zhang, H. Kong, Y. Zhao and H. H. Qu, Molecules, 2021, 26, 1512 CrossRef CAS PubMed
.
- J. Hu, J. Luo, M. Zhang, J. Wu, Y. Zhang, H. Kong, H. Qu, G. Cheng and Y. Zhao, Int. J. Nanomed., 2021, 16, 2461–2475 CrossRef PubMed
.
- M. Zhang, J. Cheng, J. Hu, J. Luo, Y. Zhang, F. Lu, H. Kong, H. Qu and Y. Zhao, J. Nanobiotechnol., 2021, 19, 1–14 CrossRef CAS PubMed
.
- L. Li, H. Cui, T. Li, J. Qi, H. Chen, F. Gao, X. Tian, Y. Mu, R. He, S. Lv, F. Chu, B. Xu, P. Wang, H. Lei, H. Xu and C. Wang, Front. Pharmacol., 2020, 11, 1210 CrossRef PubMed
.
- T. Li, P. L. Wang, W. B. Guo, X. M. Huang, X. H. Tian, G. R. Wu, B. Xu, F. F. Li, C. Yan, X. J. Liang and H. M. Lei, ACS Nano, 2019, 13, 6770–6781 CrossRef CAS PubMed
.
- X. Lin, X. Huang, X. Tian, Z. Yuan, J. Lu, X. Nie, P. Wang, H. Lei and P. Wang, ACS Omega, 2022, 7, 43510–43521 CrossRef CAS PubMed
.
- P. Wang, W. Guo, G. Huang, J. Zhen, Y. Li, T. Li, L. Zhao, K. Yuan, X. Tian, X. Huang, Y. Feng, H. Lei and A. Xu, ACS Appl. Mater. Interfaces, 2021, 13, 32729–32742 CrossRef CAS PubMed
.
- C. Zhong, C. Chen, X. Gao, C. Tan, H. Bai and K. Ning, Plant Biotechnol. J., 2022, 20, 1874–1887 CrossRef CAS PubMed
.
- A. Saha, J. Adamcik, S. Bolisetty, S. Handschin and R. Mezzenga, Angew. Chem., Int. Ed., 2015, 54, 5408–5412 CrossRef CAS PubMed
.
- J. Zheng, R. Fan, H. Q. Wu, H. H. Yao, Y. J. Yan, J. M. Liu, L. Ran, Z. F. Sun, L. Z. Yi, L. Dang, P. P. Gan, P. Zheng, T. L. Yang, Y. Zhang, T. Tang and Y. Wang, Nat. Commun., 2019, 10, 1604 CrossRef CAS PubMed
.
- G. Deng, C. Ma, H. Zhao, S. Zhang, J. Liu, F. Liu, Z. Chen, A. T. Chen, X. Yang, J. Avery, P. Zou, F. Du, K. P. Lim, D. Holden, S. Li, R. E. Carson, Y. Huang, Q. Chen, W. T. Kimberly, J. M. Simard, K. N. Sheth and J. Zhou, Theranostics, 2019, 9, 6991–7002 CrossRef CAS PubMed
.
- J. Pi, S. Wang, W. Li, D. Kebebe, Y. Zhang, B. Zhang, D. Qi, P. Guo, N. Li and Z. Liu, Asian J. Pharm. Sci., 2019, 14, 154–164 CrossRef PubMed
.
- Z. Li, Y. Liu, J. Wang, X. Feng, E. O. Nwafor, Y. Zhang, R. Liu, W. Dang, Q. Zhang, C. Yu, J. Pi and Z. Liu, Drug Delivery Transl. Res., 2022, 12, 3017–3028 CrossRef CAS PubMed
.
- H. G. Sheng, Y. N. Zhang, J. J. Nai, S. H. Wang, M. M. Dai, G. T. Lin, L. Q. Zhu and Q. Zhang, Pharm. Biol., 2020, 58, 518–527 CrossRef CAS PubMed
.
- S. Xiong, W. Liu, Y. L. Zhou, Y. S. Mo, Y. Liu, X. J. Chen, H. F. Pan, D. S. Yuan, Q. Wang and T. K. Chen, Asian J. Pharm. Sci., 2020, 15, 518–528 CrossRef PubMed
.
- Y. Ma, Y. Yang, J. Xie, J. Xu, P. Yue and M. Yang, Int. J. Nanomed., 2018, 13, 3763–3779 CrossRef CAS PubMed
.
- J. Shi-Ying, H. Jin, J. Shi-Xiao, L. Qing-Yuan, B. Jin-Xia, H. G. Chen, L. Rui-Sheng, W. Wei and Y. Hai-Long, Chin. J. Nat. Med., 2014, 12, 71–80 Search PubMed
.
- L. Tu, Y. Yi, W. Wu, F. Hu, K. Hu and J. Feng, Int. J. Pharm., 2013, 458, 135–140 CrossRef CAS PubMed
.
- J. Zhang, K. Hu, L. Di, P. Wang, Z. Liu, J. Zhang, P. Yue, W. Song, J. Zhang and T. Chen, Adv. Drug Delivery Rev., 2021, 178, 113964 CrossRef CAS PubMed
.
- M. J. Mitchell, M. M. Billingsley, R. M. Haley, M. E. Wechsler, N. A. Peppas and R. Langer, Nat. Rev. Drug Discovery, 2021, 20, 101–124 CrossRef CAS PubMed
.
- L. Feng, C. Zhu, H. Yuan, L. Liu, F. Lv and S. Wang, Chem. Soc. Rev., 2013, 42, 6620–6633 RSC
.
- S. E. Birk, A. Boisen and L. H. Nielsen, Adv. Drug Delivery Rev., 2021, 174, 30–52 CrossRef CAS PubMed
.
- J. W. Hickey, J. L. Santos, J. M. Williford and H. Q. Mao, J. Controlled Release, 2015, 219, 536–547 CrossRef CAS PubMed
.
- Q. Wang, R. Ma, P. Liu, G. Cheng, Q. Yang, X. Chen, Z. Wu, D. Yuan and T. Chen, Pharmaceutics, 2022, 14, 1731 CrossRef CAS PubMed
.
- S. K. Tiwari, S. Agarwal, B. Seth, A. Yadav, S. Nair, P. Bhatnagar, M. Karmakar, M. Kumari, L. K. Chauhan, D. K. Patel, V. Srivastava, D. Singh, S. K. Gupta, A. Tripathi, R. K. Chaturvedi and K. C. Gupta, ACS Nano, 2014, 8, 76–103 CrossRef CAS PubMed
.
- R. Xu, J. Wang, J. Xu, X. Song, H. Huang, Y. Feng and C. Fu, Int. J. Nanomed., 2020, 15, 1149–1160 CrossRef CAS PubMed
.
- T. Wu, X. Hou, J. Li, H. Ruan, L. Pei, T. Guo, Z. Wang, T. Ci, S. Ruan, Y. He, Z. He, N. Feng and Y. Zhang, ACS Nano, 2021, 15, 20087–20104 CrossRef CAS PubMed
.
- Y. Geng, J. Xiang, S. Shao, J. Tang and Y. Shen, J. Controlled Release, 2022, 342, 122–133 CrossRef CAS PubMed
.
- J. Feng, Y. Wang, Y. Lv, S. Fang, M. Ren, M. Yao, M. Lan, Y. Zhao and F. Gao, Mol. Pharm., 2022, 19, 4157–4170 CrossRef CAS PubMed
.
- L. Li, Y. Wang, R. Guo, S. Li, J. Ni, S. Gao, X. Gao, J. Mao, Y. Zhu, P. Wu, H. Wang, D. Kong, H. Zhang, M. Zhu and G. Fan, J. Controlled Release, 2020, 317, 259–272 CrossRef CAS PubMed
.
- S. W. Xu, Z. A. Feng, Y. Zhang, H. Y. Ni, Z. G. Liu and D. Y. Wang, Int. J. Biol. Macromol., 2022, 222, 1936–1947 CrossRef CAS PubMed
.
- P. Gu, G. Cai, Y. Yang, Y. Hu, J. Liu and D. Wang, Int. J. Biol. Macromol., 2022, 207, 559–569 CrossRef CAS PubMed
.
- P. F. Gu, A. Wusiman, S. Y. Wang, Y. Zhang, Z. G. Liu, Y. L. Hu, J. G. Liu and D. Y. Wang, Carbohydr. Polym., 2019, 223, 115128 CrossRef CAS PubMed
.
- Y. Zhang, L. A. Jiao, Z. Y. Wu, P. F. Gu, Z. Feng, S. W. Xu, Z. G. Liu, Y. Yang and D. Y. Wang, Int. J. Biol. Macromol., 2022, 209, 513–524 CrossRef CAS PubMed
.
- Y. Zhang, P. F. Gu, A. Wusiman, S. W. Xu, H. Y. Ni, T. X. Qiu, Z. G. Liu, Y. L. Hu, J. G. Liu and D. Y. Wang, Int. J. Nanomed., 2020, 15, 5527–5543 CrossRef CAS PubMed
.
- Z. Feng, S. Peng, Z. Y. Wu, L. A. Jiao, S. W. Xu, Y. Wu, Z. G. Liu, Y. L. Hu, J. G. Liu, Y. Wu and D. Y. Wang, Int. J. Biol. Macromol., 2021, 182, 2024–2036 CrossRef CAS PubMed
.
- K. Xiong, Y. Zhang, Q. Wen, J. Luo, Y. Lu, Z. Wu, B. Wang, Y. Chen, L. Zhao and S. Fu, Int. J. Pharm., 2020, 589, 119875 CrossRef CAS PubMed
.
- Y. Guo, S. Liu, F. Luo, D. Tang, T. Yang, X. Yang and Y. Xie, Pharmaceutics, 2022, 14, 422 CrossRef CAS PubMed
.
- F. Liu, L. Li, M. Lan, T. Zou, Z. Kong, T. Cai, X. Wu and Y. Cai, Nanomedicine, 2021, 16, 2411–2430 CrossRef CAS PubMed
.
- B. Y. Ding, M. A. Wahid, Z. J. Wang, C. Xie, A. Thakkar, S. Prabhu and J. Wang, Nanoscale, 2017, 9, 11739–11753 RSC
.
- M. Zhou, J. Q. Dong, J. Q. Huang, W. Ye, Z. S. Zheng, K. B. Huang, Y. H. Pan, J. J. Cen, Y. P. Liang, G. N. Shu, S. Ye, X. X. Lu and J. X. Zhang, Adv. Sci., 2022, 9, e2105077 CrossRef PubMed
.
- H. Y. Que, W. Q. Hong, T. X. Lan, H. Zeng, L. Chen, D. D. Wan, Z. F. Bi, W. Y. Ren, M. Luo, J. Y. Yang, C. He, A. L. Zhong and X. W. Wei, Signal Transduction Targeted Ther., 2022, 7, 399 CrossRef CAS PubMed
.
- Y. Zhao, Y. Yang, J. Zhang, R. Wang, B. Cheng, D. Kalambhe, Y. Wang, Z. Gu, D. Chen, B. Wang and Y. Huang, Acta Pharm. Sin. B, 2020, 10, 1966–1976 CrossRef CAS PubMed
.
- Q. Wang, C. Wei, W. Weng, R. Bao, M. Adu-Frimpong, E. Toreniyazov, H. Ji, X. M. Xu and J. Yu, Int. J. Pharm., 2021, 592, 120036 CrossRef CAS PubMed
.
- R. Rezaei-Sadabady, A. Eidi, N. Zarghami and A. Barzegar, Artif. Cells, Nanomed., Biotechnol., 2016, 44, 128–134 CrossRef CAS PubMed
.
- K. T. Li, Q. Q. Duan, Q. Chen, J. W. He, S. Tian, H. D. Lin, Q. Gao and D. Q. Bai, Cancer Med., 2016, 5, 361–369 CrossRef CAS PubMed
.
- D. A. Smith, K. Beaumont, T. S. Maurer and L. Di, J. Med. Chem., 2019, 62, 2245–2255 CrossRef CAS PubMed
.
- Q. Yang, J. Yang, S. Sun, J. Zhao, S. Liang, Y. Feng, M. Liu and J. Zhang, Int. J. Nanomed., 2022, 17, 3633–3653 CrossRef PubMed
.
- Y. Wang, L. Cao, L. M. Xu, F. F. Cao, B. Peng, X. Zhang, Y. F. Shen, G. Uzan and D. H. Zhang, J. Neuroimmune Pharmacol., 2015, 10, 506–516 CrossRef PubMed
.
- L. Xi, Z. Lin, F. Qiu, S. Chen, P. Li, X. Chen, Z. Wang and Y. Zheng, Acta Pharm. Sin. B, 2022, 12, 339–352 CrossRef CAS PubMed
.
- X. Zhang, H. Chen, Y. Zhang, Q. Huang, J. Feng, H. Xing, X. Fu, X. Yan, Y. Zhang, Q. Xu and J. Liang, Int. J. Nanomed., 2022, 17, 5137–5151 CrossRef CAS PubMed
.
- Y. Zhu, J. Liang, C. Gao, A. Wang, J. Xia, C. Hong, Z. Zhong, Z. Zuo, J. Kim, H. Ren, S. Li, Q. Wang, F. Zhang and J. Wang, J. Controlled Release, 2021, 330, 641–657 CrossRef CAS PubMed
.
- J. Xia, S. Ma, X. Zhu, C. Chen, R. Zhang, Z. Cao, X. Chen, L. Zhang, Y. Zhu, S. Zhang, S. Li, G. Gu, X. Wei, K. Yu and J. Wang, Sci. Adv., 2022, 8, eabj1262 CrossRef CAS PubMed
.
- S. Barui, S. Saha, G. Mondal, S. Haseena and A. Chaudhuri, Biomaterials, 2014, 35, 1643–1656 CrossRef CAS PubMed
.
- L. Zhang, S. Zhang, M. Jiang, L. Lu, Y. Ding, N. Ma, Y. Zhao, S. Xuchen and N. Zhang, Int. J. Nanomed., 2021, 16, 5531–5550 CrossRef PubMed
.
- R. Sun, J. Dai, M. Ling, L. Yu, Z. Yu and L. Tang, J. Nanobiotechnol., 2022, 20, 194 CrossRef CAS PubMed
.
- Y. Xi, T. Jiang, Y. Yu, J. Yu, M. Xue, N. Xu, J. Wen, W. Wang, H. He, Y. Shen, D. Chen, X. Ye and T. J. Webster, Int. J. Nanomed., 2019, 14, 6425–6437 CrossRef CAS PubMed
.
- M. Zhang, T. Dai and N. Feng, Nanoscale Res. Lett., 2017, 12, 274 CrossRef PubMed
.
- C. Zhu, S. Gong, J. Ding, M. Yu, E. Ahmad, Y. Feng and Y. Gan, Acta Pharm. Sin. B, 2019, 9, 107–117 CrossRef PubMed
.
- X. Wang, Y. Zheng, L. Qiu, H. Ouyang, X. Xu, W. Xu, Y. Zhang and W. Xu, Int. J. Pharm., 2022, 625, 122138 CrossRef CAS PubMed
.
- T. E. Chen, L. X. Tu, G. Wang, N. Qi, W. Wu, W. Zhang and J. F. Feng, Int. J. Pharmaceut., 2020, 578, 119105 CrossRef CAS PubMed
.
- X. Wang, Y. Chen, F. Z. Dahmani, L. Yin, J. Zhou and J. Yao, Biomaterials, 2014, 35, 7654–7665 CrossRef CAS PubMed
.
- X. Wang, L. Qiu, X. Wang, H. Ouyang, T. Li, L. Han, X. Zhang, W. Xu and K. Chu, Int. J. Pharm., 2020, 573, 118840 CrossRef CAS PubMed
.
- X. Wang, Y. Guo, L. Qiu, X. Wang, T. Li, L. Han, H. Ouyang, W. Xu and K. Chu, Carbohydr. Polym., 2019, 206, 121–131 CrossRef CAS PubMed
.
- G. Li, Y. Lu, Y. Fan, Q. Ning and W. Li, Drug Delivery, 2020, 27, 1010–1017 CrossRef CAS PubMed
.
- P. Ding, H. Shen, J. Wang and J. Ju, Artif. Cells, Nanomed., Biotechnol., 2018, 46, 668–674 CrossRef CAS PubMed
.
- Y. Hao, K. Song, X. Tan, L. Ren, X. Guo, C. Zhou, H. Li, J. Wen, Y. Meng, M. Lin, Y. Zhang, H. Huang, L. Wang and W. Zheng, ACS Nano, 2022, 16, 20739–20757 CrossRef CAS PubMed
.
- X. Zhang, X. Xu, X. Wang, Y. Lin, Y. Zheng, W. Xu, J. Liu and W. Xu, Carbohydr. Polym., 2023, 303, 120439 CrossRef CAS PubMed
.
- C. Shen, L. Zhao, X. Du, J. Tian, Y. Yuan, M. Jia, Y. He, R. Zeng, R. Qiao and C. Li, Mol. Pharm., 2021, 18, 1419–1430 CrossRef CAS PubMed
.
- H. Wu, T. Yu, Y. Tian, Y. Wang, R. Zhao and S. Mao, Phytomedicine, 2018, 44, 1–8 CrossRef CAS PubMed
.
- X. Zheng, C. Sun, R. Yu, X. Chu, J. Xu, C. Liu, M. Zhao, X. Xu, M. Xia and C. Wang, Int. J. Pharm., 2020, 577, 119034 CrossRef CAS PubMed
.
- R. Rasouli, A. Barhoum, M. Bechelany and A. Dufresne, Macromol. Biosci., 2019, 19, e1800256 CrossRef PubMed
.
- S. C. Lenaghan, L. Xia and M. Zhang, J. Biomed. Nanotechnol., 2009, 5, 472–476 CrossRef CAS PubMed
.
- H. Zhang, M. Zhang, X. Wang, M. Zhang, X. Wang, Y. Li, Z. Cui, X. Chen, Y. Han and W. Zhao, Drug Delivery, 2022, 29, 174–185 CrossRef CAS PubMed
.
- Z. Xu, B. Deng, X. Wang, J. Yu, Z. Xu, P. Liu, C. Liu, Y. Cai, F. Wang, R. Zong, Z. Chen, H. Xing and G. Chen, J. Nanobiotechnol., 2021, 19, 404 CrossRef CAS PubMed
.
- K. Chen, H. Pan, D. X. Ji, Y. J. Li, H. L. Duan and W. S. Pan, Mater. Sci. Eng., C, 2021, 127, 112245 CrossRef CAS PubMed
.
- Y. Yang, W. Zhu, Z. Dong, Y. Chao, L. Xu, M. Chen and Z. Liu, Adv. Mater., 2017, 29, 1703588 CrossRef PubMed
.
- M. Gong, C. Chi, J. J. Ye, M. H. Liao, W. Q. Xie, C. G. Wu, R. Shi and L. Q. Zhang, Colloids Surf., B, 2018, 170, 201–209 CrossRef CAS PubMed
.
- J. Li, X. Wang, H. Jiang, X. Lu, Y. Zhu and B. Chen, Nanoscale, 2011, 3, 3115–3122 RSC
.
- H. Li, H. Wan, T. Xia, M. Chen, Y. Zhang, X. Luo and X. Li, Nanoscale, 2015, 7, 13075–13087 RSC
.
- S. Rao, Y. Lin, R. Lin, J. Liu, H. Wang, W. Hu, B. Chen and T. Chen, J. Nanobiotechnol., 2022, 20, 278 CrossRef CAS PubMed
.
- W. Deng, H. Wang, B. Wu and X. Zhang, Acta Pharm. Sin. B, 2019, 9, 74–86 CrossRef PubMed
.
- M. Cong, S. Tan, S. Li, L. Gao, L. Huang, H. G. Zhang and H. Qiao, Adv. Drug Delivery Rev., 2022, 182, 114108 CrossRef CAS PubMed
.
- J. C. Du, Z. Liang, J. T. Xu, Y. Zhao, X. Y. Li, Y. L. Zhang, D. D. Zhao, R. X. Chen, Y. Liu, T. Joshi, J. H. Chang, Z. Q. Wang, Y. X. Zhang, J. D. Zhu, Q. Liu, D. Xu and C. Y. Jiang, Sci. China: Life Sci., 2019, 62, 309–320 CrossRef CAS PubMed
.
- X. Li, Z. Liang, J. Du, Z. Wang, S. Mei, Z. Li, Y. Zhao, D. Zhao, Y. Ma and J. Ye, Sci. China Life Sci., 2019, 62, 333–348 CrossRef CAS PubMed
.
- K. Sundaram, D. P. Miller, A. Kumar, Y. Teng, M. Sayed, J. Y. Mu, C. Lei, M. K. Sriwastva, L. F. Zhang, Y. Jun, M. L. Merchant, L. Q. He, Y. Fang, S. Q. Zhang, X. Zhang, J. W. Park, R. J. Lamont and H. G. Zhang, iScience, 2019, 21, 308–327 CrossRef CAS PubMed
.
- X. Zhuang, X. Xiang, W. Grizzle, D. Sun, S. Zhang, R. C. Axtell, S. Ju, J. Mu, L. Zhang, L. Steinman, D. Miller and H. G. Zhang, Mol. Ther., 2011, 19, 1769–1779 CrossRef CAS PubMed
.
- B. Tang, W. Zeng, L. L. Song, H. M. Wang, L. Q. Qu, H. H. Lo, L. Yu, A. G. Wu, V. K. W. Wong and B. Y. K. Law, Pharmaceuticals, 2022, 15, 83 CrossRef CAS PubMed
.
- L. Qiao, M. Han, S. Gao, X. Shao, X. Wang, L. Sun, X. Fu and Q. Wei, J. Mater. Chem. B, 2020, 8, 6333–6351 RSC
.
- W. Wang, Y. Li, Y. Li, D. Sun, H. Li and L. Chen, Curr. Top. Med. Chem., 2022, 22, 3–23 CrossRef CAS PubMed
.
- Y. Bao, S. Zhang, Z. Chen, A. T. Chen, J. Ma, G. Deng, W. Xu, J. Zhou, Z. Q. Yu, G. Yao and J. Chen, Mol. Pharm., 2020, 17, 1343–1351 CrossRef CAS PubMed
.
- S. Bastianetto, C. Menard and R. Quirion, BBA, Mol. Basis Dis., 2015, 1852, 1195–1201 CrossRef CAS PubMed
.
- C. Wu, B. Li, Y. Zhang, T. Chen, C. Chen, W. Jiang, Q. Wang and T. Chen, Asian J. Pharm. Sci., 2020, 15, 326–335 CrossRef PubMed
.
- S. Xiong, Z. Li, Y. Liu, Q. Wang, J. Luo, X. Chen, Z. Xie, Y. Zhang, H. Zhang and T. Chen, Biomaterials, 2020, 260, 120339 CrossRef CAS PubMed
.
- Z. F. Wang, J. Liu, Y. A. Yang and H. L. Zhu, Curr. Med. Chem., 2020, 27, 1997–2011 CrossRef CAS PubMed
.
- M. Mukherjee, N. Bhaskaran, R. Srinath, H. N. Shivaprasad, J. J. Allan, D. Shekhar and A. Agarwal, Indian J. Exp. Biol., 2010, 48, 269–274 Search PubMed
.
- A. M. Aly, L. Al-Alousi and H. A. Salem, AAPS PharmSciTech, 2005, 6, E74–E82 CrossRef PubMed
.
- Z. Bi, Y. Zheng, J. Yuan and Z. Bian, Curr. Pharm. Des., 2017, 23, 5163–5172 CrossRef CAS PubMed
.
- X. Wang, T. Wu, Y. Yang, L. Zhou, S. Wang, J. Liu, Y. Zhao, M. Zhang, Y. Zhao, H. Qu, H. Kong and Y. Zhang, J. Nanobiotechnol., 2023, 21, 63 CrossRef CAS PubMed
.
- M. Zhang, J. Cheng, Z. Sun, H. Kong, Y. Zhang, S. Wang, X. Wang, Y. Zhao and H. Qu, Nanoscale Res. Lett., 2019, 14, 377 CrossRef CAS PubMed
.
- X. Ma, L. Kuang, Y. Yin, L. Tang, Y. Zhang, Q. Fan, B. Wang, Z. Dong, W. Wang, T. Yin and Y. Wang, ACS Nano, 2023, 17, 2341–2355 CrossRef CAS PubMed
.
- Z. Lv, Y. Cao, D. Xue, H. Zhang, S. Zhou, N. Yin, W. Li, L. Jin, Y. Wang and H. Zhang, J. Mater. Chem. B, 2023, 11, 1100–1107 RSC
.
- X. Li, Q. Cai, B. A. Wilson, H. Fan, H. Dave, M. Giannotta, R. Bachoo and Z. Qin, Nanoscale, 2023, 15, 3387–3397 RSC
.
- B. Y. Li, N. Li, L. Q. Chen, S. X. Ren, D. Gao, H. Geng, J. X. Fu, M. Zhou and C. F. Xing, ACS Appl. Mater Interfaces, 2022, 14, 48416–48425 CrossRef CAS PubMed
.
- C. Li, G. Tu, C. Luo, Y. Guo, M. Fang, C. Zhu, H. Li, J. Ou, Y. Zhou, W. Liu, K. K. L. Yung and Z. Mo, Prog. Neuropsychopharm. Biol. Psychiatry, 2018, 86, 379–389 CrossRef CAS PubMed
.
- Y. F. Xian, Z. X. Lin, M. Zhao, Q. Q. Mao, S. P. Ip and C. T. Che, Planta Med., 2011, 77, 1977–1983 CrossRef CAS PubMed
.
- C. Zhang, X. Wu, Y. Xian, L. Zhu, G. Lin and Z. X. Lin, J. Evidence-Based Complementary Altern. Med., 2019, 2019, 4016323 Search PubMed
.
- S. Imamura, M. Tabuchi, H. Kushida, A. Nishi, H. Kanno, T. Yamaguchi, K. Sekiguchi, Y. Ikarashi and Y. Kase, Cell. Mol. Neurobiol., 2011, 31, 787–793 CrossRef CAS PubMed
.
- S. Mourtas, A. N. Lazar, E. Markoutsa, C. Duyckaerts and S. G. Antimisiaris, Eur. J. Med. Chem., 2014, 80, 175–183 CrossRef CAS PubMed
.
- J. Hu and D. H. Xu, Zhongguo Zhong Xi Yi Jie He Za Zhi, 2008, 28, 637–639 Search PubMed
.
- G. Wang, Q. Xiao, Y. Wu, Y. J. Wei, Y. Jing, X. R. Cao and Z. N. Gong, J. Cell. Physiol., 2019, 234, 16431–16446 CrossRef CAS PubMed
.
- G. S. Chen, Y. L. Liu, G. L. Shi, Y. T. Luo, S. Fu, A. H. Yang, Y. Y. Zhou, Y. Q. Wu, L. F. Lin and H. Li, J. Drug Delivery Sci. Technol., 2022, 75, 103630 CrossRef CAS
.
- H. Si, H. Wang, H. Xiao, Y. Fang and Z. Wu, Cancer Manage. Res., 2021, 13, 1099–1111 CrossRef CAS PubMed
.
- Y. Chen, D. Qu, R. Fu, M. Guo, Y. Qin, J. Guo and Y. Chen, Int. J. Nanomed., 2018, 13, 7275–7287 CrossRef CAS PubMed
.
- K. Tang, Q. Huang, J. Zeng, G. Wu, J. Huang, J. Pan and W. Lu, Molecules, 2014, 19, 10177–10188 CrossRef PubMed
.
- B. Lallemand, M. Gelbcke, J. Dubois, M. Prevost, I. Jabin and R. Kiss, Mini-Rev. Med. Chem., 2011, 11, 881–887 CrossRef CAS PubMed
.
- M. Negishi, A. Irie, N. Nagata and A. Ichikawa, Biochim. Biophys. Acta, 1991, 1066, 77–82 CrossRef CAS PubMed
.
- C. Zhang, W. Wang, T. Liu, Y. Wu, H. Guo, P. Wang, Q. Tian, Y. Wang and Z. Yuan, Biomaterials, 2012, 33, 2187–2196 CrossRef CAS PubMed
.
- W. Huang, W. Wang, P. Wang, C. N. Zhang, Q. Tian, Y. Zhang, X. H. Wang, R. T. Cha, C. H. Wang and Z. Yuan, J. Mater. Sci.: Mater. Med., 2011, 22, 853–863 CrossRef CAS PubMed
.
- Q. Tian, C. N. Zhang, X. H. Wang, W. Wang, W. Huang, R. T. Cha, C. H. Wang, Z. Yuan, M. Liu, H. Y. Wan and H. Tang, Biomaterials, 2010, 31, 4748–4756 CrossRef CAS PubMed
.
- W. Huang, W. Wang, P. Wang, Q. Tian, C. Zhang, C. Wang, Z. Yuan, M. Liu, H. Wan and H. Tang, Acta Biomater., 2010, 6, 3927–3935 CrossRef CAS PubMed
.
- F. Chen, J. Zhang, Y. He, X. Fang, Y. Wang and M. Chen, Biomater. Sci., 2016, 4, 167–182 RSC
.
- Z. Shariatinia, Int. J. Biol. Macromol., 2018, 120, 1406–1419 CrossRef CAS PubMed
.
- X. Lv, W. Zhang, Y. Liu, Y. Zhao, J. Zhang and M. Hou, Carbohydr. Polym., 2018, 198, 86–93 CrossRef CAS PubMed
.
- R. Z. Zhao, D. Yuan, S. J. Liu, Y. J. Chen, L. J. Liu and Y. Zhao, J. Ethnopharmacol., 2010, 132, 421–428 CrossRef PubMed
.
-
R.-Z. Zhao, Y.-J. Chen and J.-X. Cai, IEEE International Conference on Bioinformatics & Biomedicine Workshops, 2011, https://ieeexplore.ieee.org/abstract/document/6112462 Search PubMed.
- R. Zhao, S. Liu, S. Mao and Y. Wang, J. Ethnopharmacol., 2009, 126, 415–420 CrossRef CAS PubMed
.
- X. Yan, Y. Yang, L. He, D. Peng and D. Yin, Int. J. Pharm., 2017, 522, 110–118 CrossRef CAS PubMed
.
- H. H. Guo, C. L. Feng, W. X. Zhang, Z. G. Luo, H. J. Zhang, T. T. Zhang, C. Ma, Y. Zhan, R. Li, S. Wu, Z. Abliz, C. Li, X. L. Li, X. L. Ma, L. L. Wang, W. S. Zheng, Y. X. Han and J. D. Jiang, Nat. Commun., 2019, 10, 1981 CrossRef PubMed
.
- Y. Cheng, P. Zhao, S. Wu, T. Yang, Y. Chen, X. Zhang, C. He, C. Zheng, K. Li, X. Ma and G. Xiang, Int. J. Pharm., 2018, 545, 261–273 CrossRef CAS PubMed
.
- A. Ghosh, A. K. Mandal, S. Sarkar and N. Das, Drug Delivery, 2011, 18, 451–459 CrossRef CAS PubMed
.
- C. Mohanty and S. K. Sahoo, Drug Discovery Today, 2017, 22, 1582–1592 CrossRef CAS PubMed
.
- D. Akbik, M. Ghadiri, W. Chrzanowski and R. Rohanizadeh, Life Sci., 2014, 116, 1–7 CrossRef CAS PubMed
.
- M. Doostan, M. Doostan, P. Mohammadi, K. Khoshnevisan and H. Maleki, Int. J. Biol. Macromol., 2023, 228, 506–516 CrossRef CAS PubMed
.
- W. P. Li, J. Y. Wang, Z. Q. Cheng, G. X. Yang, C. L. Zhao, F. Gao, Z. K. Zhang and Y. J. Qian, RSC Adv., 2022, 12, 27300–27308 RSC
.
- M. Zhou, Y. Hong, X. Lin, L. Shen and Y. Feng, J. Ethnopharmacol., 2017, 206, 363–375 CrossRef PubMed
.
- K. Y. Zheng, Z. X. Zhang, C. Y. Du, W. L. Zhang, C. W. Bi, R. C. Choi, T. T. Dong and K. W. Tsim, Planta Med., 2014, 80, 159–164 CrossRef CAS PubMed
.
- Y. He, Z. Wei, Y. Xie, X. Yi, Y. Zeng, Y. Li and C. Liu, J. Pharm. Anal., 2020, 10, 178–186 CrossRef PubMed
.
- M. Xu, Q. Niu, Y. Hu, G. Feng, H. Wang and S. Li, Oxid. Med. Cell. Longevity, 2019, 2019, 8549035 Search PubMed
.
- Y. Wang, Y. Zhang, C. Ding, C. Jia, H. Zhang, T. Peng, S. Cheng, W. Chen, Y. Tan, X. Wang, Z. Liu, P. Wei, X. Wang, M. Jiang and Q. Hua, Front. Oncol., 2022, 12, 790713 CrossRef PubMed
.
- Y. Zhang, Y. Wang, Z. Chen, S. Cheng, C. Ding, J. Zhang, T. Peng, W. Chen, D. Zhang, Y. Tan, X. Wang, R. Dong, M. Jiang and Q. Hua, Toxicol. Res., 2021, 10, 334–344 CrossRef PubMed
.
- L. Wang, G. B. Zhou, P. Liu, J. H. Song, Y. Liang, X. J. Yan, F. Xu, B. S. Wang, J. H. Mao, Z. X. Shen, S. J. Chen and Z. Chen, Proc. Natl. Acad. Sci. U. S. A., 2008, 105, 4826–4831 CrossRef CAS PubMed
.
- Y. Tan, X. Wang, J. Zhang, H. Zhang, H. Li, T. Peng, W. Chen, P. Wei, Z. Liu, F. He, J. Li, H. Ding, N. Li, Z. Wang, Z. Zhang and Q. Hua, Front. Pharmacol., 2022, 13, 850175 CrossRef CAS PubMed
.
- Z. Zhang, W. Cheng, Y. Pan and L. Jia, J. Mater. Chem. B, 2020, 8, 655–665 RSC
.
- X. Wang, Y. Tan, Y. Zhang, Z. Xu, B. Xu, H. Lei, C. Ding, S. Cheng, X. Wang, P. Wei, Z. Wang, Q. Mao, C. Ai and Q. Hua, Pharmacol. Res., 2020, 161, 105233 CrossRef CAS PubMed
.
- S. Du, Y. Lv, N. Li, X. Huang, X. Liu, H. Li, C. Wang and Y. F. Jia, Drug Delivery, 2020, 27, 953–963 CrossRef CAS PubMed
.
- J. Liu, C. Chen, T. Wei, O. Gayet, C. Loncle, L. Borge, N. Dusetti, X. Ma, D. Marson and E. Laurini, Exploration, 2021, 1, 21–34 CrossRef
.
- D. Jana and Y. Zhao, Strategies for enhancing cancer chemodynamic therapy performance, Exploration, 2022, 2, 20210238 CrossRef
.
- V. Gadekar, Y. Borade, S. Kannaujia, K. Rajpoot, N. Anup, V. Tambe, K. Kalia and R. K. Tekade, J. Controlled Release, 2021, 330, 372–397 CrossRef CAS PubMed
.
- S. Ahadian, J. A. Finbloom, M. Mofidfar, S. E. Diltemiz, F. Nasrollahi, E. Davoodi, V. Hosseini, I. Mylonaki, S. Sangabathuni, H. Montazerian, K. Fetah, R. Nasiri, M. R. Dokmeci, M. M. Stevens, T. A. Desai and A. Khademhosseini, Adv. Drug Delivery Rev., 2020, 157, 37–62 CrossRef CAS PubMed
.
- W. Zhou, X. Cheng and Y. Zhang, Pharmacol. Ther., 2016, 162, 170–178 CrossRef CAS PubMed
.
Footnote |
† These authors contributed equally to this manuscript. |
|
This journal is © The Royal Society of Chemistry 2023 |
Click here to see how this site uses Cookies. View our privacy policy here.