DOI:
10.1039/D0RA01263G
(Paper)
RSC Adv., 2020,
10, 11755-11765
Mixed Tb/Dy coordination ladders based on tetra(carboxymethyl)thiacalix[4]arene: a new avenue towards luminescent molecular nanomagnets†
Received
9th February 2020
, Accepted 2nd March 2020
First published on 24th March 2020
Abstract
The macrocyclic ligand calix[4]arene (L1) and its sulphur-containing analogue thia[4]calixarene (L2) are promising precursors for functional molecular materials as they offer rational functionalization with various organic groups. Here, we present the first example of lanthanide-based coordination polymers built from the macrocyclic thiacalix[4]arene backbone bearing four carboxylic moieties, namely, ligand H4L3. The combination of H4L3 with the Tb3+ and Dy3+ cations led to the formation of 1D ladder-type coordination polymers with the formula [LnIIIHL3DMF3]·(DMF) (where DMF = dimethylformamide and Ln = Tb or Dy, denoted as HL3–Tb and HL3–Dy), which resulted from the coordination of the lanthanide cations with the partially deprotonated ligand HL33− that behaved as a T-shape connector. The coordination sphere around the metal was completed by the coordinated DMF solvent molecules. By combining both Tb3+ and Dy3+ cations, isostructural heterobimetallic solid solutions HL3–Tb1−xDyx were also prepared. HL3–Tb and HL3–Dy showed visible light photoluminescence originating from the f–f electronic transitions of pale green emissive Tb3+ and pale yellow emissive Dy3+ with efficient sensitization by the functionalized thia[4]calixarene ligand HL3. In the HL3–Tb1−xDyx solid solutions, the Tb/Dy ratio governed both the emission colour as well as the emission quantum yield, which reached even 28% at room temperature for HL3–Tb. Moreover, HL3–Dy exhibited a slow magnetic relaxation effect related to the magnetic anisotropy of the dodecahedral Dy3+ complexes, which were well isolated in the crystal lattice by expanded organic spacers.
Introduction
Coordination polymers (CPs),1,2 MOFs3 and molecular networks4,5 have molecular architectures that offer properties such as gas storage,6,7 catalysis,8,9 magnetism10,11 and luminescence.12–14 The generation of such molecular species is based on the formation of coordination bonds between organic coordinating ligands and metal centres or complexes.15 A large number of coordination polymers that display different connectivity patterns have been generated using different types of polytopic ligands and metals.
Concerning the luminescence properties7 of CPs, special attention has been devoted to lanthanide-based CPs,16–18 owing to their outstanding photoluminescence originating from the triplet excited states of f–f transitions. Lanthanide-based CPs may find applications in luminescence sensing, light emission together with photonics,19–21 and as ligands presenting a strong antenna effect,22 allowing energy transfer from an organic ligand to the metal. The use of macrocyclic ligands for the formation of such assemblies has been documented;23 however, most of the reports are mainly related to porphyrin-based lanthanide CPs.24
Concerning the magnetic properties of lanthanide-based CPs, examples of molecular nanomagnets have been reported earlier.25–27 In addition, LnCPs may combine both SMM behaviour and tunable photoluminescence, as shown recently.28–30
For building new CPs based on macrocycles, the use of calixarene derivatives presents a nice alternative. Calix[4]arenes31–33 are macrocyclic compounds composed of four phenolic moieties bridged by methylene groups as in the “classic” calix[4]arene, CA, L1 (Fig. 1), sulphur atoms in the case of thiacalix[4]arene, TCA, L2 (Fig. 1),34 or hydroxyl or mercapto groups as in tetramercaptothiacalix[4]arene (TMTCA).35,36 These molecules can be easily modified by the functionalization of the upper and/or lower rims of the macrocyclic scaffold. They can adopt four different conformations (cone, partial cone, 1,2-alternate and 1,3-alternate), which make these compounds attractive precursors for designing molecular networks. The generation of coordination polymers with various dimensionalities using tetrasubstituted (tetramercapto)(thia)calix[4]arene derivatives that bear different coordinating sites is well documented.37
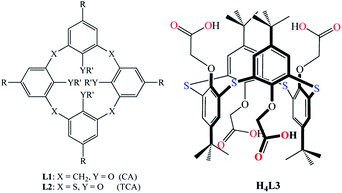 |
| Fig. 1 Calix[4]arene (L1, CA) and thiacalix[4]arene (L2, CA) and tetramethylenecarboxylate derivative of thiacalix[4]arene (H4L3) adopting a 1,3-alternate conformation. | |
By combining functionalized calix[6]arene bearing amide groups, calix[8]arene,38,39 unsubstituted calix[4]arene and thiacalix[4]arene derivatives in cone conformation40–43 with lanthanides salts or mixtures of 3d/4f,44–51 different types of clusters have been obtained. Among them, the use of calixarenes bearing carboxylic coordinating sites, for which the lanthanides cations present great affinity, has been reported for the formation of isolated complexes.52,53
It is important to note that, to date, only a few lanthanide-based coordination networks involving calixarene derivatives, which are mainly formed by the tetrasulfonate derivatives of (thia)calix[4]arenes in cone conformation,54–57 have been reported. To the best of our knowledge, no examples involving carboxylate derivatives or (thia)calix[4]arenes in 1,3-alternate conformation have been reported so far.
Several calixarene derivatives bearing carboxylic/ate coordinating groups have been described. Among them, the compound H4L3 has been used in the formation of coordination polymers with 3d metals, such as Co(II) or Mn(II), and ancillary ligands.58,59 An analogous ligand without the tert-butyl groups has been used for the formation of coordination polymers with Ag+, Ni2+, Co2+, and Zn2+ together with K+.60,61 TMTCA derivative-based coordination polymers using 3d metals have also been reported62 and shown to form hydrogen-bonded networks.63
In this study, we report the structure of a series of isostructural new 1D lanthanide-based coordination networks, derived from tetrasubstituted TCA in 1,3-alternate conformation bearing carboxylate moieties (HL33−). A series of isostructural, homo and heterometallic lanthanide-based periodic architectures (Dy/Tb, because of the isostructurality of the related CPs) has been prepared. Mixing lanthanide cations in solid solution coordination networks can lead to the combination of two phosphorescent centres with large emission differences, which is a common strategy to gain self-referenced luminescence. Heteronuclear lanthanide Gd/Eu or La/Tb, Tb/Eu or Dy/Ln polymers are usually reported for the fine-tuning (colour and brightness) of the corresponding luminescence properties.64–66 Generally, the mixed Eu/Tb compounds allow energy transfer in the mixed coordination polymers due to the optimal energy match between the lanthanide cations.67–72 But tuneable emission can also arise when mixed Tb/Dy coordination compounds are used.73
The ligand fluorescence and Dy3+ and Tb3+ phosphorescence were thoroughly studied in the solid-state in the corresponding (Dy/Tb) solid solutions. In addition, the magnetic properties of the anisotropic Dy3+ compound were investigated in detail.
Results and discussion
Synthesis and structural details
The synthesis of compound H4L3 was achieved following a previously reported procedure that involves a nucleophilic substitution reaction between TCA and ethyl bromoacetate in the presence of Cs2CO3 in acetone, followed by hydrolysis under basic conditions using LiOH in a THF/H2O mixture.63,74,75 The combination of compound H4L3 with lanthanide(III) nitrate salts (Ln = Tb or Dy) under solvothermal conditions in DMF (see Experimental part) led to the formation of isostructural 1D coordination networks. The structural investigation of both single crystals of HL3–Ln (Ln = Dy and Tb) was carried out using X-ray diffraction. The formula derived from the crystallographic analysis was found to be [C48H53O12S4Ln(C3H7NO)3]·(C3H7NO) ([LnIIIHL3DMF3]·(DMF), Ln = Dy or Tb), as shown in Table 1. In the present CP, the ligand/metal ratio is 1/1.
Table 1 Crystallographic parameters for HL3–Dy and HL3–Tb recorded at 173 K
Formula |
C48H53O12S4Dy(C3H7NO)3, C3H7NO, C60H81DyN4O16S4, HL3–Dy |
C48H53O12S4Tb(C3H7NO)3, C3H7NO, C60H81TbN4O16S4, HL3–Tb |
Molecular weight |
1405.02 |
1401.44 |
Crystal system |
Triclinic |
Triclinic |
Space group |
P![[1 with combining macron]](https://www.rsc.org/images/entities/char_0031_0304.gif) |
P![[1 with combining macron]](https://www.rsc.org/images/entities/char_0031_0304.gif) |
a (Å) |
15.0091(17) |
15.0160(17) |
b (Å) |
15.9798(18) |
15.9630(14) |
c (Å) |
16.7401(18) |
16.7660(16) |
α (deg) |
62.217(5) |
62.226(3) |
β (deg) |
67.626(5) |
67.513(3) |
γ (deg) |
79.181(6) |
79.132(4) |
V (Å3) |
3284.5(7) |
3285.2(6) |
Z |
2 |
2 |
Colour |
Colourless |
Colourless |
Crystal dim (mm3) |
0.090 × 0.100 × 0.100 |
0.090 × 0.100 × 0.100 |
Dcalc (g cm−3) |
1.421 |
1.417 |
F(000) |
1454 |
1452 |
μ (mm−1) |
1.332 |
1.270 |
Wavelength (Å) |
0.71073 |
0.71073 |
Number of data meas |
83 469 |
36 284 |
Number of data with I > 2σ(I) |
17 032 [R(int) = 0.0752] |
17 822 [R(int) = 0.0558] |
R |
R1 = 0.0557, wR2 = 0.1391 |
R1 = 0.0494, wR2 = 0.1073 |
Rw |
R1 = 0.0756, wR2 = 0.1559 |
R1 = 0.0708, wR2 = 0.1186 |
GOF |
1.057 |
1.011 |
Largest peak in final difference (eÅ−3) |
1.740 and −1.406 |
1.411 and −1.140 |
The crystals were composed of HL33− in its tris-deprotonated form (see Table 2 for C–O distances), the trivalent Ln cation (Ln(III)) and four DMF solvent molecules. The description of the structure in the solid-state is reported below only for HL3–Tb. The terbium atom was octacoordinated and surrounded by oxygen atoms. Two carboxylate moieties coordinated with the lanthanide in a bidentate mode and the third carboxylate presented a monodentate coordination mode. The 3 oxygen atoms belonging to three DMF molecules completed the coordination sphere of Tb (Fig. 2c). Using the SHAPE program,76 the geometry around the Tb centres was found to be a deformed triangular dodecahedron, as shown in ESI.†
Table 2 Selected bond distances (Å) for HL3–Dy and HL3–Tb
|
HL3–Dy |
HL3–Tb |
C–O (carboxyl-ate/-ic) |
1.232(5) and 1.273(5) |
1.215(4) and 1.269(4) |
1.239(5) and 1.268(4) |
1.253(4) and 1.258(4) |
1.254(5) and 1.261(5) |
1.243(4) and 1.259(4) |
1.189(5) and 1.323(5) (carboxylic) |
1.194(5) and 1.320(5) (carboxylic) |
Ln–O (DMF) |
2.354(3) |
2.355(3) |
2.366(3) |
2.369(2) |
2.380(3) |
2.395(3) |
Ln–O (carboxylate) |
2.256(3) |
2.277(2) |
2.380(3) |
2.393(3) |
2.393(3) |
2.411(3) |
2.404(3) |
2.418(3) |
2.438(3) |
2.440(2) |
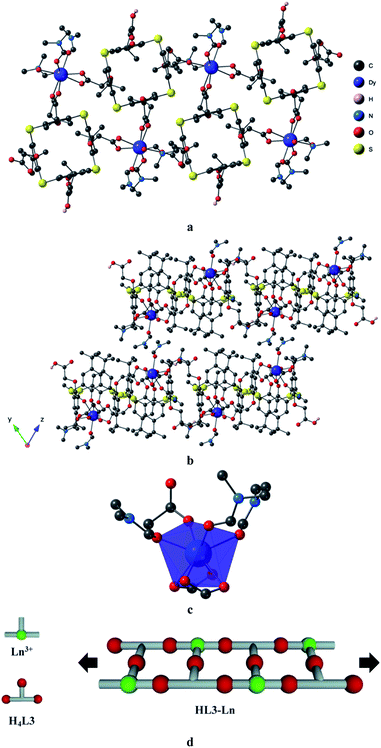 |
| Fig. 2 (a) A portion of the crystal structure of HL3–Tb, (b) crystal packing of HL3–Tb, (c) the coordination environment of Ln3+ and (d) the schematic representation of connectivity in HL3–Tb. | |
The crystal also contained a non-coordinated DMF molecule occupying the interstices between antiparallel 1D coordination networks without forming any specific interactions with them. The terbium atoms acted as T-shaped connectors, connecting three carboxylate groups belonging to three adjacent HL33− ligands, leading to a double chain ladder-like structure (Fig. 2a and b and schematically represented in d). Consequently, the fourth carboxylic group remained protonated without interactions with the other components of the crystal.
As expected, different C–O distances were observed for the carboxylic moieties of HL33−, revealing the presence of one non-coordinated carboxylic group and three coordinated carboxylate moieties (see Table 2).
Within the 1-D arrays, the shortest Tb–Tb distance was equal to 11.879(3) Å, and the packing of the 1D networks in the yOz plane (Fig. 2b, packing along the a-axis) led to a shorter Tb–Tb distance of 7.836(4) Å between two Tb atoms belonging to two different chains.
Since both compounds HL3–Dy and HL3–Tb were isostructural (which is not the case for Gd and Eu analogues, for example), solid solutions of formula HL3–Tb1−xDyx were prepared using the same synthetic procedure as the one used for HL3–Dy and HL3–Tb (see Experimental section). Meanwhile, in our case, the related Eu compound obtained with H4L3 was not isostructural with HL3–Tb or HL3–Dy.
Starting with different experimental Dy/Tb ratios, microcrystalline compounds of the following formula were obtained: HL3–Tb0.07Dy0.93, HL3–Tb0.20Dy0.80, HL3–Tb0.42Dy0.58, HL3–Tb0.67Dy0.33 and HL3–Tb0.95Dy0.05.
The structure of the solid solution was investigated by XRPD, as shown in Fig. 3 (for the determined cell parameters of the corresponding single crystals, see Table S1, ESI†). XRPD clearly demonstrated the structural homogeneity of the phase of the formed coordination compounds. All the HL3–Tb1−xDyx solid solutions were isostructural and displayed the same structure as those of HL3–Tb or HL3–Dy. Since there was only one type of crystallographically independent Ln ion in the unit cell, owing to similar size of both lanthanide cations, one may assume a statistical distribution of the lanthanide cations in the crystal.
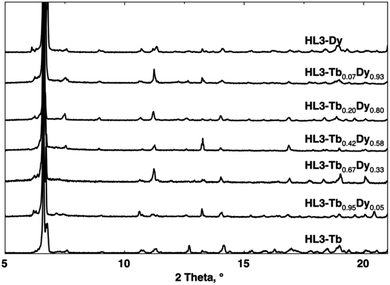 |
| Fig. 3 Comparison of the simulated PXRD patterns for HL3–Tb (bottom) and HL3–Dy (top) and the recorded PXRD patterns for HL3–Tb1−xDyx solid solutions. Discrepancies in intensity between the observed and simulated patterns are due to the preferential orientations of the microcrystalline powders. | |
The composition of the HL3–Tb1−xDyx solid solutions was also checked using energy-dispersive X-ray spectroscopy (EDS), as shown in ESI.†
The experimentally determined Tb/Dy ratios measured were in good agreement with the relative quantities of the lanthanide salts used for synthesis. The slight differences were probably due to weighing inaccuracy and the overestimation of Tb3+ content in the EDS measurements (Table 3).
Table 3 Comparison of the observed and experimental Tb/Dy ratios in HL3–Tb1−xDyx coordination polymers
|
Tb/Dy experimental ratio |
Tb/Dy observed ratio (EDS) |
HL3–Tb0.95Dy0.05 |
95 : 05 |
95 : 05 |
HL3–Tb0.67Dy0.33 |
60 : 40 |
67 : 33 |
HL3–Tb0.42Dy0.58 |
40 : 60 |
42 : 58 |
HL3–Tb0.20Dy0.80 |
20 : 80 |
20 : 80 |
HL3–Tb0.07Dy0.93 |
10 : 90 |
07 : 93 |
The luminescence and magnetic properties of this HL3–Dy series of compounds are presented below.
Photoluminescence properties
For all the investigated compounds, the measurements were carried out in the solid-state using polycrystalline powders.
Among the bands of the excitation spectra of HL3–Tb and HL3–Dy at RT in the 350–500 nm region with emission at λem = 534 nm and 576 nm, respectively (see Fig. S1, ESI†), those attributed to the typical f–f transitions of the Tb3+ and Dy3+ cations presented lower intensities compared with the band attributed to the π → π* transitions of H4L3 (300–350 nm). These observations clearly indicated the occurrence of an energy transfer from the excited level of ligand HL33− to the emitting state of the Tb3+ (or Dy3+) cations, as seen in the case of antenna effect.77 The capability of calixarene carboxylate derivatives to act as antennae for Tb3+ complexes has already been documented.53
The room-temperature emission spectra of the HL3–Tb and HL3–Dy coordination networks, when excited at λex = 350 nm, are depicted in Fig. 4. For HL3–Tb, the emission spectrum is composed of the characteristic five electronic transitions, i.e., 5D4 → 7F6, 5D4 → 7F5 (strongest), 5D4 → 7F4, 5D4 → 7F3 and 5D4 → 7F2 (weakest), whereas for HL3–Dy, the 4F9/2 → 6H15/2, 4F9/2 → 6H13/2 (strongest) and 4F9/2 → 6H11/2 (weakest) electronic transitions are observed, as shown in Fig. 4a and b, respectively. These were typical f–f transitions with emission in the green region for Tb3+ and yellow-white region for Dy3+. The corresponding emission spectra at 77 K are presented in Fig. S2a and b, ESI.†
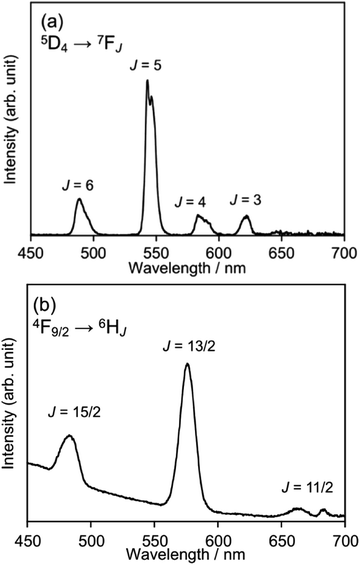 |
| Fig. 4 Emission spectra (λex = 350 nm) of HL3–Tb (a) and HL3–Dy (b) at RT. | |
The photophysical behaviour of the mixed HL3–Tb1−xDyx solid solutions was analysed at RT and 77 K. The luminescence spectra of the HL3–Tb1−xDyx solid solutions observed at RT and 77 K are shown in Fig. 5 and S3.†
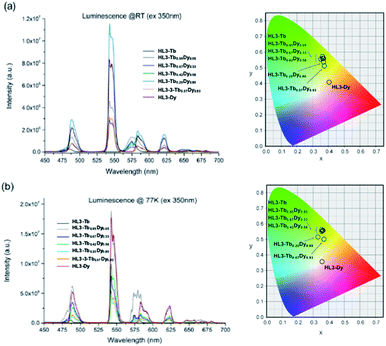 |
| Fig. 5 Emission spectra (λex = 350 nm) of HL3–Dy (black) and HL3–Tb (brown), and the solid solutions HL3–Tb0.07Dy0.93 (grey), HL3–Tb0.20Dy0.80 (blue), HL3–Tb0.42Dy0.58 (green), HL3–Tb0.67Dy0.33 (light blue) and HL3–Tb0.95Dy0.05 (orange) at (a) room temperature and (b) 77 K and the corresponding emission colours presented on the CIE 1931 chromaticity diagrams. | |
At RT, the HL3–Tb1−xDyx solid solutions exhibited the typical luminescence bands of the f–f transitions of Tb3+ at 545 nm and those of Dy3+ at 574 nm. The band intensity at 545 nm decreased when x was decreased, and that at 574 nm increased when x was decreased, as shown in Fig. 5a and b. The introduction of Dy3+ in the HL3–Tb1−xDyx solid solutions allowed slight tuning of the emission colour ranging from pale green to pale yellow, as shown in Fig. 5a and b.
The absolute quantum yields of Tb3+ in the series of HL3–Tb1−xDyx solid solutions were observed (see Fig. S3a and b, ESI†), and the values at 77 K were found to be 58.9% (27.9% at RT) for HL3–Tb, 32.6% for HL3–Tb0.95Dy0.05, and 15.1% for HL3–Tb0.20Dy0.80. Therefore, the existence of Dy3+ ion in the solid solution played a role in decreasing the luminescence quantum yields of the HL3–Tb1−xDyx solid solutions.
The luminescence decay profile and fitted results are summarized in Tables S2 and S3 and Fig. S3–S5 in ESI.† As described above, the luminescence quantum yields of Dy3+ in the neat and solid solutions with Tb3+ were quite low, and it was not possible to obtain the decay profiles at both RT and 77 K for HL3–Dy. The lifetimes of HL3–Tb at RT and 77 K were estimated as a single component, and the τ values were 0.866 and 0.916 ms, respectively. After the addition of Dy3+ in the HL3–Tb1−xDyx solid solutions, the τ values decreased, and the number of luminescence components of Tb3+ increased to two or three (see Tables S2 and S3 in ESI†). The luminescence decay curve of HL3–Tb0.2Dy0.8 could be divided into two components, namely 0.378 and 0.932 ms (λ = 543 nm), at RT. The latter value corresponded to the one observed for HL3–Tb. The second and third emission lifetimes were related to the increasing number of Dy3+ centers surrounding the Tb3+ centers.
The τobs values (Tables S2 and S3 in ESI†) were in accordance with those reported for other calixarene-based coordination compounds in the solid-state.53,78
Magnetic measurements
As HL3–Dy contains the magnetically anisotropic Dy3+ cations, which may lead to a potential single-molecule magnet character, its magnetic properties were investigated. The magnetic behaviour of HL3–Tb is not presented here because of the lack of significant anisotropy for Tb. As shown in Fig. 6, at 300 K, the χMT product reached 14.1 cm3 mol−1 K, which is the exact value expected for free-ion approximation. A typical decrease in the χMT product was observed upon cooling, which could be related to thermal depopulation within the mJ levels of the ground 6H15/2 multiplet of Dy3+. Besides a slow decrease in the signal, the χMT versus T plot did not display abrupt changes, which suggested the lack of magnetic interactions down to 1.8 K as a result of the efficient magnetic isolation of lanthanide centres in the crystal lattice. Magnetization measured at T = 1.8 K increased quickly with increasing magnetic field to 10 kOe and much slower at higher fields, reaching 5.4 μB at 60 kOe without saturation, as expected for anisotropic Dy3+ (inset in Fig. 6).79
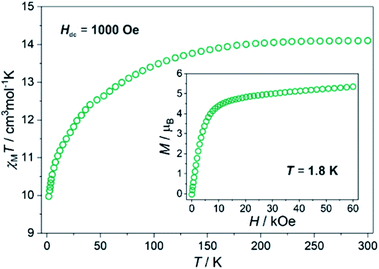 |
| Fig. 6 The temperature dependence of the χMT product of HL3–Dy at Hdc = 1000 Oe, and the field dependence of molar magnetization at T = 1.8 K (inset). | |
To investigate the slow magnetic relaxation effects in HL3–Dy, the alternate-current (ac) magnetic characteristics were gathered at Hdc = 0 (Fig. 7a). The maxima of the frequency dependence of out-of-phase magnetic susceptibility, χM”, appeared on the edge of the measurement range, even at 1.8 K, quickly moving towards higher frequencies while the temperature increased. This indicated the presence of slow magnetic relaxation, a characteristic effect of the single-molecule magnet (SMM) behaviour. To slow down the relaxation by reducing the presumably disturbing quantum tunnelling of the magnetization (QTM) process, an external dc magnetic field was applied (see Fig. S7, ESI†). The field-variable ac magnetic characteristics were analysed using the generalized Debye model for a single relaxation.80 The extracted relaxation times were plotted against the magnetic field, and the resulting dependence was analysed by taking into account the QTM effect (Quantum Tunnelling of the Magnetisation), which was dominant at low fields, and a field-induced direct process responsible for the acceleration of the relaxation process at higher fields (see ESI, Fig. S7†).81 An equilibrium between these processes appeared at H = 1000 Oe, which was selected as the optimal dc field to investigate magnetic relaxation in HL3–Dy. The dc magnetic characteristics were gathered in the 1.8–5 K temperature range (Fig. 7b and see Fig. S8, ESI†). They were analysed using the generalized Debye model for a single relaxation, and the resulting relaxation times are presented as the function of temperature (Fig. 7c).
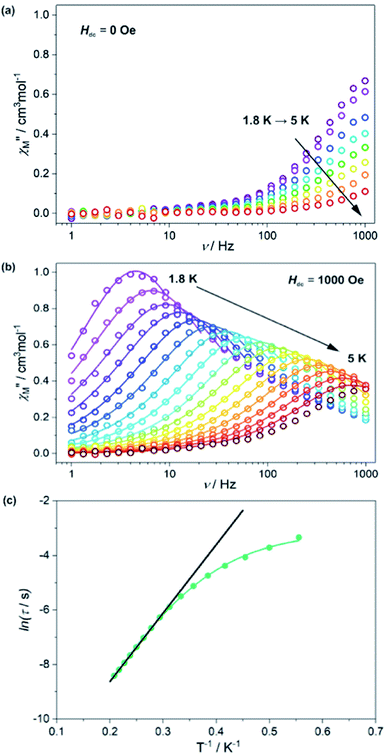 |
| Fig. 7 For HL3–Dy: (a) Zero dc field χM”(ν) curves in the range of 1.8–5 K, (b) dc field χM”(ν) curves in the range 1.8–5 K under a dc field of 1 kOe, and (c) temperature dependence of the relaxation time, τ, at 1 kOe, fitted using the Arrhenius law in the limited 3.4–5 K range (extrapolated black line) and within the full 1.8–5 K range using the contributions from the Orbach, Raman, direct and QTM relaxation processes (green line; see text for details). | |
In the higher temperature range of 3.4–4.8 K, the temperature dependence of the relaxation times was close to linear, and thus, it could be analysed by a simple Arrhenius law, which is presented in eqn (1):
|
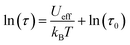 | (1) |
where
Ueff represents the effective thermal energy barrier, while
τ0 is the relaxation attempt time for spin reversal at infinite temperature. The obtained parameters
Ueff/
kB = 25.08(9) K,
τ0 = 1.2(2) × 10
−6 s were within the characteristic limits of single-molecule magnets with moderate magnetic anisotropy.
79 More precisely, the full temperature dependence curve of the relaxation times was fitted using contributions from the Orbach and Raman relaxation processes together with direct and QTM processes taken from the previous analysis of field-dependent relaxation. Therefore,
eqn (2) was used:
|
 | (2) |
where the first term represents the Orbach process related to the Arrhenius law, the second introduces a two-phonon Raman relaxation pathway, the third denotes the QTM effect and the last one shows a direct process.
82,83 To avoid over-parameterization, the two last terms describing the QTM effect and direct process taken from the field-dependence analysis and the related parameters were not optimized during the fitting procedure (Fig. S8
†). The obtained values
Ueff/
kB = 31(3) K and
τ0 = 1.2(7) × 10
−6 s were in good agreement with those extracted from the Arrhenius law. The Raman process had to be included with
BRaman = 0.27(1) s
−1 K
−n and
n = 6.0(3). Thus, the last value expected for lanthanide-based SMMs would be located in the 2–9 range.
39 As several examples of SMMs lacking the Orbach process have been reported,
84 we tried to fit the temperature dependence of the relaxation times excluding Orbach process; however, it was not possible to obtain a satisfying fit.
Based on these results, we could clearly postulate that the Dy3+ complexes in HL3–Dy exhibit single-molecule magnet characteristics with a moderated thermal energy barrier originating from the Orbach relaxation process and a strong QTM effect, partially reduced by using the external dc magnetic field.
Conclusions
In this work, we have demonstrated the possibility of growing a series of lanthanide-based isostructural homometallic coordination polymers using thiacalix[4]arene bearing carboxylic moieties. Owing to their isostructural nature, a series of unprecedented heterometallic solid solutions based on the macrocyclic thiacalix[4]arene derivatives were generated. The single crystals of the two isostructural Tb3+- and Dy3+-based coordination polymers (HL3–Tb and HL3–Dy, formula [LnIIIHL3DMF3]·(DMF)) were structurally characterized using X-ray diffraction, and their photophysical properties were investigated. The formation of the 1D ladder-type coordination compounds resulted in the specific binding of the Ln3+ cation to the partially deprotonated organic ligands that behaved as a “T-Shape” connector. In the solid solutions that combined Tb3+ and Dy3+ forming HL3–Tb1−xDyx, the Ln3+ cations are randomly distributed within the crystals.
The photoluminescence properties of the coordination polymers were investigated. Ligand HL33− sensitized the luminescence of the Tb3+ or Dy3+ cations in HL3–Tb and HL3–Dy at RT, and they exhibited the characteristic f–f transition due to the presence of the Ln3+ cations. The introduction of Dy3+ in HL3–Tb allowed the tuning of the emission colour of the solid solutions, which ranged between pale yellow and pale green.
In addition, the magnetic measurements of HL3–Dy revealed significant magnetic anisotropy in the Dy3+ complexes attached to the thiacalix[4]arene moieties, leading to SMM behaviour. Therefore, this series of new coordination polymers can be considered as a novel source of bifunctional magneto-luminescent molecule-based materials.85 We present a novel and elegant pathway for the construction of luminescent molecular nanomagnets by the incorporation of lanthanide ions in coordination chains based on functionalized thiacalix[4]arene ligands.
Experimental methods
X-ray crystallography
Data were collected at 173(2) K on a Bruker Apex-II-CCD diffractometer equipped with an Oxford Cryosystem liquid N2 device using graphite-monochromated Mo-Kα (λ = 0.71073 Å) radiation. For all the structures, diffraction data were corrected for absorption. The structures were solved using SHELXS-97 and refined by full matrix least-squares on F2 using SHELXL-97. Hydrogen atoms were introduced at calculated positions and refined using a riding model.86 The structures can be obtained free-of-charge from the Cambridge Crystallographic Data Centre via www.ccdc.cam.ac.uk/datarequest/cif. CCDC: 1898825 (HL3–Tb) and 1898826 (HL3–Dy).
Powder diffraction studies (PXRD)
The diffraction diagrams were collected on a Bruker D8 diffractometer using monochromatic Cu-Kα radiation in a scanning range of 4 and 40° using a scan step size of 8° min−1.
Elemental analysis
Elemental analysis was performed by the Analysis Service of the Faculty of Chemistry of the University of Strasbourg.
EDS experiments
Elemental analyses using EDS were performed on a Bruker-QUANTAX System, where the EDS Detector was equipped with a GEMINI FE-SEM ULTRA55 (Carl Zeiss). The samples were mixed with colloidal graphite in isopropanol, dried and pressed into pellets. The EDS analysis was conducted at an accelerating voltage of 15 kV.
Photophysical properties
Luminescence and excitation spectra were recorded on a Fluorolog 3-22 (Horiba Jobin Yvon).
The absolute luminescence quantum yields and luminescence lifetimes were determined using an absolute luminescence quantum yield spectrometer C9920-02 (Hamamatsu Photonics K. K.) and a Quantaurus-Tau C11367-12 (Hamamatsu Photonics K. K.), respectively, with pulsed excitation light sources.
Magnetic properties
The magnetic properties were investigated using a Quantum Design MPMS-3 Evercool magnetometer with the powder sample of HL3–Dy dispersed in paraffin oil to avoid the rotation of the crystals under the magnetic field. The magnetic data were corrected for the diamagnetic contributions from the sample, oil and the sample holder.
Synthesis
All reagents were purchased from commercial sources and used without further purification. H4L3 was prepared using a previously reported procedure with some modifications.63,74
Crystallization conditions
HL3–Tb. A solution containing H4L3 (5 mg, 0.005 mmol) dissolved in DMF (1 mL) was mixed with a 0.01 M solution of Tb(NO3)3·6H2O in DMF (1 mL, 0.01 mmol). Then, 2 drops of 1 M HCl were added to the mixture. In a vial closed with a cap, the solution was heated at 80 °C for 3 days, and after cooling to RT, colourless single crystals were obtained (5.3 mg, 73%). They were filtrated and washed with DMF (2 mL) and diethyl ether (2 mL). Formula: C60H81TbN4O16S4, anal. calcd: C, 51.42%; H, 5.83%; N, 4.00%; found: C, 51.45%; H, 5.87%; N, 4.06%.
HL3–Tb0.95Dy0.05. The same procedure was applied starting from a 0.01 M solution of Tb(NO3)3·6H2O in DMF (0.95 mL, 0.0095 mmol) and a 0.01 M solution of Dy(NO3)3·5H2O (0.05 mL, 0.005 mmol). Yield, 4.6 mg (64%). Formula: C60H81Tb0.05Dy0.95N4O16S4, anal. calcd: C, 51.30%; H, 5.81%; N, 3.99%; found: C, 51.38%; H, 5.85%; N, 4.05%.
HL3–Tb0.67Dy0.33. The same procedure was applied starting from a 0.01 M solution of Tb(NO3)3·6H2O in DMF (0.75 mL, 0.0075 mmol) and a 0.01 M solution of Dy(NO3)3·5H2O (0.25 mL, 0.0025 mmol). Yield, 4.5 mg (62%). Formula: C60H81Tb0.67Dy0.33N4O16S4, anal. calcd: C, 51.38%; H, 5.82%; N, 4.00%; found: C, 51.42%; H, 5.96%; N, 4.08%.
HL3–Tb0.42Dy0.58. The same procedure was applied starting from a 0.01 M solution of Tb(NO3)3·6H2O in DMF (0.5 mL, 0.005 mmol) and a 0.01 M solution of Dy(NO3)3·5H2O (0.5 mL, 0.005 mmol). Yield, 5.2 mg (65%). Formula: C60H81Tb0.42Dy0.58N4O16S4, anal. calcd: C, 51.35%; H, 5.82%; N, 3.99%; found: C, 51.52%; H, 5.85%; N, 3.98%.
HL3–Tb0.2Dy0.8. The same procedure was applied starting from a 0.01 M solution of Tb(NO3)3·6H2O in DMF (0.25 mL, 0.0025 mmol) and a 0.01 M solution of Dy(NO3)3·5H2O (0.75 mL, 0.0075 mmol). Yield, 5.8 mg (75%). Formula: C60H81Tb0.2Dy0.8N4O16S4, anal. calcd: C, 51.32%; H, 5.81%; N, 3.99%; found: C, 51.39%; H, 5.83%; N, 4.02%.
HL3–Tb0.07Dy0.93. The same procedure was applied starting from a 0.01 M solution of Tb(NO3)3·6H2O in DMF (0.1 mL, 0.001 mmol) and a 0.01 M solution of Dy(NO3)3·5H2O (0.9 mL, 0.009 mmol). Yield, 5.3 mg (73%). Formula: C60H81Tb0.07Dy0.93N4O16S4, anal. calcd: C, 51.30%; H, 5.81%; N, 3.99%; found: C, 51.45%; H, 5.79%; N, 4.08%.
HL3–Dy. The same procedure was applied starting from a 0.01 M solution of Dy(NO3)3·5H2O in DMF (1 mL, 0.01 mmol). Yield, 4.8 mg (68%). Formula: C60H81O16N4S4Dy, anal. calcd: C, 51.29%; H, 5.81%; N, 3.99%; found: C, 51.35%; H, 5.86%; N, 4.02%.
Conflicts of interest
There are no conflicts to declare.
Acknowledgements
We thank the Russian Science Foundation (grant no. 17-73-20117) for financial support for the synthesis and crystal preparation. Financial supports from the University of Strasbourg, the International Centre for Frontier Research in Chemistry (icFRC), Laboratory of excellence LabEx CSC, Strasbourg, the Institut Universitaire de France, the CNRS, and MEXT Grants-in-Aid for Scientific Research on Innovative Areas of “Soft Crystals (Area Number: 2903, No. 17H06374) are acknowledged. Center for Instrumental Analysis, College of Science and Engineering, Aoyama Gakuin University also supports to analyse EDS.
Notes and references
- B. F. Abrahams, B. F. Hoskins and R. Robson, A new type of infinite 3D polymeric network containing 4-connected, peripherally-linked metalloporphyrin building blocks, J. Am. Chem. Soc., 1991, 113, 3606–3607 CrossRef CAS.
- S. R. Batten and R. Robson, Interpenetrating Nets: Ordered, Periodic Entanglement, Angew. Chem., Int. Ed., 1998, 37, 1460–1494 CrossRef PubMed.
- Special issue on Metal–Organic Frameworks: Chem. Rev., 2012, 112 Search PubMed.
- M. W. Hosseini, Reflexion on molecular tectonics, CrystEngComm, 2004, 6, 318–322 RSC.
- M. W. Hosseini, Molecular Tectonics:
From Simple Tectons to Complex Molecular Networks, Acc. Chem. Res., 2005, 38, 313–323 CrossRef CAS PubMed. - G. Férey, C. Serre, T. Devic, G. Maurin, H. Jobic, P. L. Llewellyn, G. De Weireld, A. Vimont, M. Daturi and J.-S. Chang, Why hybrid porous solids capture greenhouse gases?, Chem. Soc. Rev., 2011, 40, 550–562 RSC.
- H.-C. Zhou, J. R. Long and O. M. Yaghi, Introduction to Metal–Organic Frameworks, Chem. Rev., 2012, 112, 673–674 CrossRef CAS PubMed.
- L. Q. Ma, C. Abney and W. B. Lin, Enantioselective catalysis with homochiral metal–organic frameworks, Chem. Soc. Rev., 2009, 38, 1248–1256 RSC.
- M. Yoon, R. Srirambalaji and K. Kim, Homochiral Metal–Organic Frameworks for Asymmetric Heterogeneous Catalysis, Chem. Rev., 2012, 112, 1196–1231 CrossRef CAS PubMed.
- P. Dechambenoit and J. R. Long, Microporous magnets, Chem. Soc. Rev., 2011, 40, 3249–3265 RSC.
- G. M. Espallargas and E. Coronado, Magnetic functionalities in MOFs: from the framework to the pore, Chem. Soc. Rev., 2018, 47, 533–557 RSC.
- M. D. Allendorf, C. A. Bauer, R. K. Bhakta and R. J. T. Houk, Luminescent metal–organic frameworks, Chem. Soc. Rev., 2009, 38, 1330–1352 RSC.
- B. Chen, S. Xiang and G. Qian, Metal–Organic Frameworks with Functional Pores for Recognition of Small Molecules, Acc. Chem. Res., 2010, 43, 1115–1124 CrossRef CAS PubMed.
- Y. Cui, Y. Yue, G. Qian and B. Chen, Luminescent Functional Metal–Organic Frameworks, Chem. Rev., 2012, 112, 1126–1162 CrossRef CAS.
- Advances in Inorganic chemistry, Supramolecular Chemistry, ed. R. van Eldik and R. Puchta, Academic Press, 2018 Search PubMed.
- J. Rocha, L. D. Carlos, F. A. A. Paz and D. Ananias, Luminescent multifunctional lanthanides-based metal–organic frameworks, Chem. Soc. Rev., 2011, 40, 926–940 RSC.
- Y. Cui, B. Chen and G. Qian, Lanthanide metal–organic frameworks for luminescent sensing and light-emitting applications, Coord. Chem. Rev., 2014, 273–274, 76–86 CrossRef CAS.
- Y. Cui, B. Li, H. He, W. Zhou, B. Chen and G. Qian, Metal–Organic Frameworks as Platforms for Functional Materials, Acc. Chem. Res., 2016, 49, 483–493 CrossRef CAS.
- S. Roy, A. Chakraborty and T. K. Maji Qian, Lanthanide–organic frameworks for gas storage and as magneto-luminescent materials, Coord. Chem. Rev., 2014, 273–274, 139 CrossRef CAS.
- Y. Hasegawa and T. Nakanishi, Luminescent lanthanide coordination polymers for photonic applications, RSC Adv., 2015, 5, 338–353 RSC.
- J. Wu, H. Zhang and S. Du, Tunable luminescence and white light emission of mixed lanthanide–organic frameworks based on polycarboxylate ligands, J. Mater. Chem. C, 2016, 1, 3364–3374 RSC.
- S. Tobita, M. Arakawa and I. Tanaka, The paramagnetic metal effect on the ligand localized S1∼–T1 intersystem crossing in the rare-earth-metal complexes with methyl salicylate, J. Phys. Chem., 1985, 89, 5649–5654 CrossRef CAS.
- J. C. G. Bünzli, Review: Lanthanide coordination chemistry: from old concepts to coordination polymers, J. Coord. Chem., 2014, 67, 3706–3733 CrossRef.
- Q. Zha, X. Rui, T. Wei and Y. Xie, Recent advances in the design strategies for porphyrin-based coordination polymers, CrystEngComm, 2014, 16, 7371–7384 RSC.
- J. J. Baldovi, E. Coronado, A. Gaita-Arino, C. Gamer, M. Gimenez-Marques and G. Minguez Espallargas, A Sim-MOF: Three-Dimensional Organisation of Single-Ion Magnets with Anion-Exchange Capabilities, Chem.–Eur. J., 2014, 20, 10695–10702 CrossRef CAS PubMed.
- X. Zhang, V. Vieru, X. Feng, J.-L. Liu, Z. Zhang, B. Na, W. Shi, B.-W. Wang, A. K. Powell, L. F. Chibotaru, S. Gao, P. Cheng and J. R. Long, Influence of Guest Exchange on the Magnetization Dynamics of Dilanthanide Single-Molecule-Magnet Nodes within a Metal–Organic Framework, Angew. Chem., Int. Ed., 2015, 54, 9861–9865 CrossRef CAS PubMed.
- D.-D. Yin, Q. Chen, Y.-S. Meng, H.-L. Sun, Y.-Q. Zhang and S. Gao, Slow magnetic relaxation in a novel carboxylate/oxalate/hydroxyl bridged dysprosium layer, Chem. Sci., 2015, 6, 3095–3101 RSC.
- S. Mohapatra, B. Rajeswaran, A. Chakraborty, A. Sundaresan and T. K. Maji, Bimodal Magneto-Luminescent Dysprosium (DyIII)-Potassium (KI)-Oxalate Framework: Magnetic Switchability with High Anisotropic Barrier and Solvent Sensing, Chem. Mater., 2013, 25, 1673–1679 CrossRef CAS.
- R. Jankowski, J. J. Zakrzewski, O. Surma, S. Ohkoshi, S. Chorazy and B. Sieklucka, Near-infrared emissive Er(III) and Yb(III) molecular nanomagnets in metal–organic chains functionalized by octacyanidometallates(IV), Inorg. Chem. Front., 2019, 6, 2423–2434 RSC.
- Y. Xin, J. Wang, M. Zychowicz, J. J. Zakrzewski, K. Nakabayashi, B. Sieklucka, S. Chorazy and S. Ohkoshi, Dehydration–Hydration Switching of Single-Molecule Magnet Behavior and Visible Photoluminescence in a Cyanido-Bridged DyIIICoIII Framework, J. Am. Chem. Soc., 2019, 141, 18211–18220 CrossRef CAS PubMed.
- C. D. Gutsche in Calixarenes Revised: Monographs in Supramolecular Chemistry, The Royal Society of Chemistry, Cambridge, 1998, vol. 6 Search PubMed.
- Z. Asfari, V. Böhmer, J. Harrowfield and J. Vicens, in Calixarenes 2001, ed. Z. Asfari, V. Böhmer, J. Harrowfield and J. Vicens, Kluwer Academic, Dordrecht, 2001 Search PubMed.
- M. W. Hosseini, ACS Series, ed. G. J. Lumetta, R. D. Rogers and A. S. Gopalan, 2000, vol. 557, p. 296 Search PubMed.
- H. Kumagai, M. Hasegawa, S. Miyanari, Y. Sugawa, Y. Sato, T. Hori, S. Ueda, H. Kamiyama and S. Miyano, Facile synthesis of p-tert-butylthiacalix[4]arene by the reaction of p-tert-butylphenol with elemental sulfur in the presence of a base, Tetrahedron Lett., 1997, 38, 3971–3972 CrossRef CAS.
- P. Rao, M. W. Hosseini, A. De Cian and J. Fischer, Synthesis and structural analysis of mercaptothiacalix[4]arene, Chem. Commun., 1999, 2169–2170 RSC.
- H. Akdas, E. Graf, M. W. Hosseini, A. de Cian, A. Bilyk, B. W. Skelton, G. A. Koutsantonis, I. Murray, J. M. Harrowfield and A. H. White, Koilands from thiophiles: mercury(II) clusters from thiacalixarenes, Chem. Commun., 2002, 1042–1043 RSC.
- A. Ovsyannikov, S. Solovieva, I. Antipin and S. Ferlay, Coordination Polymers based on calixarene derivatives: Structures and properties, Coord. Chem. Rev., 2017, 352, 151–186 CrossRef CAS.
- F. M. Ramirez, L. Charbonnière, G. Muller and J.-C. G. Bünzli, Tuning the
Stoichiometry of Lanthanide Complexes with Calixarenes: Bimetallic Complexes with a Calix[6]arene Bearing Ether-Amide Pendant Arms, Eur. J. Inorg. Chem., 2004, 2348–2355 CrossRef CAS.
- K. Z. Su, F. L. Jiang, J. J. Qian, J. D. Pang, F. L. Hu, S. M. Bawaked, M. Mokhtar, S. A. Al-Thabaiti and M. Hong, Synthesis and characterization of decanuclear Ln(III) cluster of mixed calix[8]arene-phosphonate ligands (Ln = Pr, Nd), Inorg. Chem. Commun., 2015, 54, 34–37 CrossRef CAS.
- T. Kajiwara, N. Iki and M. Yamashita, Transition metal and lanthanide cluster complexes constructed with thiacalix[n]arene and its derivatives, Coord. Chem. Rev., 2007, 251, 1734–1746 CrossRef CAS.
- B. S. Creavena, D. F. Donlona and J. McGinley, Coordination chemistry of calix[4]arene derivatives with lower rim functionalisation and their applications, Coord. Chem. Rev., 2009, 253, 893–962 CrossRef.
- S. Sanz, R. D. McIntosh, C. M. Beavers, S. J. Teat, M. Evangelisti, E. K. Brechin and S. J. Dalgarno, Calix[4]arene-supported rare earth octahedra, Chem. Commun., 2012, 48, 1449–1451 RSC.
- J. P. Chinta, B. Ramanujam and C. P. Rao, Structural aspects of the metal ion complexes of the conjugates of calix[4]arene: Crystal structures and computational models, Coord. Chem. Rev., 2012, 256, 2762–2794 CrossRef CAS.
- Y. F. Bi, Y. L. Li, W. P. Liao, H. J. Zhang and D. Q. Li, A Unique Mn2Gd2 Tetranuclear Compound of p-tert-Butylthiacalix[4]arene, Inorg. Chem., 2008, 47, 9733–9734 CrossRef CAS PubMed.
- Y. F. Bi, X. T. Wang, B. W. Wang, W. P. Liao, X. F. Wang, H. J. Zhang, S. Gao and D. Q. Li, Two MnII2LnIII4 (Ln = Gd, Eu) hexanuclear compounds of p-tert-butylsulfinylcalix[4]arene, Dalton Trans., 2009, 12, 2250–2254 RSC.
- G. Karotsis, S. Kennedy, S. J. Teat, C. M. Beavers, D. A. Fowler, J. J. Morales, M. Evangelisti, S. J. Dalgarno and E. K. Brechin, [MnIII4LnIII4] Calix[4]arene Clusters as Enhanced Magnetic Coolers and Molecular Magnets, J. Am. Chem. Soc., 2010, 132, 12983–12990 CrossRef CAS PubMed.
- S. Sanz, K. Ferreira, R. D. McIntosh, S. J. Dalgarno and E. K. Brechin, Calix[4]arene-supported FeIII2LnIII2 clusters, Chem. Commun., 2011, 47, 9042–9044 RSC.
- Y. Bi, S. Du and W. Liao, Thiacalixarene-based nanoscale polyhedral coordination cages, Coord. Chem. Rev., 2014, 276, 61–72 CrossRef CAS.
- M. Coletta, R. McLellan, A. Waddington, S. Sanz, K. J. Gagnon, S. J. Teat, E. K. Brechin and S. J. Dalgarno, Core expansion of bis-calix[4]arene-supported clusters, Chem. Commun., 2016, 52, 14246–14249 RSC.
- M. Coletta, R. McLellan, S. Sanz, K. J. Gagnon, S. J. Teat, E. K. Brechin and S. J. Dalgarno, A New Family of 3d–4f Bis-Calix[4]arene-Supported Clusters, Chem.–Eur. J., 2017, 23, 14073–14079 CrossRef CAS PubMed.
- H. Han, X. Li, X. Zhu, G. Zhang, S. Wang, X. Hang, J. Tang and W. Liao, Single-Molecule-Magnet Behavior in a Calix[8]arene-Capped {Tb6IIICrIII} Cluster, Eur. J. Inorg. Chem., 2017, 2088–2093 CrossRef CAS.
- A. Jäschke, M. Kischel, A. Mansel and B. Kersting, Hydroxyquinoline–Calix[4]arene Conjugates as Ligands for Polynuclear Lanthanide Complexes: Preparation, Characterization, and Properties of a Dinuclear EuIII Complex, Eur. J. Inorg. Chem., 2017, 894–901 CrossRef.
- R. S. Viana, C. A. F. Oliveira, J. Chojnacki, B. S. Barros, S. Alves Jr and J. Kulesza, Structural and spectroscopic investigation of new luminescent hybrid materials based on calix[4]arene-tetracarboxylate
and Ln3+ ions (Ln = Gd, Tb or Eu), J. Solid State Chem., 2017, 251, 26–32 CrossRef CAS.
- S. J. Dalgarno, M. J. Hardie, J. L. Atwood and C. L. Raston, Bilayers, Corrugated Bilayers, and Coordination Polymers of p-Sulfonatocalix[6]arene, Inorg. Chem., 2004, 43, 6351–6356 CrossRef CAS PubMed.
- W. Liao, C. Liu, X. Wang, G. Zhu, X. Zhao and H. Zhang, 3D metal–organic frameworks incorporating water-soluble tetra-p-sulfonatocalix[4]arene, CrystEngComm, 2009, 11, 2282–2284 RSC.
- I. Ling, Y. Alias and C. L. Raston, Structural diversity of multi-component self-assembled systems incorporating p-sulfonatocalix[4]arene, New J. Chem., 2010, 34, 1802–1811 RSC.
- I. Ling and C. L. Raston, Primary and secondary directing interactions of aquated lanthanide(III) ions with p-sulfonated calix[n]arene, Coord. Chem. Rev., 2018, 375, 80–105 CrossRef CAS.
- K. Kim, S. Park, K.-M. Park, K.-M. Park and S. S. Lee, Thiacalix[4]arene-Based Three-Dimensional Coordination Polymers Incorporating Neutral Bridging Coligands, Cryst. Growth Des., 2011, 11, 4059–4067 CrossRef CAS.
- Z. Zhang, A. Drapailo, Y. Matvieiev, L. Wojtas and M. J. Zaworotko, A calixarene based metal organic material, calixMOM, that binds potassium cations, Chem. Commun., 2013, 8353–8355 RSC.
- H. Akdas, E. Graf, M. W. Hosseini, A. De Cian and J. McB. Harrowfield, Design, synthesis and structural investigation of a 2-D coordination network based on the self-assembly of the tetracarboxylate derivative of tetrathiacalix[4]arene, Commun, 2000, 2219–2220 RSC.
- J.-Y. Kim, K. Kim, K.-M. Park and S. S. Lee, Isostructural Heteronuclear (K+/M2+: M = Ni, Co, and Zn) One-Dimensional Coordination Polymers of Thiacalix[4]arene Tetraacetate, Bull. Korean Chem. Soc., 2014, 35, 289–292 CrossRef CAS.
- A. S. Ovsyannikov, S. Ferlay, S. E. Solovieva, I. S. Antipin, A. I. Konovalov, N. Kyritsakas and M. W. Hosseini, Molecular tectonics: high dimensional coordination networks based on methylenecarboxylate-appended tetramercaptothiacalix[4]arene in the 1,3-alternate conformation, CrystEngComm, 2018, 20, 1130–1140 RSC.
- A. S. Ovsyannikov, M. Lang, S. Ferlay, S. E. Solovieva, I. S. Antipin, A. I. Konovalov, N. Kyritsakas and M. W. Hosseini, Molecular tectonics: tetracarboxythiacalix[4]arene derivatives as tectons for the formation of hydrogen-bonded networks, CrystEngComm, 2016, 18, 8622–8630 RSC.
- K. A. White, D. A. Chengelis, K. A. Gogick, J. Stehman, N. L. Rosi and S. Petoud, Near-Infrared Luminescent Lanthanide MOF Barcodes, J. Am. Chem. Soc., 2009, 131, 18069–18071 CrossRef CAS PubMed.
- S. Dang, J. H. Zhang and Z. M. Sun, Tunable emission based on lanthanide(III) metal–organic frameworks: an alternative approach to white light, J. Mater. Chem., 2012, 22, 8868–8873 RSC.
- V. Haquin, M. Etienne, C. Daiguebonne, S. Freslon, G. Calvez, K. Bernot, L. Le Pollès, S. E. Ashbrook, M. R. Mitchell, J.-C. Bünzli, S. V. Eliseeva and O. Guillou, Color and Brightness Tuning in Heteronuclear Lanthanide Terephthalate Coordination Polymers, Eur. J. Inorg. Chem., 2013, 3464–3476 CrossRef CAS.
- D. T. de Lill, N. S. Gunning and C. L. Cahill, Toward Templated Metal–Organic Frameworks:
Synthesis, Structures, Thermal Properties, and Luminescence of Three Novel Lanthanide–Adipate Frameworks, Inorg. Chem., 2005, 44, 258–266 CrossRef CAS PubMed. - K. Liu, H. You, Y. Zheng, G. Jia, Y. Song, Y. Huang, M. Yang, J. Jia, N. Guo and H. Zhang, Facile and rapid fabrication of metal–organic framework nanobelts and color-tunable photoluminescence properties, J. Mater. Chem., 2010, 20, 3272–3279 RSC.
- S. Mohapatra, S. Adhikari, H. Riju and T. K. Maji, Terbium(III), Europium(III), and Mixed Terbium(III)–Europium(III) Mucicate Frameworks: Hydrophilicity and Stoichiometry-Dependent Color Tunability, Inorg. Chem., 2012, 51, 4891–4893 CrossRef CAS PubMed.
- Y. Cui, H. Xu, Y. Yue, Z. Guo, J. Yu, Z. Chen, J. Gao, Y. Yang, G. Qian and B. Chen, A Luminescent Mixed-Lanthanide Metal–Organic Framework Thermometer, J. Am. Chem. Soc., 2012, 134, 3979–3982 CrossRef CAS PubMed.
- A. R. Ramya, D. Sharma, S. Natarajan and M. L. P. Reddy, Highly Luminescent and Thermally Stable Lanthanide Coordination Polymers Designed from 4-(Dipyridin-2-yl)aminobenzoate: Efficient Energy Transfer from Tb3+ to Eu3+ in a Mixed Lanthanide Coordination Compound, Inorg. Chem., 2012, 51, 8818–8826 CrossRef CAS PubMed.
- S. Sato, A. Ishii, C. Yamada, J. Kim, H. S. Chul, A. Fujiwara, M. Takata and M. Hasegawa, Luminescence of fusion materials of polymeric chain-structured lanthanide complexes, Polym. J., 2015, 47, 195–200 CrossRef CAS.
- K. Kumar, S. Chorazy, K. Nakabayashi, H. Sato, B. Sieklucka and S.-I. Ohkoshi, TbCo and Tb0.5Dy0.5Co layered cyanido-bridged frameworks for construction of colorimetric and ratiometric luminescent thermometers, J. Mater. Chem. C, 2018, 6, 8372–8384 RSC.
- H. Akdas, G. Mislin, E. Graf, M. Hosseini, A. De Cian and J. Fisher, Synthesis and solid state structural analysis of conformers of tetrakis((ethoxycarbonyl)methoxy)tetrathiacalix[4]arene, Tetrahedron Lett., 1999, 40, 2113–2116 CrossRef CAS.
- N. Iki, N. Morohashi, F. Narumi, T. Fujimoto, T. Suzuki and S. Miyano, Novel molecular receptors based on a thiacalix[4]arene platform. Preparations of the di- and tetracarboxylic acid derivatives and their binding properties towards transition metal ions, Tetrahedron Lett., 1999, 40, 7337–7341 CrossRef CAS.
- The program is available free of charge at http://www.ee.ub.edu/.
- G. F. de Sá, O. L. Malta, C. M. Donegá, A. M. Simas, R. L. Longo, P. A. Santa-Cruz and E. F. da Silva Jr, Spectroscopic properties and design of highly luminescent lanthanide coordination complexes, Coord. Chem. Rev., 2000, 196, 165–195 CrossRef.
- M. F. Hazenkamp, G. Blasse, N. Sabbatini and R. Ungaro, The solid state luminescence of the encapsulation complex of Eu3+ in p-t-butyl-calix[4] arene tetra-amide, Inorg. Chim. Acta, 1990, 172, 93–95 CrossRef CAS.
- D. Woodruff, R. E. P. Winpenny and R. A. Layfield, Lanthanide Single-Molecule Magnets, Chem. Rev., 2013, 113, 5110–5148 CrossRef CAS.
- Y.-N. Guo, G.-F. Xu, Y. Guo and J. Tang, Relaxation dynamics of dysprosium(III) single molecule magnets, Dalton Trans., 2011, 40, 9953–9963 RSC.
- A. Amjad, A. Figuerola and L. Sorace, Tm(III) complexes undergoing slow relaxation of magnetization: exchange coupling and aging effects, Dalton Trans., 2017, 46, 3848–3856 RSC.
- K. S. Pedersen, J. Dreiser, H. Weihe, R. Sibile, H. V. Johannesen, M. A. Sorensen, B. E. Nielsen, M. Sigrist, H. Mutka, S. Rols, J. Bendix and S. Piligkos, Design of Single-Molecule Magnets: Insufficiency of the Anisotropy Barrier as the Sole Criterion, Inorg. Chem., 2015, 54, 7600–7606 CrossRef CAS PubMed.
- S. Chorazy, J. J. Zakrzewski, M. Reczyński, K. Nakabayashi, S. Ohkoshi and B. Sieklucka, Humidity driven molecular switch based on photoluminescent DyIIICoIII single-molecule magnets, J. Mater. Chem. C, 2019, 7, 4164–4172 RSC.
- J. J. Zakrzewski, S. Chorazy, K. Nakabayashi, S. Ohkoshi and B. Sieklucka, Photoluminescent Lanthanide(III) Single-Molecule Magnets in Three-Dimensional Polycyanidocuprate(I)-Based Frameworks, Chem.–Eur. J., 2019, 25, 11820–11825 CrossRef CAS PubMed.
- J.-H. Jia, Q.-W. Li, Y.-C. Chen, J.-L. Liu and M.-L. Tong, Luminescent single-molecule magnets based on lanthanides: Design strategies, recent advances and magneto-luminescent studies, Coord. Chem. Rev., 2019, 378, 365–381 CrossRef CAS.
- G. M. Sheldrick, A short history of SHELX, Acta Crystallogr., Sect. A: Found. Crystallogr., 2008, 64, 112–122 CrossRef CAS PubMed.
Footnote |
† Electronic supplementary information (ESI) available: Analysis using Shape program, measured cells for the solid solutions, EDS analysis for the solid solutions, excitation spectra, absolute luminescence quantum yields, decay profile analysis and details of magnetic measurements. CCDC 1898825 and 1898826. For ESI and crystallographic data in CIF or other electronic format see DOI: 10.1039/d0ra01263g |
|
This journal is © The Royal Society of Chemistry 2020 |
Click here to see how this site uses Cookies. View our privacy policy here.