DOI:
10.1039/D0RA01518K
(Paper)
RSC Adv., 2020,
10, 11766-11776
The impact of phenyl–phenyl linkage on the thermodynamic, optical and morphological behavior of carbazol derivatives†
Received
17th February 2020
, Accepted 11th March 2020
First published on 24th March 2020
Abstract
The impact of structural differentiation between phenylcarbazoles (PhC, mCP, CBP, TCB) and phenylamines (TPA, BDB, TPB, TDAB) on the phase equilibria, optical spectrum, band gap, and thin-film morphology is evaluated and discussed. The carbazolyl units lead to a lower electronic conjugation contributing to a wide band gap when compared with the diphenylamine analogs. The fusion and sublimation equilibria indicate that entropic contribution is the key factor for the distinguished melting behavior and solid-phase volatility between phenylcarbazole derivatives and phenylamine analogs. The molecular differentiation between the two classes of compounds is not reflected in the crystal packing and intermolecular interactions. However, compared with the diphenylamino groups, the incorporation of carbazolyl moieties contributes to a less flexible molecule. Moreover, the results evidence that intermolecular bonding disruption along the fusion transition is more extensive for phenylamine derivatives. Due to the asymmetric nonplanar structure, mCP is characterized by a ratio of {Tg/Tm ≈ 3/4} while the more symmetric CBP and TCB molecules display ratios closer to {Tg/Tm ≈ 2/3}. Vapor-deposited thin films of mCP, CBP, and TCB are amorphous and their morphology is highly dependent on the substrate roughness. The lower flexibility of nonplanar phenylcarbazoles induces the formation of a glassy state due to the harder packing mechanism leading to the lower ability of the crystallization process.
Introduction
The allure of cheaper and more sustainable organic electronic devices such as solar cells (OPVs), field-effect transistors (OFETs) and light-emitting diodes (OLEDs) has brought the attention of the scientific community to investigate and develop stable organic semiconducting materials (OSCs).1–5 Among the semiconductors, hole-transporting materials (HTMs) based on conductive small organic molecules have become one of the hottest topics in molecular electronics.6–10 High-performance HTMs should exhibit high hole mobilities, a low potential of ionization and good thermal stability in the amorphous phase.11–13 Amorphous materials consisting of phenylamine and/or carbazol moieties are usually incorporated as hole-transporting thin films in OLEDs and OPVs.14–18 Thin films of diamine and triphenylamine derivatives (e.g. TPD and NPB) are commonly used as the typical hole transporting layers in OLEDs and efforts have been made to understand their thermophysical characteristics, with emphasis on thermal and transport properties in the thin film state.19–22 Likewise, carbazole derivatives have also gathered research interest due to the low-cost and good charge transport ability of the carbazolyl moiety.23–25 Carbazol unit provides a wide range of charge transport molecules, such as carbazole-based HTMs with triphenylamine moieties, which have been explored as promising candidates for high-performing perovskite solar cells.26–28 Moreover, carbazole compounds are very relevant in developing phosphorescent organic light-emitting diodes (PHOLEDs), where these compounds act as the host materials, mainly due to their high triplet state energy and excellent hole transport capability.29–31 Among the carbazole-based compounds, the 4,4′-bis(N-carbazolyl)-1,1′-biphenyl (CBP) and 1,3-bis(N-carbazolyl)benzene (mCP) have seen wide use in PHOLEDs, with interesting results in light-emitting cells.32,33 In addition to molecular electronic devices, carbazol derivatives are exciting liquid organic hydrogen carriers (LOHCs) and have been investigating by different research groups aiming for sustainable industrial applications.34–39 Despite carbazole derivatives having a recognized technological relevance for energy storage, there is a general lack of reports regarding their thermal behavior, including phase change properties and volatility studies. These properties are also of great importance for the manufacturing technology of thin films by vapor deposition procedures.19,21,40–44 In previous works, thermophysical, optical and morphological studies were carried out for an extended series of aromatic N-phenylamines.19,21 N-Phenylcarbazoles have molecular structures analogous to the phenylamines, with an additional chemical bond between the phenyl groups (phenyl–phenyl linkage) in the carbazolyl unit (Fig. 1).45,46
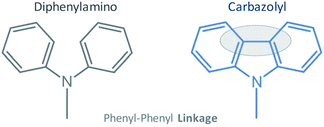 |
| Fig. 1 Structural differentiation between diphenylamino and carbazolyl moieties. | |
In this context, the aim of this work is to acquire a better understanding of the impact of this molecular differentiation on the phase transition equilibria, optical spectrum, band gap, and morphological properties. The molecular structures of carbazol derivatives studied in this work (PhC, mCP, CBP, and TCB) are presented in Fig. 2. Molecular structures of corresponding phenylamines are depicted for comparison.
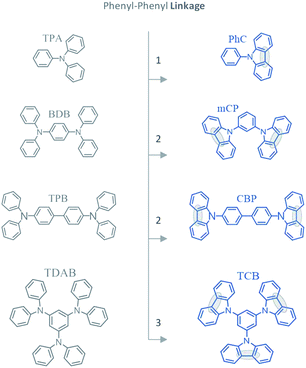 |
| Fig. 2 Molecular structure of the materials studied and the adopted abbreviations: triphenylamine (TPA); 9-phenylcarbazole (PhC); 1,4-bis(diphenylamino)benzene (BDB); 1,3-bis(N-carbazolyl)benzene (mCP); tetra-N-phenylbenzidine (TPB); 4,4′-bis(N-carbazolyl)-1,1′-biphenyl (CBP); 1,3,5-tris(diphenylamino)benzene (TDAB); 1,3,5-tris(N-carbazolyl)benzene (TCB). | |
Experimental section
Materials
The materials studied, 1,3-bis(N-carbazolyl)benzene (mCP; CAS number 550378-78-4; mass fraction 0.997), 4,4′-bis(N-carbazolyl)-1,1′-biphenyl (CBP; CAS number 58328-31-7; mass fraction 0.989), and 1,3,5-tris(N-carbazolyl)benzene (TCB; CAS number 148044-07-9; mass fraction 0.987) were commercially purchased from Sigma-Aldrich/Merck. To obtain high purity samples, the solids CBP and TCB were purified by sublimation under reduced pressure at T = 548 K and T = 573 K, respectively. Prior to fundamental studies and thin film deposition, the volatile content of mCP, CBP, and TCB were removed by vacuum thermal evaporation (p < 10−4 Pa) at T = 448 K, T = 473 K, and T = 523 K, respectively. The high degree of purity (final mass fraction purity of 0.999) of the compounds was checked by gas–liquid chromatography, using an Agilent chromatograph model 4890D, equipped with an HP-5 column and a flame ionization detector (FID). The relative atomic masses used were those recommended by IUPAC.47
UV-visible absorption spectroscopy
UV-vis absorption properties of mCP, CBP and TCB were explored using a diode array spectrophotometer (Agilent 8543 UV-visible spectroscopy system). Absorption spectra in diluted solutions (≈10−5 mol dm−3) using CH2Cl2 as a solvent were recorded over the range of 200–700 nm, using quartz cells with a path length of 10.00 mm maintained at a constant temperature of T = 298.15 K. Temperature control was attained by means of a refrigerated circulator. The optical band gap of each phenylcarbazole derivative was inferred from the offset wavelength of each UV-vis spectrum, according to the methodology described in a previous work.4
Differential scanning calorimetry (DSC)
The melting properties (melting temperatures and enthalpies associated to the solid-to-liquid phase transition) of mCP, CBP and TCB were explored in a heat flux differential scanning calorimeter (NETZSCH, DSC 200 F3 Maia) applying a constant flow of nitrogen (50 cm3 min−1), a heating rate of 2 K min−1 and using hermetically sealed aluminium crucibles (25 μl). For each material (samples of about 5 mg), at least four independent experiments were performed from T = 298 K to a temperature of 20 K higher than their melting temperature. The temperature and heat flux scales of the DSC were calibrated by an accurate determination of the temperature and the enthalpy of fusion of several reference materials48,49 for thermal analysis (o-terphenyl, benzoic acid, indium, triphenylene, tin, perylene, zinc), and the compounds under study were measured using the same experimental method as in the calibration runs.
Knudsen effusion/volatility measurements
The volatility of mCP, CBP, and TCB was explored by a Knudsen effusion methodology based on a quartz crystal microbalance.50 Requiring small amounts of sample and providing accurate experimental data in low effusion times, this method has been commonly applied for the measurement of equilibrium vapor pressures of low volatile organic materials (0.01–1 Pa). The performance and accuracy of the Knudsen effusion approach was evaluated by measuring the equilibrium vapor pressures of 1,3,5-triphenylbenzene, an established reference organic compound for the enthalpy of sublimation and a recommended compound for sublimation studies at relatively high temperatures.42,48–55 The vapor pressure of mCP, CBP, and TCB was determined at different temperature intervals: mCP (453.2 to 478.8 K); CBP (523.2 to 550.6 K); TCB (555.7 to 582.6 K).
Physical vapor deposition (PVD)
Thin films of mCP, CBP, and TCB were prepared through a customized procedure of PVD using the ThinFilmVD apparatus developed in our laboratory.42 The procedural methodology and the capabilities of this technique have been presented in recent reports.21,42,43,56,57 The materials studied were deposited on the surface of three different substrates (maintained at a constant temperature of 293 K): 6 MHz gold-coated quartz crystals; 6 MHz silver-coated quartz crystals; 6 MHz aluminium-coated quartz crystals. Based on the results of Knudsen effusion, thin films were deposited by thermal evaporation in high vacuum conditions (<10−4 Pa) under the same experimental conditions: equilibrium vapor pressure of ≈0.3 Pa; mass flow rate at the substrate surface of ≈22 ng cm−2 s−1; deposition time of 60 minutes; for these experimental conditions, mCP, CBP and TCB were evaporated at 472, 545, and 573 K, respectively. The substrates were cleaned with ethanol and dried with a pure and dry argon gas flow. Vapor-deposited thin film samples were stored in vacuum conditions to avoid contamination.
High-resolution scanning electron microscopy (SEM)
The topography of the vapor-deposited thin films was investigated by scanning electron microscopy (SEM) through the high-resolution FEI Quanta 400 FEG ESEM/EDAX Genesis X4M instrument at the CEMUP (Centro de Materiais da Universidade do Porto) services. SEM micrographs (top views) were acquired using a secondary electron detector (SE). The acceleration voltage was 10 keV, while an in-lens detector was employed with a working distance of about 10 mm.
Results and discussion
Optical properties
The optical properties of each carbazole derivative (diluted solution in CH2Cl2) were examined by means of UV-visible absorption spectroscopy. Fig. 3 presents the experimental UV-vis spectra of mCP, CBP and TCB (Fig. 3A) and the data for corresponding phenylamines (TDAB and TPB) are depicted for comparison (Fig. 3B and C). The detailed spectrum of each compound is presented as ESI.† Table 1 lists the experimental data for the absorption wavelengths at the maxima and corresponding molar absorptivities, absorption edge wavelengths and derived optical band gaps for a series of N-phenylcarbazoles (mCP, CBP and TCB) and corresponding N-phenylamines (BDB, TPB, and TDAB). The UV-vis spectra exhibit absorption peaks in the ultraviolet region (<350 nm) as a result of electronic transitions occurring due to the interaction of radiation with the molecules studied. The so-called “optical gap” of each material can be derived from each spectrum through the onset of the long-wavelength absorption maxima.4,58 Four main absorption peaks, centred at ≈240, 293, 325 and 340 nm, respectively, are observed (Fig. 3A) for each carbazole derivative studied (mCP, CBP and TCB), without any significant bathochromic shift being perceived. Absorption peaks located at the same wavelengths were observed for a diluted solution of 9-phenylcarbazole (PhC) in DCM.59 The absorption peak located at ≈293 nm is appointed to π → π* electronic transition, whereas the peaks located at ≈326 nm and ≈340 nm are assigned to n → π* electronic transitions.59 The absorption peaks relative to π → π* transition of mCP and CBP display similar values of molar absorptivity as both molecules present the same number of carbazolyl units.
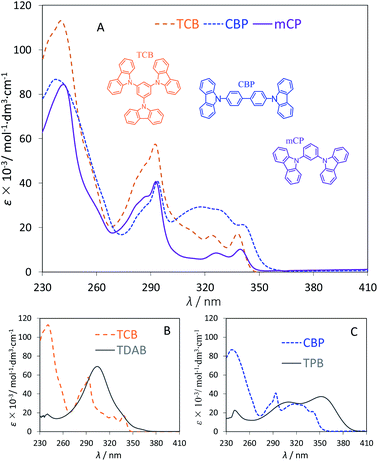 |
| Fig. 3 UV-vis spectra, molar absorptivity (ε) as a function of wavelength (λ), recorded in CH2Cl2, at T = 298.1 K, for mCP, CBP and TCB (A) and UV-vis spectra comparing the carbazol derivative with its corresponding phenylamine: TCB vs. TDAB (B) and CBP vs. TPB (C). | |
Table 1 Wavelengths (λabs.max.) and molar absorptivities (εmax.) at the maxima, absorption edge wavelengths (λabs.edge) and derived optical band gaps (Eoptical gap) for the studied N-phenylcarbazoles (mCP, CBP and TCB). Values for the corresponding N-phenylamines are presented for comparison4
Material |
λabs.max., nm |
εmax.10−3, mol−1 dm3 cm−1 |
λabs.edge, nm |
Eoptical gap, eV |
Data from ref. 4. |
mCP |
242 |
84 |
347 |
3.57 |
293 |
41 |
326 |
9 |
339 |
10 |
CBP |
237 |
87 |
353 |
3.51 |
294 |
41 |
318 |
29 |
341 |
21 |
TCB |
240 |
113 |
345 |
3.59 |
292 |
57 |
324 |
16 |
338 |
17 |
BDBa |
241 |
16 |
390 |
3.19 |
315 |
30 |
TPBa |
241 |
23 |
400 |
3.11 |
310 |
31 |
351 |
37 |
TDABa |
240 |
19 |
375 |
3.31 |
304 |
69 |
The presence of more chromophore groups in TCB, which is constituted by three carbazolyl units, leads to the overlap of the absorption peaks and, consequently, a hyperchromic effect is clearly noted.
The experimental results also indicate that the presence of two central aromatic rings in CBP leads to a hyperchromic effect of the absorption bands peaking at 318 and 341 nm as well as to a reduced optical band gap energy. A comparative analysis between the UV-vis spectra of phenylcarbazoles and phenylamines (Fig. 3B and C) evidences a higher degree of electronic conjugation in TDAB and TPB in comparison with TCB and CBP. Hence, the additional chemical bond between the phenyl groups of the carbazolyl units leads to clear hypsochromic shifts on the absorption UV-vis absorption bands and, consequently, to a higher band gap energy. According to recent reports, the short conjugation of carbazole derivatives leading to a wide band gap is a key factor for the high triplet energy presented by this class of semiconductors.24,60–63 Optical band gaps (Eg) of 3.57, 3.51 and 3.59 eV were estimated from the absorption edge wavelengths (λabs.edge) of the UV-vis spectra for mCP, CBP and TCB, respectively (Eg ≈ 1240/λabs.edge). These values are in nice agreement with literature reports presented by other authors for samples studied in DCM solution and/or in the thin-film state. There are no significant deviations between the values determined in the solution or in the thin film. In fact, carbazole derivatives are materials of relative low polarity and do not establish very specific intermolecular interactions. Moreover, DCM is a suitable solvent that dissolves these materials without affecting their optical behavior, whereby the molecular environment in diluted solutions of carbazole derivatives and in amorphous thin film state can be comparable.
Thermodynamic properties of fusion
An accurate analysis of the melting properties was carried out to evaluate the relative stability of the solid phase in terms of its fusion process. The thermodynamic properties associated with the solid–liquid phase equilibrium of all compounds depicted in Fig. 2 are listed in Table 2. Melting temperatures (Tm) and standard molar enthalpies of fusion (ΔfusH°) were directly obtained from DSC experimental data (determined by the integration of the area under the endothermic curve considering the initial and end inflections of the central peak), and the standard molar entropies of fusion (ΔfusS°) were derived as ΔfusS° = ΔfusH°/Tm. Heat capacity corrections were developed to determine hypothetical thermodynamic properties at 298.15 K. This adjustment was made to evaluate and compare the thermochemical properties of a series of compounds in a more accurate fashion, by establishing the same reference temperature.64,65 The heat capacity corrections of the enthalpies (ΔfusH°), entropies (ΔfusS°) and Gibbs energies (ΔfusG°) of fusion to the reference temperature of θ = 298.15 K, for each phenylcarbazole or phenylamine, were calculated using the eqn (1), (2), and (3), respectively. Following previous reports, the typical and recommended value of
was used as the difference between the liquid and solid phase heat capacity at 298.15 K.19,21,66 In Table 2, the values of Tm and ΔfusH° for PhC, TPA, BDB, TPB, and TDAB were obtained from literature and are listed for comparison.19,21,39,67 Fig. 4 presents an evaluation of the magnitude of enthalpies and entropies of fusion for phenylcarbazoles and phenylamines. The comparison between TPA and PhC, TPB and CBP, and TDAB and TCB evidences that both values of ΔfusH° and ΔfusS° (at the same reference temperature) are clearly higher for phenylamines. The relative stability of a solid phase, in terms of its solid-to-liquid phase transition, is typically evaluated by the magnitude of melting temperature, which results in a balance between enthalpic and entropic contributions.64 The melting temperature of a material can be derived as Tm = ΔfusH°(Tm)/ΔfusS°(Tm). Hence, higher values of ΔfusH° and lower values of ΔfusS° lead to higher melting temperatures. From a comparative analysis between melting points of phenylcarbazoles and their phenylamine analogues, the following conclusions can be drawn: Tm of PhC is 32 K lower than TPA; Tm of CBP is 49 K higher than TPB; Tm of TCB is 71 K higher than TDAB. These findings, together with the data represented in Fig. 4, indicate that entropic contribution is the preponderant thermodynamic property for the differentiation between melting temperatures of phenylcarbazoles and phenylamines. In addition, and contrary to observed between TPB and TDAB where TDAB displays higher values of ΔfusH° and ΔfusS°, a comparison between CBP and TCB shows lower values of ΔfusH° and ΔfusS° for TCB. Due to enthalpy–entropy compensation, in both cases, the compounds with higher molar mass are characterized by larger values of Tm. |
 | (1) |
|
 | (2) |
|
 | (3) |
Table 2 Thermodynamic properties associated to the solid–liquid equilibrium of PhC, mCP, CBP, TCB, TPA, BDB, TPB, and TDAB: melting temperatures (Tm), standard molar enthalpies (ΔfusH°) and entropies (ΔfusS°) of fusion at the melting temperature and derived thermodynamic properties of fusion (ΔfusH°, ΔfusS°, and ΔfusG°) at θ = 298.15 K. Experimental results and literature data
Material |
Tm, K |
ΔfusH°(Tm), kJ mol−1 |
ΔfusS°(Tm), J K−1 mol−1 |
PhCa |
367.9 ± 0.3 |
19.4 ± 0.3 |
52.7 ± 0.8 |
mCP |
453.8 ± 0.2 |
27.1 ± 1.4 |
59.8 ± 2.5 |
CBP |
553.5 ± 1.2 |
42.7 ± 2.8 |
77.2 ± 4.1 |
TCB |
597.5 ± 1.2 |
41.7 ± 2.5 |
69.9 ± 4.2 |
TPAb |
400.2 ± 0.5 |
24.9 ± 0.5 |
62.2 ± 1.2 |
BDBc |
475.4 ± 0.2 |
44.1 ± 0.4 |
92.7 ± 0.8 |
TPBc |
504.6 ± 0.1 |
46.2 ± 0.3 |
91.5 ± 0.6 |
TDABc |
526.3 ± 0.1 |
62.1 ± 0.8 |
117.9 ± 1.5 |
|
Values at θ = 298.15 K |
ΔfusG° |
ΔfusH° |
ΔfusS° |
Data from ref. 39. Data from ref. 67. Data from ref. 19. |
PhC |
3.3 ± 1.0 |
15.9 ± 0.8 |
42.2 ± 2.3 |
mCP |
7.8 ± 2.6 |
19.4 ± 2.1 |
38.8 ± 4.9 |
CBP |
16.2 ± 4.4 |
30.0 ± 3.8 |
46.3 ± 7.4 |
TCB |
16.3 ± 4.6 |
26.8 ± 3.9 |
35.1 ± 8.1 |
TPA |
5.6 ± 1.5 |
19.8 ± 1.1 |
47.5 ± 3.2 |
BDB |
14.6 ± 2.3 |
35.2 ± 1.8 |
69.4 ± 4.7 |
TPB |
16.4 ± 2.6 |
35.9 ± 2.1 |
65.2 ± 5.3 |
TDAB |
24.0 ± 3.0 |
50.7 ± 2.4 |
89.5 ± 5.9 |
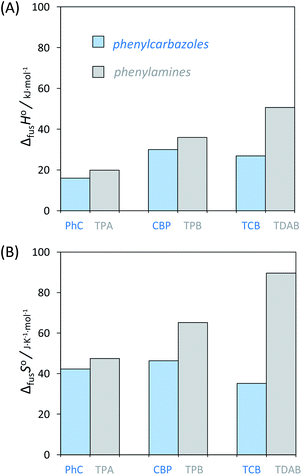 |
| Fig. 4 Schematic representation for the magnitude of enthalpies (ΔfusH°, (A)) and entropies (ΔfusS°, (B)) of fusion, at θ = 298.15 K, for series of phenylcarbazoles (PhC, CBP, TCB) and phenylamines (TPA, TPB, TDAB). | |
The phenylcarbazoles mCP and TCB result from the introduction of additional carbazole groups in meta position into the PhC moiety. That way, we can conclude that the increment of carbazolyl units (in phenylcarbazoles) is followed by increased values of Tm and ΔfusH°. In this case, both enthalpic and entropic factors are contributing to increasing Tm, as the magnitude of ΔfusS° is lower when more carbazoles are included.
Thermodynamic properties of sublimation/vaporization
The solid–gas equilibria of CBP and TCB and the liquid–gas equilibrium of mCP were evaluated determining the equilibrium vapor pressures of the compounds in temperature intervals of about 25 K using the Knudsen effusion procedure (Table 3). The accuracy of the methodology was tested with 1,3,5-triphenylbenzene; the relative deviations between experimental and recommended vapor pressures were found to be less than 2%. In addition, the derived sublimation enthalpies (ΔsubH° = 147.5 ± 1.0 kJ mol−1) and entropies (ΔsubS° = 251.3 ± 2.3 J K−1 mol−1) for 1,3,5-triphenylbenzene, at θ = 298.15 K, agree with the literature values.42,48–55 Details are presented as ESI.† The dependence of vapor pressures with the effusion temperature was used to derive thermodynamic properties of phase transition; considering the mean temperature of each experiment, 〈T〉, molar enthalpies of sublimation (for CBP and TCB, ΔsubH°) and molar enthalpies of vaporization (for mCP, ΔvapH°) were derived using the integrated form of the Clausius–Clapeyron equation, where a is a constant and b = Δsub;vapH°(〈T〉)/R, eqn (4). |
ln(p/Pa) = a − b × [(1/T)/K−1]
| (4) |
Table 3 Experimental vapor pressures of mCP (liquid), CBP (solid) and TCB (solid) obtained with the quartz crystal microbalance Knudsen effusion methodology
T/K |
p/Pa |
Δp/Pa |
T/K |
p/Pa |
Δp/Pa |
mCP (liquid) |
453.20 |
0.0770 |
−0.0003 |
469.70 |
0.2740 |
0.0014 |
456.20 |
0.0975 |
−0.0003 |
471.20 |
0.3040 |
−0.0003 |
459.20 |
0.1240 |
0.0005 |
472.70 |
0.3420 |
0.0024 |
462.20 |
0.1560 |
0.0006 |
474.20 |
0.3740 |
−0.0046 |
465.20 |
0.1950 |
0.0001 |
475.70 |
0.4260 |
0.0042 |
466.70 |
0.2180 |
−0.0002 |
477.20 |
0.4630 |
−0.0067 |
468.20 |
0.2440 |
0.0001 |
478.80 |
0.5290 |
0.0027 |
![[thin space (1/6-em)]](https://www.rsc.org/images/entities/char_2009.gif) |
CBP (solid) |
523.20 |
0.0519 |
0.0006 |
540.50 |
0.2093 |
0.0003 |
525.55 |
0.0615 |
−0.0009 |
542.40 |
0.2420 |
−0.0005 |
528.30 |
0.0788 |
0.0004 |
543.40 |
0.2630 |
0.0009 |
530.55 |
0.0935 |
−0.0007 |
545.50 |
0.3093 |
0.0009 |
533.33 |
0.1183 |
0.0002 |
546.50 |
0.3340 |
0.0009 |
535.55 |
0.1405 |
−0.0006 |
548.20 |
0.3740 |
−0.0055 |
536.40 |
0.1520 |
0.0009 |
549.50 |
0.4190 |
−0.0001 |
538.30 |
0.1760 |
0.0003 |
550.60 |
0.4580 |
0.0025 |
539.40 |
0.1920 |
0.0004 |
|
|
|
![[thin space (1/6-em)]](https://www.rsc.org/images/entities/char_2009.gif) |
TCB (solid) |
555.70 |
0.0785 |
−0.0004 |
569.50 |
0.2260 |
0.0010 |
558.35 |
0.0965 |
−0.0003 |
570.60 |
0.2440 |
0.0000 |
560.70 |
0.1150 |
−0.0010 |
572.35 |
0.2780 |
0.0004 |
561.50 |
0.1240 |
0.0007 |
573.35 |
0.2990 |
0.0003 |
563.35 |
0.1420 |
0.0001 |
575.60 |
0.3510 |
−0.0009 |
564.50 |
0.1550 |
0.0002 |
576.50 |
0.3760 |
0.0004 |
565.70 |
0.1690 |
−0.0004 |
578.35 |
0.4270 |
−0.0022 |
566.50 |
0.1820 |
0.0021 |
579.50 |
0.4670 |
0.0009 |
567.50 |
0.1940 |
0.0001 |
582.60 |
0.5780 |
−0.0032 |
568.35 |
0.2070 |
0.0004 |
|
|
|
The plots of ln(p) = f (1/T) for mCP, CBP and TCB are shown in Fig. 5. Table 4 lists the parameters of the Clausius–Clapeyron equation (a and b) with associated standard deviations, the effusion temperatures interval and equilibrium vapor pressures (EVP), and the standard molar enthalpies, Δsub;vapH°(〈T〉), entropies, Δsub;vapS°(〈T〉), and Gibbs energies, Δsub;vapG°(〈T〉), of sublimation/vaporization at the mean temperature. Δsub;vapH°(〈T〉) was determined from the parameter b of the eqn (4) and Δsub;vapS°(〈T〉) were calculated using eqn (5). Δsub;vapG°(〈T〉) was derived as {ΔH°(〈T〉) − 〈T〉·ΔS°(〈T〉)}. Heat capacity corrections were made to determine molar enthalpies and entropies of sublimation (at θ = 298.15 K), for solids CBP and TCB. The value of
was used as the difference between the gas and solid phase heat capacity at θ = 298.15 K. The estimation of
was based on previous determinations of solid heat capacities of compounds with similar chemical structure, such as the phenylamines TPB and TPD, whose obtained values of
, are (−17.8 ± 10) and (−20.2 ± 10) J K−1 mol−1, respectively.19
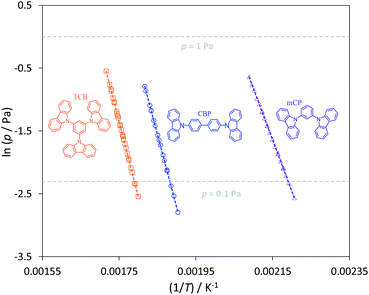 |
| Fig. 5 Plots of ln(p) versus 1/T for mCP (triangles), CBP (circles) and TCB (squares). | |
Table 4 Standard (p° = 105 Pa) thermodynamic properties (values at the mean temperature of the effusion experiments) associated to the liquid–gas equilibrium of mCP and to the solid–gas equilibria of CBP and TCB: effusion temperatures (Teffusion), equilibrium vapor pressures (EVP), and enthalpies (Δsub;vapH°), entropies (Δsub;vapS°) and Gibbs energies (Δsub;vapG°) of phase transition. Parameters a and b were obtained from the integrated form of Clausius–Clapeyron equation
Property |
mCP (l) |
CBP (s) |
TCB (s) |
a |
33.3 ± 0.1 |
40.9 ± 0.1 |
40.7 ± 0.1 |
b |
16 263 ± 54 |
22 954 ± 62 |
24 029 ± 48 |
Teffusion |
454 to 478 |
524 to 550 |
556 to 583 |
EVP |
0.077 to 0.53 |
0.052 to 0.46 |
0.079 to 0.58 |
Δsub;vapH° |
135.2 ± 0.5 |
190.9 ± 0.5 |
199.9 ± 0.4 |
Δsub;vapS° |
181.3 ± 1.0 |
244.4 ± 1.0 |
242.8 ± 0.7 |
Δsub;vapG° |
81.1 ± 0.5 |
118.0 ± 0.6 |
127.5 ± 0.5 |
The value of
obtained as
, was used as the difference between the gas and liquid phase heat capacity at θ = 298.15 K for the liquid mCP. The standard molar enthalpies and entropies of sublimation/vaporization, at the reference temperature of θ = 298.15 K, were determined by eqn (6) and (7). Δsub;vapG°(θ) was derived as {Δsub;vapH°(θ) − θ·Δsub;vapS°(θ)}. Thermodynamic parameters associated with the equilibria processes of fusion, sublimation, and vaporization, at the reference temperature θ, are interconnected by means of eqn (8). Sublimation and vaporization properties, at θ = 298.15 K, are listed in Table 5.
|
 | (5) |
|
 | (6) |
|
 | (7) |
|
Δsub[H°;S°;G°] = Δvap[H°;S°;G°] + Δfus[H°;S°;G°]
| (8) |
Table 5 Standard molar enthalpies (ΔH°), entropies (ΔS°), and Gibbs energies (ΔG°) of sublimation and vaporization, at θ = 298.15 K, for mCP, CBP, and TCB. Literature values for PhC, BDB, TPB, and TDAB are listed for comparison
Material |
ΔH°, kJ mol−1 |
ΔS°, J K−1 mol−1 |
ΔG°, kJ mol−1 |
Data from ref. 39. Data from ref. 68. Data from ref. 19. |
Sublimation |
PhCa |
116.1 ± 1.7 |
211.3 ± 5.0 |
53.1 ± 2.3 |
mCP |
166.5 ± 2.7 |
251.7 ± 6.7 |
91.4 ± 3.4 |
CBP |
195.7 ± 2.5 |
256.2 ± 6.0 |
119.3 ± 3.0 |
TCB |
205.3 ± 2.7 |
255.8 ± 6.5 |
129.0 ± 3.4 |
TPAb |
92.0 ± 2.5 |
|
|
BDBc |
178.8 ± 1.6 |
292.4 ± 4.3 |
91.7 ± 2.0 |
TPBc |
198.5 ± 2.0 |
294.0 ± 5.2 |
110.9 ± 2.5 |
TDABc |
200.8 ± 2.0 |
298.1 ± 5.2 |
111.9 ± 2.5 |
![[thin space (1/6-em)]](https://www.rsc.org/images/entities/char_2009.gif) |
Vaporization |
PhC |
100.2 ± 1.9 |
169.1 ± 5.5 |
49.8 ± 2.5 |
mCP |
147.1 ± 1.8 |
212.9 ± 4.6 |
83.6 ± 2.2 |
CBP |
165.7 ± 4.5 |
209.9 ± 9.5 |
103.1 ± 5.4 |
TCB |
178.5 ± 4.8 |
220.7 ± 10.4 |
112.7 ± 5.7 |
TPA |
72.2 ± 3.2 |
|
|
BDB |
143.6 ± 2.4 |
223.0 ± 6.4 |
77.1 ± 3.0 |
TPB |
162.6 ± 2.9 |
228.8 ± 7.4 |
94.5 ± 3.6 |
TDAB |
150.1 ± 3.1 |
208.6 ± 7.8 |
87.9 ± 3.9 |
The experimental results of Table 3 and Fig. 5 evidence a volatility differentiation between mCP, CBP and TCB. The data for mCP corresponds to the liquid phase; the vapor pressures of solid mCP at the triple point are very low and outside the measuring range of the Knudsen apparatus (0.1 to 1 Pa).50 The higher volatility of CBP, in comparison with TCB, was found to be enthalpically driven (entropies of sublimation are similar) indicating stronger cohesive energies in the crystalline phase of TCB. Comparing to their phenylamine analogues, the solid phase of phenylcarbazoles is less volatile presenting higher stability: CBP is less volatile than TPB; TCB is less volatile than TDAB. These results are highlighted by the higher values of ΔsubG° exhibited by phenylcarbazole derivatives. The values listed in Table 5 are useful to compare the relative stability of the condensed phases of all compounds. Concerning the solid phase, higher cohesive energies of TPA, in comparison with PhC, can be perceived from the magnitude of ΔsubH° values. For the other pairs of compounds (phenylcarbazole vs. phenylamine congener) there is no significant differentiation when comparing the ΔsubH°, as deviations are less than 5 kJ mol−1: 195.7 and 198.5 kJ mol−1 for CBP and TPB, respectively; 205.3 and 200.8 kJ mol−1 for TCB and TDAB, respectively. The experimental data indicate that the differentiation in the molecular structure of phenylcarbazoles and phenylamines does not affect significantly the crystal packing and intermolecular interactions. Hence, the noticeable difference that explains the lower volatility of solid phenylcarbazoles is the entropy of sublimation. Curiously, attending to the experimental uncertainties, the phenylcarbazole derivatives mCP, CBP and TCB have similar values of ΔsubS° (from 251.7 to 256.2 J K−1 mol−1). Comparatively, the solid phase of phenylamines DDP, TPB and TDAB have higher values of ΔsubS°, but also similar between them (from 292.4 to 298.1 J K−1 mol−1). These results are consistent with fusion results indicating a clear entropic differentiation of phenylcarbazoles and phenylamines with respect to their liquid and gas phases. The additional chemical bond between the phenyl groups in the carbazolyl units leads to a less flexible chemical structure with a significant decrease of translational and rotational motions of the rings. The most stable conformations of N-phenylcarbazole and triphenylamine are depicted in Fig. 6. In comparison to phenylamines, the more rigid phenylcarbazole derivatives exhibit lower absolute entropies in liquid and gas phases and thus, lower values of ΔfusS° and ΔsubS°. Fig. 7 systematizes an additive scheme representing the increments in ΔsubH° and ΔsubS° (at the same reference temperature) upon successive introduction of carbazolyl or diphenylamino groups. The enthalpic increments between biphenyl and CBP and between biphenyl and TPB were found to be similar (114.2 and 117.0 kJ mol−1), whereas a large differentiation is found by analysing the ΔsubS° increments (75.9 vs. 113.7 J K−1 mol−1). A comparison between PhC, mCP, and TCB shows that increments in ΔsubH° arising from the successive introductions of carbazolyl moieties decrease due to intramolecular repulsions between the groups. The same effect is observed when analysing the effect of introducing additional diphenylamino groups in TPA. The clear entropic differentiation between phenylcarbazoles and phenylamines is well emphasized by comparing the additive scheme for ΔsubS°: lower values are systematically observed for derivatives containing carbazolyl moieties replacing diphenylamino groups. Concerning the phenylcarbazoles, to better elucidate the effect of incorporating carbazolyl groups in meta position on the sublimation thermodynamic properties of sublimation, Fig. 8 shows the dependence of ΔsubH°, T·ΔsubS°, and ΔsubG° with the number of carbazolyl moieties. A linear dependence is observed for ΔsubH° values indicating a large intensity of intermolecular interactions in the lattice for TCB.
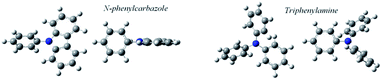 |
| Fig. 6 Views of the B3LYP/6-31+G(d,p)-optimized structures of the minimum energy conformers of N-phenylcarbazole and triphenylamine. | |
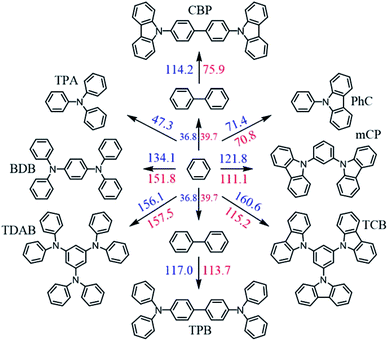 |
| Fig. 7 Additive scheme for ΔsubH°(298.15 K) (blue values, in kJ mol−1) and ΔsubS°(298.15 K) (red values, in J K−1 mol−1), representing the increments in these quantities upon successive introduction of carbazolyl or diphenylamino groups. The errors in the calculated increments are omitted for clarity. ΔsubH° and ΔsubS° values for benzene (44.7 kJ mol−1 and 140.6 J K−1 mol−1) and biphenyl (81.5 and 180.3 J K−1 mol−1) were obtained from literature.69 | |
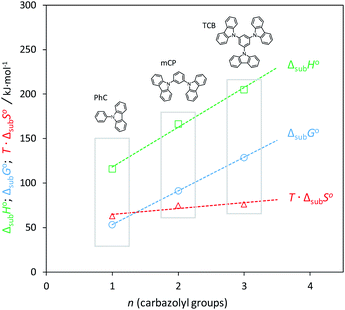 |
| Fig. 8 Dependence of the thermodynamic properties of sublimation with the number of carbazolyl groups: ΔsubH° (squares); T·ΔsubS° (triangles); ΔsubG° (circles). | |
The relative stability of the solid phase of phenylcarbazole derivatives is mostly enthalpically driven and increases linearly with the molar mass (carbazolyl groups). The same conclusion was reached in a recent work for a series of phenylamine derivatives with a different number of diphenylamino groups.21 According to Fig. 8, there is no clear entropic differentiation along with the series and the enthalpy–entropy compensation leads to a highly linear dependence (r2 > 0.9999) observed for ΔsubG° values. The vaporization results, derived from the combination of fusion and sublimation data, were used to evaluate the relative stability of the liquid state. Looking at the sublimation and fusion data, there are clear entropic differentiations between phenylcarbazoles and their corresponding phenylamines. This distinction, however, is not so obvious for ΔvapS° values. Moreover, there is a clear enthalpy–entropy compensation that explains the higher volatility of the liquid phase of phenylamines: when comparing each pair of congener compounds (CBP vs. TPB and TCB vs. TDAB), ΔvapG° is higher for the compounds containing carbazolyl moieties; CBP and TPB display similar values of ΔvapH°; compared to TDAB, TCB is characterized by a higher value ΔvapH° which arises from the larger differentiation in the fusion enthalpies of these compounds. In fact, compared to other molecules, TDAB requires a larger amount of energy to melt (≈62 kJ mol−1 at Tm). The enthalpic and entropic factors associated with differentiation in terms of intermolecular interactions and molecular flexibility can be explored in more detail by inferring the cohesive energies in the liquid phase. As discussed in recent works, a liquid with a significant level of structuration displays a low ratio of ΔfusH°/ΔsubH° as most of the intermolecular interactions are not disrupted even after a melting process.64,70 A similar effect is observed for phenylcarbazoles (Fig. 9).
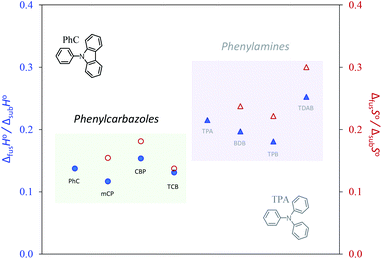 |
| Fig. 9 ΔfusH°/ΔsubH° ratios (blue solid circles and triangles) and ΔfusS°/ΔsubS° ratios (red open circles and triangles) for series of phenylcarbazoles (circles) and phenylamines (triangles). The thermodynamic properties were derived at θ = 298.15 K. | |
In order to rationalize this effect emphasizing the structural differentiation of carbazoles and phenylamines, Fig. 9 represents the magnitude of {ΔfusH°/ΔsubH°} and {ΔfusS°/ΔsubS°} ratios for the studied compounds. Both ratios are clearly lower for phenylcarbazoles. The chemical bond between the phenyl groups in the carbazolyl unit contributes to a substance with a higher level of structuration and organization in the liquid phase as in comparison with phenylamines, molecules are more rigid and available to perform more stable and powerful intermolecular interactions. For instance, ΔfusH°/ΔsubH° ratios of 0.25 and 0.13 were found for TDAB and TCB, respectively; compared to TCB, the high flexibility of TDAB is also expressed by a larger ratio of ΔfusS°/ΔsubS° (0.30 for TDAB vs. 0.14 for TCB). The results clarify that intermolecular bonding disruption along the fusion transition is more extensive for phenylamine derivatives.
Characterization of thin films
Based on volatility studies, thin films of mCP, CBP, and TCB were prepared by physical vapor deposition. A customized procedure of vacuum thermal evaporation has been used. SEM micrographs of vapor-deposited thin films onto quartz crystal surfaces coated with gold (Au), silver (Ag) or aluminium (Al) are presented by Fig. 10. The mass flow rate (φ, ng cm−2 s−1) can be estimated according to eqn (9), in which φsubstrate and φKnudsen cell are the mass flow rates on the substrate surface and from the Knudsen cell orifice, respectively. This equation considers a geometrical factor g that is dependent on the distance between the deposition source (Knudsen cell) and the deposition surface (substrate). For the calculation of φKnudsen cell, T is the evaporation temperature, p is the equilibrium vapor pressure at T, wo is a transmission probability factor, MM is the molar mass of the vapor effused, R is the gas constant, m is the mass of sample evaporated, t is the deposition time, and Ao is the area of the Knudsen cell orifice. |
 | (9) |
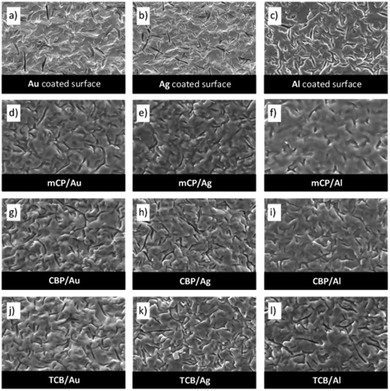 |
| Fig. 10 Topographic images obtained by SEM of the vapor-deposited thin films produced in this work: gold (Au) coated quartz crystal surface (a); silver (Ag) coated quartz crystal surface (b); aluminium (Al) coated quartz crystal surface (c); thin film morphology of mCP deposited on Au (d), Ag (e), and Al (f); thin film morphology of CBP deposited on Au (g), Ag (h), and Al (i); thin film morphology of TCB deposited on Au (j), Ag (k), and Al (l). The thin films were deposited under the same experimental conditions: equilibrium vapor pressure of ≈0.3 Pa; mass flow rate at the substrate surface of ≈22 ng cm−2 s−1; deposition time of 60 minutes; substrate temperature ≈293 K. For these experimental conditions, mCP, CBP, and TCB were evaporated at 472, 545, and 573 K, respectively. | |
The vapor deposition process was accomplished under the same experimental conditions for all compounds; the same equilibrium vapor pressure (≈0.3 Pa) and derived mass flow rate, as well as the deposition time (60 minutes), were applied. A constant mass flow rate on the surface of the substrate (≈22 ng cm−2 s−1) was regulated by the evaporation temperature of each compound. Regarding the morphological analysis (Fig. 10), the irregular topography of the quartz crystals is depicted by images (a)–(c). In all cases, the several cavities and the surface roughness of the quartz crystal have an important role for the nucleation and growth mechanisms as well as to the topography of thin films. There is perceptible differentiation in the topography of the three types of quartz crystals (Au, Ag, Al) used as the grain morphology of the coated metal is dissimilar. The structure and surface morphology of organic materials produced by vapor deposition methods, particularly the vacuum thermal evaporation, is highly dependent on the structural, thermal and morphological properties of the surface.19,21,42,43,56,57,71–74 The morphology of vapor-deposited thin films of mCP, CBP, and TCB does not evidence a significant growth of crystal structures as the images suggest an amorphous appearance, with emphasis on mCP, accompanied by a high dependence with the substrate roughness. Looking at the SEM images, grains' shape and size are not recognized. SEM of an amorphous material doesn't release any features and no particles can be distinguished. These findings agree with a recent work regarding similar systems, upon which XRD was used to confirm the amorphous nature of thin films.21 Strong binding of organic thin films can be perceived and is a typical observation for as-deposited thin organic films growing by PVD methods.21 Predictably, the large coverage and homogeneity of these organic materials indicate a layer-by-layer growth process.75,76 One of the key properties for the amorphous nature of as-deposited films of phenylcarbazoles is the glass transition temperature (Tg). Literature reports indicate values of Tg of ≈330 K, ≈335 K, and ≈400 K for mCP,77,78 CBP,79,80 and TCB,81,82 respectively. As the thin film deposition of this work were performed on surfaces kept at a constant temperature of 293 K, the amorphous nature of materials would be expected. The glassy state is usually observed for nonplanar molecules as the hard packing of molecules avoids the crystallization process. The ratio Tg/Tm = 0.73 (≈3/4) is observed for mCP, which would be expected due to the asymmetric nonplanar structure of this compound.19 Lower values of Tg/Tm (0.61 for CBP and 0.67 for TCB) are typical for a more symmetric nonplanar structure, whose ratio of Tg/Tm is expected to be around 2/3.19 The existence of a glassy state is also a consequence of the less flexibility of phenylcarbazole derivatives in comparison with some phenylamines. For instance, the higher flexibility of TDAB is related to the lower ability to form a glassy state. As discussed in previous reports, despite being nonplanar, due to the high flexibility (suitable ring rotation potential profile) this compound has a strong ability to crystallize (very lower liquid undercooling stability) which avoids the formation of the glassy state. Due to this fact, a vapor-deposited thin film of TDAB is always crystalline.19,42,82
Conclusions
The optical, morphological and thermodynamic behavior were explored for a series of carbazole derivatives (mCP, CBP, and TCB), organic semiconductors with wide use in molecular electronics and exciting liquid organic hydrogen carriers. The structural differentiation between phenylcarbazoles and phenylamine analogues was reflected in the physical chemistry behavior of each class of materials. Regarding the UV-vis characterization, phenylcarbazoles exhibit a blue shift compared to phenylamines, noticeably so as more moieties are present in the molecule, resulting in higher optical band gaps. From the thermodynamic study of phase transitions, it can be concluded that entropic factors are of greater importance for the higher melting points and low volatility exhibited by the solid phase of carbazole derivatives. Analysis of fusion and sublimation data suggests phenylcarbazoles retain higher levels of structuration when compared to their phenylamine counterparts. This trend also seems to persist after the melting point. The additional bond in phenylcarbazoles severely limits the freedom of movement of the end groups, resulting in less flexible structures that also remain partially interactive even after there is greater molecular mobility. From the morphological study of vapor-deposited phenylcarbazole thin films, it can be concluded that these compounds do not show significant crystal growth, as their smooth appearance strongly suggests it is largely amorphous. The experimental data reveals the role of the chemical bond between the phenyl groups in the carbazolyl unit for a more rigid structure leading to the amorphous nature of thin films.
Conflicts of interest
There are no conflicts to declare.
Acknowledgements
The authors thank Fundacão para a Ciência e Tecnologia (FCT), for financial support (funded by national funds through the FCT/MCTES (PIDDAC)) to CIQUP, Faculty of Science, University of Porto (Project UIDB/00/2020), and LEPABE, Faculty of Engineering, University of Porto (Project UIDB/00511/2020). Dr José Costa also thank FCT for the award of the research grant SFRH/BPD/116930/2016.
References
- V. Coropceanu, J. Cornil, D. A. da Silva Filho, Y. Olivier, R. Silbey and J.-L. Brédas, Chem. Rev., 2007, 107, 926–952 CrossRef CAS PubMed.
- N. T. Kalyani and S. J. Dhoble, Renewable Sustainable Energy Rev., 2012, 16, 2696–2723 CrossRef.
- C. Wang, H. Dong, W. Hu, Y. Liu and D. Zhu, Chem. Rev., 2012, 112, 2208–2267 CrossRef CAS PubMed.
- J. C. S. Costa, R. J. S. Taveira, C. F. R. A. C. Lima, A. Mendes and L. M. N. B. F. Santos, Opt. Mater., 2016, 58, 51–60 CrossRef CAS.
- C. Wang, H. Dong, L. Jiang and W. Hu, Chem. Soc. Rev., 2018, 47, 422–500 RSC.
- Y. Shirota, J. Mater. Chem., 2000, 10, 1–25 RSC.
- Y. Shirota, K. Okumoto and H. Inada, Synth. Met., 2000, 111, 387–391 CrossRef.
- Y. Tao, C. Yang and J. Qin, Chem. Soc. Rev., 2011, 40, 2943–2970 RSC.
- J. Melas-Kyriazi, I.-K. Ding, A. Marchioro, A. Punzi, B. Hardin, G. F. Burkhard, N. Tétreault, M. Grätzel, J.-E. Moser and M. D. McGehee, Adv. Energy Mater., 2011, 1, 407–414 CrossRef CAS.
- L. Calió, S. Kazim, M. Grätzel and S. Ahmad, Angew. Chem., 2016, 55, 14522–14545 CrossRef PubMed.
- Y. Shirota, J. Mater. Chem., 2005, 15, 75–93 RSC.
- T. Leijtens, I.-K. Ding, T. Giovenzana, J. T. Bloking, M. D. McGehee and A. Sellinger, ACS Nano, 2012, 62, 1455–1462 CrossRef PubMed.
- M. Saliba, S. Orlandi, T. Matsui, S. Aghazada, M. Cavazzini, J.-P. Correa-Baena, P. Gao, R. Scopelliti, E. Mosconi, K.-H. Dahmen, F. De Angelis, A. Abate, A. Hagfeldt, G. Pozzi, M. Grätzel and M. K. Nazeeruddin, Nat. Energy, 2016, 1, 15017 CrossRef CAS.
- Y. Kuwabara, H. Ogawa, H. Inada, N. Noma and Y. Shirota, Adv. Mater., 1994, 6, 677–679 CrossRef CAS.
- P. Agarwala and D. Kabra, J. Mater. Chem. A, 2017, 5, 1348–1373 RSC.
- R. Braveenth, H. Woo Bae, I. Jang Ko, W. Qiong, Q. P. B. Nguyen, P. G. S. Jayashantha, J. H. Kwon and K. Y. Chai, Org. Electron., 2017, 51, 463–470 CrossRef CAS.
- X. Liu, X. Shi, C. Liu, Y. Ren, Y. Wu, W. Yang, A. Alsaedi, T. Hayat, F. Kong, X. Liu, Y. Ding, J. Yao and S. Dai, J. Phys. Chem. C, 2018, 122, 26337–26343 CrossRef CAS.
- G. Krucaite, D. Volyniuk, J. Simokaitiene, S. Grigalevicius, C.-H. Lin, C.-M. Shao and C.-H. Chang, Dyes Pigm., 2019, 162, 196–202 CrossRef CAS.
- J. C. S. Costa and L. M. N. B. F. Santos, J. Phys. Chem. C, 2013, 117, 10919–10928 CrossRef CAS.
- K.-H. Lin, A. Prlj and C. Corminboeuf, J. Mater. Chem. C, 2018, 6, 960–965 RSC.
- J. C. S. Costa, A. Mendes and L. M. N. B. F. Santos, J. Mater. Sci., 2018, 53, 12974–12987 CrossRef CAS.
- R. F. Pineda, J. Troughton, M. Planells, I. S.-M. Santos, F. Muhith, G. S. Nichol, S. Haque, T. Watson and N. Robertson, Phys. Chem. Chem. Phys., 2018, 20, 1252–1260 RSC.
- Y. Li, Y. Zhang, P. Heng, R. Shao, Y. Liu, W. Qiao, L. Wang and J. Zhang, Org. Electron., 2018, 54, 14–20 CrossRef CAS.
- S. Oner, M. Aydemir, F. Yesil, C. Sahin and C. Varlikli, Dyes Pigm., 2018, 159, 92–99 CrossRef CAS.
- C. Yin, J. Lu, Y. Xu, Y. Yun, K. Wang, J. Li, L. Jiang, J. Sun, A. D. Scully, F. Huang, J. Zhong, J. Wang, Y.-B. Cheng, T. Qin and W. Huang, Adv. Energy Mater., 2018, 8, 1800538 CrossRef.
- A. Magomedov, S. Paek, P. Gratia, E. Kasparavicius, M. Daskeviciene, E. Kamarauskas, A. Gruodis, V. Jankauskas, K. Kantminiene, K. T. Cho, K. Rakstys, T. Malinauskas, V. Getautis and M. K. Nazeeruddin, Adv. Funct. Mater., 2018, 28, 1704351 CrossRef.
- X. Liu, X. Ding, Y. Ren, Y. Yang, Y. Ding, X. Liu, A. Alsaedi, T. Hayat, J. Yao and S. Dai, J. Mater. Chem. C, 2018, 6, 12912–12918 RSC.
- X.-D. Zhu, X.-J. Ma, Y.-K. Wang, Y. Li, C.-H. Gao, Z.-K. Wang, Z.-Q. Jiang and L.-S. Liao, Adv. Funct. Mater., 2019, 29, 1807094 Search PubMed.
- S. H. Kim and J. Jang, Appl. Phys. Lett., 2007, 90, 223505 CrossRef.
- M. Hu, Q. Xu, Y. Jiang, H. Mu, L. Gao, P. Hu, J. Huang and J. Su, Dyes Pigm., 2018, 150, 185–192 CrossRef CAS.
- S.-R. Park, S.-M. Kim, Y. Choi, J. Y. Lee, J.-H. Lee and M. C. Suh, Dyes Pigm., 2019, 170, 107621 CrossRef CAS.
- S. Gong, X. He, Y. Chen, Z. Jiang, C. Zhong, D. Ma, J. Qin and C. Yang, J. Mater. Chem., 2012, 22, 2894–2899 RSC.
- M.-S. Gong, J.-R. Cha and C. W. Lee, Org. Electron., 2017, 42, 66–74 CrossRef CAS.
- C. Papp, P. Wassercheid, J. Libuda and H.-P. Steinruck, Chem. Rev., 2014, 14, 879–896 CAS.
- K. Stark, V. N. Emel'yanenko, A. A. Zhabina, M. A. Varfolomeev, S. P. Verevkin, K. Muller and W. Arlt, Ind. Eng. Chem. Res., 2015, 54, 7953–7966 CrossRef CAS.
- V. N. Emel'yanenko, M. A. Varfolomeev, S. P. Verevkin, K. Stark, K. Muller, M. Muller, A. Bosmann, P. Wasserscheid and W. Arlt, J. Phys. Chem. C, 2015, 119, 26381–26389 CrossRef.
- K. Stark, P. Keil, S. Schug, K. Muller, P. Wasserscheid and W. Arlt, J. Chem. Eng. Data, 2016, 61, 1441–1448 CrossRef CAS.
- I. Y. Choi, B. S. Shin, S. K. Kwak, K. S. Kang, C. W. Yoon and J. W. Kang, Int. J. Hydrogen Energy, 2016, 41, 9367–9373 CrossRef CAS.
- V. N. Emel'yanenko, D. H. Zaitsau, A. A. Pimerzin and S. P. Verevkin, J. Chem. Thermodyn., 2019, 132, 122–128 CrossRef.
- K. R. Jiang, Thin Solid Films, 1990, 191, 91–126 CrossRef.
- S. M. Rossnagel, J. Vac. Sci. Technol., A, 2003, 21, S74 CrossRef CAS.
- J. C. S. Costa, R. M. Rocha, I. C. M. Vaz, M. C. Torres, A. Mendes and L. M. N. B. F. Santos, J. Chem. Eng. Data, 2015, 60, 3776–3791 CrossRef CAS.
- J. C. S. Costa, J. Azevedo, L. M. N. B. F. Santos and A. Mendes, J. Phys. Chem. C, 2017, 121, 2080–2087 CrossRef CAS.
- J. Oh, R. Zhang, P. P. Shetty, J. A. Krogstad, P. V. Braun and N. Miljkovic, Adv. Funct. Mater., 2018, 28, 1707000 CrossRef.
- M. Kurahashi, M. Fukuyo and A. Shimada, Bull. Chem. Soc. Jpn., 1969, 42, 2174–2179 CrossRef CAS.
- A. N. Sobolev, V. K. Belsky, I. P. Romm, N. Y. Chernikova and E. N. Guryanova, Acta Crystallogr., 1985, C41, 967–971 CAS.
- M. E. Wieser, N. Holden, T. B. Coplen, J. K. Bohlke, M. Berglund, W. A. Brand, P. De Bièvre, M. Groning, R. D. Loss, J. Meija, T. Hirata, T. Prohaska, R. Schoenberg, G. O'Connor, T. Walczyk, S. Yoneda and X. K. Zhu, Pure Appl. Chem., 2013, 85, 1047–1078 CAS.
- R. Sabbah, A. Xu-wu, J. S. Chickos, M. L. Planas Leitão, M. V. Roux and L. A. Torres, Reference Materials for Calorimetry and Differential Thermal Analysis, Thermochim. Acta, 1999, 331, 93–204 CrossRef CAS.
- M. V. Roux, M. Temprado, J. S. Chickos and Y. Nagano, J. Phys. Chem. Ref. Data, 2008, 37, 1855–1996 CrossRef CAS.
- L. M. N. B. F. Santos, L. M. S. S. Lima, C. F. R. A. C. Lima, F. D. Magalhães, M. C. Torres, B. Schröder and M. A. V. Ribeiro da Silva, J. Chem. Thermodyn., 2011, 43, 834–843 CrossRef CAS.
- L. M. N. B. F. Santos, A. I. M. C. Lobo Ferreira, V. Stejfa, A. S. M. C. Rodrigues, M. A. A. Rocha, M. C. Torres, F. M. S. Tavares and F. S. Carpinteiro, J. Chem. Thermodyn., 2018, 126, 171–186 CrossRef CAS.
- M. A. V. Ribeiro da Silva, M. J. S. Monte and L. M. N. B. F. Santos, J. Chem. Thermodyn., 2006, 38, 778–787 CrossRef CAS.
- S. P. Verevkin, J. Chem. Thermodyn., 1997, 29, 1495–1501 CrossRef CAS.
- L. Malaspina, G. Bardi and R. Gigli, J. Chem. Thermodyn., 1974, 6, 1053–1064 CrossRef CAS.
- N. Wakayama and H. Inokuchi, Bull. Chem. Soc. Jpn., 1967, 40, 2267–2271 CrossRef CAS.
- J. C. S. Costa, A. F. S. M. G. Coelho, A. Mendes and L. M. N. B. F. Santos, Appl. Surf. Sci., 2018, 428, 242–249 CrossRef CAS.
- J. C. S. Costa, J. Azevedo, J. P. Araújo, L. M. N. B. F. Santos and A. Mendes, Thin Solid Films, 2018, 664, 12–18 CrossRef CAS.
- J. Sworakowski, Synth. Met., 2018, 235, 125–130 CrossRef CAS.
- S. B. Suryawanshi, P. G. Mahajan, A. J. Bodake, G. B. Kolekar and S. R. Patil, Spectrochim. Acta, Part A, 2017, 183, 232–238 CrossRef CAS PubMed.
- L.-S. Cui, S.-C. Dong, Y. Liu, Q. Li, Z.-Q. Jiang and L.-S. Liao, J. Mater. Chem. C, 2013, 1, 3967–3975 RSC.
- K. L. Woon, A. Ariffin, K. W. Ho and S.-A. Chen, RSC Adv., 2018, 8, 9850–9857 RSC.
- I. A. Wright, H. A. Al-Attar, A. S. Batsanov, A. P. Monkman and M. R. Bryce, Phys. Chem. Chem. Phys., 2018, 20, 11867–11875 RSC.
- R. K. Konidena, K. H. Lee and J. Y. Lee, Chem. Commun., 2019, 55, 8178–8181 RSC.
- J. C. S. Costa and L. M. N. B. F. Santos, J. Chem. Eng. Data, 2019, 64, 2229–2246 CrossRef CAS.
- J. Chickos and D. Lipkind, J. Chem. Eng. Data, 2008, 53, 2432–2440 CrossRef CAS.
- J. S. Chickos, Thermochim. Acta, 1998, 313, 19–26 CrossRef CAS.
- W. E. Acree, Thermochim. Acta, 1993, 219, 97–104 CrossRef.
- W. V. Steele, J. Chem. Thermodyn., 1978, 10, 441–444 CrossRef CAS.
- C. F. R. A. C. Lima, M. A. A. Rocha, A. Melo, L. R. Gomes, J. N. Low and L. M. N. B. F. Santos, J. Phys. Chem. A, 2011, 115, 11876–11888 CrossRef CAS PubMed.
- J. C. S. Costa, A. Mendes and L. M. N. B. F. Santos, J. Chem. Eng. Data, 2018, 63, 1–20 CrossRef CAS.
- Z. Luo, Y. Lu, D. W. Singer, M. E. Berck, L. A. Somers, B. R. Goldsmith and A. T. C. Johnson, Chem. Mater., 2011, 23, 1441–1447 CrossRef CAS.
- J. Munemasa and T. Kumakiri, Surf. Coat. Technol., 1991, 49, 496–499 CrossRef CAS.
- M. Nisha, S. Anusha, A. Antony, R. Manoj and M. K. Jayaraj, Appl. Surf. Sci., 2005, 252, 1430–1435 CrossRef CAS.
- A. A. Virkar, S. Mannsfeld, Z. Bao and N. Stingelin, Adv. Mater., 2010, 22, 3857–3875 CrossRef CAS PubMed.
- J. A. Venables, G. D. T. Spiller and M. Hanbucken, Rep. Prog. Phys., 1984, 47, 399–459 CrossRef.
- Z. Zhang and M. G. Lagally, Science, 1997, 276, 377–383 CrossRef CAS PubMed.
- M.-S. Gong, J.-R. Cha and C. W. Lee, Org. Electron., 2017, 42, 66–74 CrossRef CAS.
- D. Sun, Z. Yang, Z. Ren, H. Li, M. R. Bryce, D. Ma and S. Yan, Chem.–Eur. J., 2014, 20, 16233–16241 CrossRef CAS PubMed.
- D. Hu, P. Lu, C. Wang, H. Liu, H. Wang, Z. Wang, T. Fei, X. Gu and Y. Ma, J. Mater. Chem., 2009, 19, 6143–6148 RSC.
- T. Zhang, Y. Liang, J. Cheng and J. Li, J. Mater. Chem. C, 2013, 1, 757–764 RSC.
- W. Jiang, Z. Ge, P. Cai, B. Huang, Y. Dai, Y. Sun, J. Qiao, L. Wang, L. Duan and Y. Qiu, J. Mater. Chem., 2012, 22, 12016–12022 RSC.
- Y. Shirota, J. Mater. Chem., 2000, 10, 1–25 RSC.
Footnote |
† Electronic supplementary information (ESI) available: Detailed UV-vis absorption spectra, DSC calibration results, experimental tests for vapor pressures determination, and enlarged SEM images. See DOI: 10.1039/d0ra01518k |
|
This journal is © The Royal Society of Chemistry 2020 |
Click here to see how this site uses Cookies. View our privacy policy here.