DOI:
10.1039/C4BM00212A
(Paper)
Biomater. Sci., 2014,
2, 1740-1749
Sub-100 nm patterning of TiO2 film for the regulation of endothelial and smooth muscle cell functions†
Received
11th June 2014
, Accepted 8th July 2014
First published on 25th July 2014
Abstract
Previous studies have shown that fundamental cell functions such as adhesion, proliferation, and morphology are regulated by the interaction of cells with basement membrane nano- and micron- scale surface topography. By taking the basement membrane as a guiding principle, the surface can be designed with biophysical cues in the form of surface topographies to modulate the cellular functions of vascular endothelial and smooth muscle cells, which are crucial for vascular diseases. Titanium-based materials whose surfaces are covered by a thin layer of titanium dioxide (TiO2) are utilized in several regenerative medicine applications such as vascular prosthetics. The utilization of TiO2-covered materials makes it essential to understand the interaction of cells with the TiO2 layer to control the cell response. While it has been shown by means of patterned micro- and nano-topography that it is important to regulate cell functions on non-metallic materials, it would be of interest in the field of regenerative medicine to study the cell response on patterned TiO2 surface layers. Previous studies have mostly focused on studying the cell response on random micro- and nano-roughened metallic and metal oxide surfaces as it is challenging to fabricate patterned TiO2 surface layers. Here, the biocompatibility of a method that is capable of the rational patterning of a continuous TiO2 surface layer of sub-100 nm resolution scale using thermally curable resin-based nanoimprinting was studied. The responses of human umbilical vein endothelial cell (HUVEC), such as proliferation, morphology and focal adhesions, and smooth muscle cell (SMC) proliferation and morphology to the nano-patterned TiO2 layer were investigated. Overall, the TiO2 layer with surface nano-gratings showed enhanced proliferation of HUVECs, while it significantly lowered the proliferation of SMCs as compared to the unpatterned control. The HUVECs and SMCs were shown by topography to be sensitive to the 70 nm gratings as evident by the regulation of proliferation and cell shape. A significantly lower focal adhesion density was found of HUVECs on TiO2 nano-gratings, while a significantly higher average focal adhesion size of HUVECs was seen on TiO2 nano-wells and nano-gratings, compared to the unpatterned controls.
1. Introduction
Endothelial cells reside on the basement membrane providing biophysical cues in the form of nano- and micro-topography for the modulation of cell functions.1 Biophysical cues such as surface patterning are important paradigms for the control of cell functions through cell–surface interactions in the design of improved substrates that interact with these cells.2 Several clinical interventions such as the implantation of vascular stents,3 and heart valves4 involve direct cell–surface interactions so the biophysical control of the cell's behaviour will also be beneficial to the treatment of vascular diseases.
Studies of vascular endothelial and smooth muscle cell responses on well-designed and lithographically micro- and nano-patterned polydimethylsiloxane (PDMS) surfaces have shown that these surfaces are able to regulate cell alignment, proliferation, morphology and adhesion,5–8 thus demonstrating the significance of topographical regulation on vascular cell behavior. Metallic and metal oxide material surface modification with random micro- and nano-structured biophysical cues has also been an important research paradigm for the modulation of cell functions.9–13 However, previous studies have mostly focused on micro- and nano-roughened metallic surfaces produced via grit blasting, chemical etching, chemical vapor deposition (CVD), and physical vapor deposition (PVD) methods,14 which are limited to the production of random surface roughness due to the intrinsic nature of the fabrication route. On the other hand, spatially arranged micron and sub-micron titanium features have been demonstrated to modulate vascular cell behavior.15,16 Among the various choices of metallic materials, titanium-based alloys are the promising candidates for vascular intervention applications because of their excellent corrosion resistance, biocompatibility and mechanical properties17–19 where corrosion resistance and biocompatibility mainly results from the TiO2 passivation layer.20–22 A thin layer of TiO2 has also been shown to improve blood compatibility when coated on stainless steel.23 There has been very limited research on cell–topography interaction studies with rationally designed nano-patterned TiO2 surface layers. The patterning resolution is limited as previous studies used mask-based lithography and micromachining techniques to fabricate the oxide surface features. In vitro studies using ordered micro- or nanopatterns on TiO2 layers for controlling the endothelial and smooth muscle cell responses are essential for a fundamental understanding of cell behaviour for vascular diseases applications, but they are currently hampered by the technological challenges of lithographic patterning of rationally designed features on clinically relevant metal oxide layers. Thus, developing effective biocompatible methods for making nano-patterned metal oxide surfaces with a well-defined feature size has become crucial for the improvement of current clinical interventions that utilize cell–surface interactions.
We have previously demonstrated the fabrication of very high-resolution nanostructures on TiO2 layers using thermally-polymerizable24 and photo-polymerizable25 titanium dioxide liquid precursors and nano-imprint lithography. Although the method is versatile with the capability of high-resolution patterning, the biocompatibility of the TiO2 layer obtained from thermally-polymerizable TiO2 resin has not been demonstrated. The primary goal of this study was to analyze the biocompatibility and cytotoxicity of the TiO2 surface layer produced via heat-treatment of thermally-polymerizable TiO2 resin and to evaluate the effect of two different nano-topographies on the proliferation, morphology, and focal adhesions of vascular endothelial cells. As it is essential to reduce the proliferation of smooth muscle cells13 for effective endothelialization and wound healing, we also studied and compared the smooth muscle cell proliferation and morphology on the nano-patterned TiO2 surface layer. For biological experiments, TiO2 samples (patterned and unpatterned) were prepared on glass cover slips acting as the substrates, by a simple spin coating method followed by imprinting and heat treatment steps, to facilitate microscopic examination and biological assay, while the cells exclusively interacted with the TiO2 surface. The same method of preparation of the TiO2 surface layer can also be applied in the preparation of other types of substrates (e.g., Ti, Ni–Ti alloy, etc.).
2. Experimental section
2.1. Fabrication and characterization of nano-patterned TiO2
All the precursors were purchased from Sigma Aldrich. Titanium methacrylate solution was prepared by mixing titanium n-butoxide, and methacrylic acid (MAA) in a molar ratio of 1
:
4 to replace all of the n-butoxy groups. The reaction yielded a yellow solution. To this ethylene glycol dimethacrylate (EDMA), a cross-linker, was added to form TiO2 resin. Finally, 2 wt% of azobisisobutyronitrile (AIBN), a thermal initiator, was added to the resin to facilitate in situ free radical polymerization during imprint lithography. Imprinting of TiO2 resulted in the formation of a polymer network, which not only trapped the metal atom but also increased the strength of the imprinted structures. Although the TiO2 surface layer can also be fabricated on substrates other than glass, glass was chosen as a substrate in the current study to fabricate a surface layer of TiO2 because of its transparency, which enables high resolution microscopy imaging. Prior to nano-imprinting, the glass substrates and Si molds were cleaned with piranha solution at 140 °C for 2 h, followed by rinsing with deionized water and blow-drying using a nitrogen gun. The glass substrates were placed in a drying oven to remove any remaining moisture on the surface. The Si molds were treated with 100 μL of perfluorodecyl-trichlorosilane for 15 min at 80 °C to reduce their surface energy in order to facilitate a clean demolding after nano-imprinting. The dimensions of the substrates used were 2 cm × 2 cm. The types of molds used were 250 nm gratings and wells, both of which had an aspect ratio of 1. TiO2 resin was spin coated onto the glass substrate at a speed of 3000 rpm for 30 s, which resulted in a film of 500 nm thickness. The Si mold, of a smaller size, was placed on top of the film. A 2-step nano-imprinting process was carried out in an Obducat imprinter (Obducat, Sweden). At first, a temperature of 30 °C and a pressure of 10 bars was applied for 5 min to allow the resist to fill the structures of the mold followed by elevating the temperature to 110 °C for 5 min to achieve in situ free radical polymerization of the resin. Samples were cooled to room temperature before the pressure was released. Subsequently, the demolding step was carried out. The yield was found to be ∼100%. Finally, the imprinted patterns were heat treated at a temperature of 450 °C for 1 h in air to remove the organic components. This resulted in an array of TiO2 nano-gratings and nano-wells. To acquire the images of TiO2 patterns, a JEOL JSM-6700F field-emission scanning electron microscope (FE-SEM) was used. A schematic diagram of the TiO2 patterning is shown in Fig. S1.†
2.2. Endothelial and smooth muscle cell culture
Human umbilical vein endothelial cells (HUVECs) were obtained from Lonza and cultured in Endothelial Cell Growth Medium (EGM-2, Lonza). Human coronary artery smooth muscle cells (SMCs) were obtained from Promocell and cultured in Smooth Muscle Cell Growth Medium-2 with SupplementMix (Promocell). Both cell types were cultured under standard cell culture conditions (37 °C, humidified, 5% CO2/95% O2 environment) and the media was changed every other day. Cells were passaged at 80–90% confluence by using trypsin (Gibco) and seeded on samples for experiments. Endothelial and smooth muscle cells for all experiments were used between passages 3–5.
2.3. Cytotoxicity analysis of nano-patterned TiO2 substrates
The cytotoxicity of the TiO2 substrates was analyzed by using AlamarBlue and live/dead assays. For the AlamarBlue assay, the HUVECs were seeded at a seeding density of 2000 cells cm−2 on glass cover slips, heat-treated, and non-heat-treated TiO2 samples were placed in 12 well plates and cultured in Endothelial Cell Growth Medium (EGM-2MV, Lonza). At day 3 and day 5, samples were transferred to 12 new well plates followed by the addition of 10% AlamarBlue (Life Technologies) in culture medium and incubation for 4 h. The redox reaction, in which AlamarBlue is reduced by the cells, was measured by absorbance readings at 540 and 630 nm and the percentage reduction of the AlamarBlue dye was calculated according to the formula provided by the vendor. A higher percentage reduction implies a higher number and viability of cells. For the live/dead assay at day 7, endothelial cells were incubated for 45 min with 3.3 mM of calcein AM and 1.7 mM of ethidium homodimer-1 (Life Technologies). After incubation, the cells were analyzed using a fluorescence microscope (Leica DM IRB) and the images were processed using Image J (NIH).
2.4. Endothelial cell proliferation assay and von Willebrand factor staining and scanning electron microscopy imaging
For assessment of cell proliferation, the HUVECs were seeded on cover slips, and unpatterned and patterned TiO2 substrates at a seeding density of 3000 cell cm−2 were placed in 12 well plates and cultured for 24 h in Endothelial Cell Growth Medium (EGM-2MV, Lonza). After 24 h, the cell proliferation was detected using an Alexa Fluor 488 Click-iT EdU cell proliferation assay kit (Invitrogen). Briefly, the cells were incubated with 10 μM 5-ethynyl-2′-deoxyuridine (EdU) for 4 h. Subsequently, the cells were fixed with 4% paraformaldehyde in 1× PBS, permeabilized in 0.1% Triton-X in 1× PBS, and stained with EdU dye according to the kit's protocol. The Click-iT EdU Cell Proliferation assay also allows the co-staining of other antibodies with EdU dye. After staining with EdU, cells were washed twice with 1× phosphate buffered saline (PBS) and blocked with 10% goat serum for 1 h. After blocking, the cells were incubated with mouse IgG1 anti-von Willebrand factor (vWF) antibody (Santa Cruz) for 1 h at room temperature at a dilution factor of 1
:
300. The samples were then incubated with 2.67 μg ml−1 of Alexa Fluor 546®-conjugated goat anti-mouse antibody (Invitrogen) for 1 h at room temperature and counterstained with 2 ng ml−1 of 4′,6-diamidino-2-phenylindole (DAPI, Sigma-Aldrich) for 30 min at room temperature. EdU incorporation in the proliferating cells was determined by fluorescent microscopy. Primary antibodies were diluted in 1% goat serum in 1× PBS while secondary antibodies were diluted in 1× PBS.
For scanning electron microscopy imaging, the HUVECs were cultured on TiO2 samples for 24 h as mentioned above. Subsequently the cells were fixed with 4% paraformaldehyde and 1% glutaraldehyde (Sigma Aldrich) in 0.1 M sodium cacodylate and 3 mM CaCl2 buffer, serially dehydrated in ethanol gradient and critical point dried (Blazer CPD 030). The samples were sputter-coated with 20 nm of platinum coating before imaging by FE-SEM (JSM-6700F).
2.5. Focal adhesion kinase staining and quantitative characterization
For visualization of vascular endothelial cell focal adhesions (FA), HUVECs were seeded on cover slips, unpatterned and patterned TiO2 substrates at a seeding density of 5000 cell cm−2 were placed in 12 well plates and cultured for 24 h in Endothelial Cell Growth Medium (EGM-2MV). After 24 h the cells were fixed, permeabilized, and blocked as described in section 2.4, and stained with rabbit anti-phosphorylated Y397 FAK primary antibody (Abcam) at a dilution factor of 1
:
100, followed by the addition of Alexa Fluor 488®-conjugated goat anti-rabbit IgG antibody (Invitrogen), with nuclei and F-actin counter-stained. For the quantification of FAs, the local contrast of each image was first enhanced using the CLAHE algorithm.26 The images were then converted to binary mode. The “analyze particles” tool in ImageJ (NIH) was used to find the density, elongation and average area of the FA. FA density is defined as the number of focal adhesions per cell. At least 50 cells from three different samples were analyzed for FA size, elongation and density quantification.
2.6. Smooth muscle cell proliferation and cell shape analysis
For the analysis of SMC proliferation and cell shape, SMCs cells were seeded on cover slips, unpatterned and patterned TiO2 substrates at a seeding density of 3000 cell cm−2 were placed in 12 well plates and cultured for 24 h in Smooth Muscle Cell Growth Medium-2 with SupplementMix (Promocell). After 24 h, SMC proliferation was detected by using the Alexa Fluor® 488 Click-iT® EdU Cell Proliferation assay kit. Briefly, the cells were incubated with 10 μM EdU for 4 h. The cells were then fixed, permeabilized, and stained with EdU dye. After staining with EdU, the cells were washed and stained with mouse anti beta-actin primary antibody (Abcam) with 1
:
500 dilution in 1× PBS followed by staining with 2.67 μg ml−1 Alexa Fluor 546®-conjugated goat anti-mouse IgG antibody (Invitrogen) for 1 h. Finally the cells were stained with 2 ng ml−1 of DAPI for 30 min.
2.7. Endothelial cell morphometric analysis
To investigate the effect of nano-topography on endothelial cell morphology, F-actin stained cells were used to analyze the cell aspect ratio. ImageJ (NIH) was used to calculate the aspect ratio of each cell. The aspect ratio is defined as the ratio between the length and breadth of each cell. At least 50 cells from three different samples were analyzed to measure the cell aspect ratio.
2.8. Statistical analysis
Data are presented as mean ± SD. Student's t-test and one-way ANOVA followed by Tukey's test were used to evaluate the statistical significance where indicated. Statistical analysis was performed with quadruplicates in all experiments.
3. Results and discussion
3.1. Characterization of nano-patterned TiO2 substrates
Heat treatment was carried out to remove the organic components and to convert the imprinted TiO2 resin into a continuous TiO2 surface layer with nano-patterns on top. Fig. 1(a) shows the SEM images of TiO2 nano-gratings of 70 nm width after heat-treatment at 450 °C for 1 h in air. The height of the gratings was also 70 nm as measured by using FE-SEM cross sectional images. Similar heat-treatment yielded 420 nm wide square-wells with a wall thickness and height of 70 nm [Fig. 1(b)]. The total feature size reduction due to loss of organics was ∼70%. Although there was a pattern size reduction, the periodicity and fidelity was still kept the same as the original Si master mold. Pattern size reduction allows the fabrication of sub-100 nm TiO2 nano-structures. Moreover, this method of patterning is useful for biological studies of oxide materials in this size range, which were previously challenging to fabricate using other lithography methods. An XRD study of the heat-treated TiO2 layer showed that the anatase phase appears at 450 °C [Fig. 1(c)]. The diffraction peaks (101), (004) and (200) of the heat-treated sample were indexed as the anatase phase of TiO2 and were found to be in agreement with those detailed in JCPDS Card no. 89-4921. The three crucial parameters for successful imprinting of TiO2 nano-structures are spin-coating speed, heat-treatment time and temperature. Optimizing these parameters appears to be crucial not only for achieving good imprint quality and yield but also for the integrity of the TiO2 structures after heat-treatment. The spin-coating speed determines the film thickness to be imprinted while the heat-treatment time and temperature determine the grain growth and phase of the imprinted TiO2. It was found that a residual layer thickness of slightly less than 400 nm was ideal for achieving TiO2 lines of good integrity after heat-treatment.27
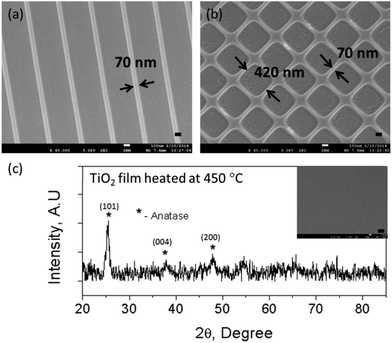 |
| Fig. 1 FE-SEM images of (a) 70 nm TiO2 gratings and (b) 420 nm TiO2 square wells with a wall thickness of 70 nm. (c) XRD of the heat treated film at 450 °C (inset shows an SEM image of the blank film). The feature height is 70 nm (scale bar = 100 nm). | |
Our method has several advantages for nano-imprinting of TiO2. Firstly, the in situ free radical polymerization of TiO2 resin during imprinting not only rigidly shapes the imprint, but also traps the metal atoms. Furthermore, polymerization reduces the surface energy and strengthens the imprinted structures, which results in ∼100% yield after demolding. Secondly, the use of conventional silicon moulds in conjunction with liquid metal methacrylate resin results in the achievement of high resolution imprinting over large areas, which is difficult to achieve using other methods such as etching of TiO2 films. More importantly, by using this method for the fabrication of TiO2 nano-structures, periodic sub-100 nm resolution structures can be achieved due to the size reduction of the features during heat treatment. To exclude the possibility of serum proteins covering the nano-topography, the integrity of the nano-features was confirmed by incubating the samples in culture media overnight followed by FE-SEM analysis. The incubation of samples in culture media did not affect the surface topography (Fig. S1†).
3.2. Cytotoxicity analysis of nano-patterned TiO2 substrates
Heat treatment was performed to remove the organic compounds, which could be toxic to most cells. An unpatterned heat-treated TiO2 layer, which was imprinted with a pristine Si mold without any topographical pattern, was studied for cytotoxicity. Fig. 2 shows phase contrast microscopy images of vascular endothelial cells, showing the cell growth on the cover slip control, heat-treated (HT) TiO2 and non-heat-treated (non-HT) TiO2 (i.e., thermally polymerized TiO2 resin). The images were taken at 2 h after seeding, on day 3, andon day 5. As can be seen from Fig. 2, the cell growth on HT-TiO2 was on a par with that of the cover slip control. Initial cell attachment was observed on the non-HT TiO2 samples after 2 h of seeding. By day 3, very few cells were observed on non-HT-TiO2 samples and essentially no cells were observed by day 5. The cell viability on the cover slip, non-HT TiO2 and HT-TiO2 was measured by AlamarBlue assay on day 3 and day 5 in culture [Fig. 3(a)]. The cell viability was found to be slightly higher on HT-TiO2 compared to that on the glass cover slip control, which implied that the HT-TiO2 is not cytotoxic and confirmed the results obtained by phase contrast microscopy. As expected, the cells did not show any appreciable growth on the non-HT TiO2 samples. The cytotoxicity of the heat-treated TiO2 resist was also evaluated by using the live/dead assay with a cover slip as the control. As shown in Fig. 3(b, c), on day 7 in culture, HT-TiO2 showed cell viability, which was comparable to the cover slip control. Hardly any cells were found on the non-HT TiO2 samples by day 7 (data not shown). Taken together, these results indicate that the TiO2 used in this study is non-toxic to cells and it can be used to evaluate the effect of patterned TiO2 nano-topography on cellular responses.
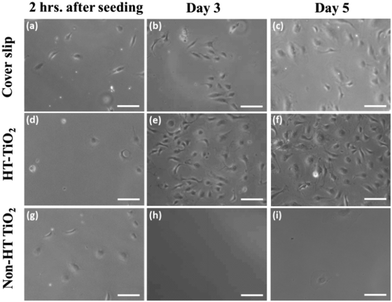 |
| Fig. 2 Phase contrast microscopy images of HUVEC growth on (a–c) cover slip control, (d–f) unpatterned heat-treated TiO2 and (g–i) non-heat-treated TiO2. The images were taken 2 h after seeding, on day 3, and on day 5 (scale bar = 100 μm). | |
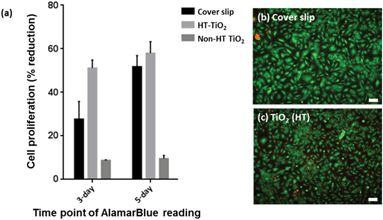 |
| Fig. 3 HUVEC viability as measured by (a) AlamarBlue assay on day 3 and day 5 on a glass cover slip, heat-treated TiO2 (HT-TiO2) and non-heat-treated TiO2 (non-HT TiO2) by incubating cells with AlamarBlue dye for 4 h. Live/dead assay carried out on day 7 on (b) a cover slip control and (c) heat-treated TiO2. The green colour shows the viable cells while the red shows the dead cells (scale bar = 100 μm). | |
3.3. Functional marker expression of HUVECs on nano-patterned TiO2
vWF is a functional marker for vascular endothelial cells. HUVECs were stained for vWF to confirm that the vascular endothelial cells were not de-differentiated into a fibroblastic-like phenotype and that the cells showed vascular endothelial-specific functional marker after growing on patterned HT-TiO2. As shown in Fig. 4, vWF was well expressed by the HUVECs on the cover slip control, unpatterned TiO2, TiO2 nano-wells, and TiO2 nano-gratings. The results suggest that the HUVECs were not de-differentiated on TiO2 substrates, and the substrates did not alter the functional characteristics of the endothelial cells. Moreover, the vascular endothelial cells on the TiO2 nano-gratings were elongated along the direction of the gratings.
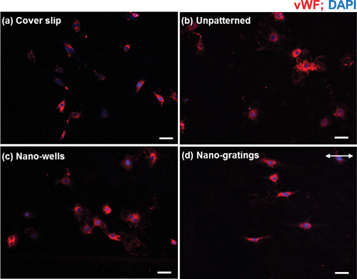 |
| Fig. 4 vWF expression of HUVEC on (a) a cover slip, (b) unpatterned TiO2, (c) TiO2 nano-wells and (d) TiO2 nano-gratings. The arrow shows the direction of the gratings (scale bar = 50 μm). | |
3.4. Endothelial and smooth muscle cell proliferation
The proliferation of vascular endothelial and smooth muscle cells was measured by EdU incorporation assay. Seeding density and experiment time points for both cell types were kept the same to facilitate comparison of the results between the two cell types. As shown in Fig. 5(a), vascular endothelial cells showed an increased proliferation rate on TiO2 nano-gratings as compared to the cover slip control and unpatterned TiO2 surface, though not statistically significant. Previously, endothelial cells demonstrated decreased proliferation on grating surfaces with a decreasing pitch size and their proliferation was significantly lower than that on the planar control.8 The data presented in this study suggests that imprinted TiO2 nano-gratings do not lower the proliferation rate of HUVECs as compared to the planar control. In fact, the proliferation rate was found to be slightly higher on nano-gratings as compared to the cover slip control and unpatterned TiO2. Nano-imprinted TiO2, on the other hand, demonstrated a greater impact on the proliferation of SMCs. As shown in Fig. 5(b), SMCs showed a significantly decreased proliferation rate on all of the TiO2 surfaces as compared to that on the cover slip control. Within various TiO2 surfaces, SMCs showed a statistically significant higher proliferation rate on the nano-wells as compared to that on unpatterned TiO2 and TiO2 nano-gratings. These results reveal that ther SMCs are able to sense underlying sub-100 nm biophysical cues and respond to them by modulating their proliferation capacity. It has been shown that the proliferation of SMCs is one of the major causes of restenosis after stent application.28,29 Hence, a decreased proliferation rate of SMCs is relevant and desired to resist restenosis. The current findings suggest that nano-imprinted TiO2 may be utilized to suppress the proliferation of SMCs and to achieve the competitive advantage of the proliferation of endothelial cells.
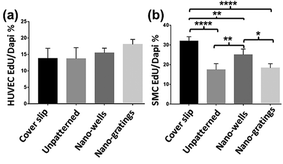 |
| Fig. 5 (a) HUVECs and (b) human coronary artery SMCs proliferation on various topographies as measured by the incorporation of EdU in the DNA of the proliferating cells. *p < 0.05; **p < 0.01; ***p < 0.005; ****p < 0.0001. | |
3.5. Effect of nano-imprinted TiO2 topography on cell shape
The actin cytoskeleton of the vascular endothelial cells was stained to analyze the shape of the cells. As shown in Fig. 6(a–d), endothelial cells showed a rounded morphology on unpatterned TiO2, and TiO2 nano-wells. On the contrary, on the TiO2 nano-gratings, the endothelial cells assumed a more elongated morphology along the direction of the gratings. In addition, the beta-actin of the SMCs was stained to visualize the cell shape [Fig. 6(e–h)]. The SMCs had a larger area as compared to the vascular endothelial cells and they were also aligned along the nano-gratings. Similarly, the SMCs did not show any visible trend in cell shape on unpatterned TiO2 and TiO2 nano-wells. To further investigate the effect of topography on the vascular endothelial and smooth muscle cell shape, morphometric analysis of the cell shape was carried out to quantify the cell aspect ratio. Endothelial cells on nano-gratings and patterned surfaces had a significantly higher aspect ratio (long axis
:
short axis) as compared to that on the nano-wells and unpatterned surfaces [Fig. 6(i)]. In the case of SMCs, no significant differences were found between the cell aspect ratio on different topographies [Fig. 6(j)]. SMCs had an aspect ratio of about 5–6 on cover slips, patterned, and unpatterned TiO2. The orientation and alignment of endothelial cells are important processes for the formation of new vessels and during endothelialization. Essential cell processes may be controlled by changes in the cell shape.30 Endothelial cells that line the blood vessel have a slightly elongated morphology due to the shear forces applied by the blood flow.31 A nano-patterned TiO2 surface layer could be used to modulate the endothelial cell shape by promoting a more native cellular morphology. It is well-known that cells can be aligned and elongated along the axis of micron and sub-micron gratings.7,32 However, there is a lack of understanding on the size threshold that the cells can sense and then align themselves accordingly. The proliferation and cell shape analysis shows that HUVECs and SMCs can sense and respond to gratings and well borders as small as 70 nm, by showing significant changes in proliferation and morphological changes.
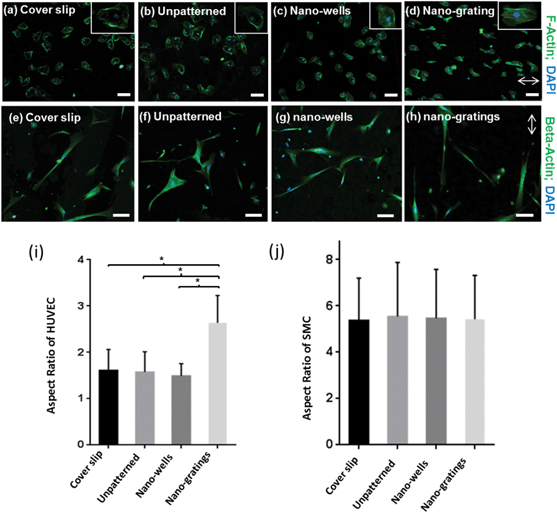 |
| Fig. 6 Fluorescent staining of F-actin in HUVECs showing the cell morphology on (a) a cover slip control, (b) unpatterned TiO2, (c) TiO2 nano-wells and (d) TiO2 nano-gratings. The inset shows a magnified view of the HUVEC cell morphology. Fluorescent staining of beta-actin to visualize the morphology of SMCs on (e) a cover slip control, (f) unpatterned TiO2, (g) TiO2 nano-wells and (h) TiO2 nano-gratings. Aspect ratio of (i) HUVEC and (j) SMCs on a cover slip control and various TiO2 substrates. The aspect ratio is defined as the ratio between the long axis and short axis of the cells. *p < 0.001 (scale bar = 100 μm). | |
3.6. Cell–surface interaction, focal adhesion kinase size, distribution and density
The detailed interaction of endothelial cells and patterned surfaces was characterized by scanning electron microscopy (SEM) [Fig. 7(a–d)]. The SEM images revealed that the cells were elongated along the nano-gratings. Meanwhile, no trends in the cell alignment were observed on unpatterned or nano-wells, on which the cells were randomly spread. More extensive filopodia formation of the HUVECs was observed on the cover slips and the TiO2 nano-wells. Endothelial cells were also stained for phosphorylated focal adhesion kinase (pFAK) to visualize the effect of topography on the size, distribution and density of FAs. As shown in Fig. 7(e–h), FA distribution, size and density were modulated by the underlying TiO2 nano-topography. HUVECs on unpatterned TiO2 and TiO2 nano-wells surfaces had an increased number of FAs as compared to HUVECs on TiO2 nano-gratings. FAs were distributed throughout the cytoplasm on unpatterned and TiO2 nano-wells while FAs were mostly found on the cell periphery, in the case of nano-gratings with the greater density, at the poles of elongated cells [Fig. 7(e–h)]. More FAs were observed on unpatterned TiO2 and TiO2 nano-wells as compared to the cover slip control and TiO2 nano-gratings. On the cover slip controls, the FAs were mostly located on the cell periphery. Modulation of FAs by patterned TiO2 topography was also analyzed quantitatively in terms of FAs size, FAs elongation and number of FAs per cell (Fig. 8). As shown in Fig. 8(a), FA size was significantly lower on unpatterned TiO2 as compared to that on the glass cover slips, TiO2 nano-gratings and nano-wells. No significant difference in FA size was found between the nano-gratings and nano-wells samples. FA size distribution can provide more useful information about the range of FA sizes found on various substrates. Fig. 8(b) shows the distribution of FA sizes. Almost half of the FAs on unpatterned TiO2 were found to be larger than 3 μm2 in size as compared to FAs on TiO2 nano-gratings and TiO2 nano-wells, where more than 65% of the FAs were larger than 3 μm2 in size. On both of the patterned TiO2 topography, FA size distribution was observed to be extended to up to 20 μm2 size. However, a more tight distribution was found on unpatterned TiO2 and almost no FAs were found beyond a size of 12 μm2. The FA size distribution on the glass cover slip was found to be similar to that on unpatterned TiO2 with a slightly less tight FA size distribution. Fig. 8(c) shows the elongation of FAs on various substrates. On the glass coverslip and unpatterned TiO2, the FAs were not elongated. However, on TiO2 nano-wells and nano-gratings, the elongation of FAs was significantly higher as compared to that on unpatterned TiO2. FA elongation was similar on nano-wells and nano-gratings although the area of the FAs was higher on the nano-wells.
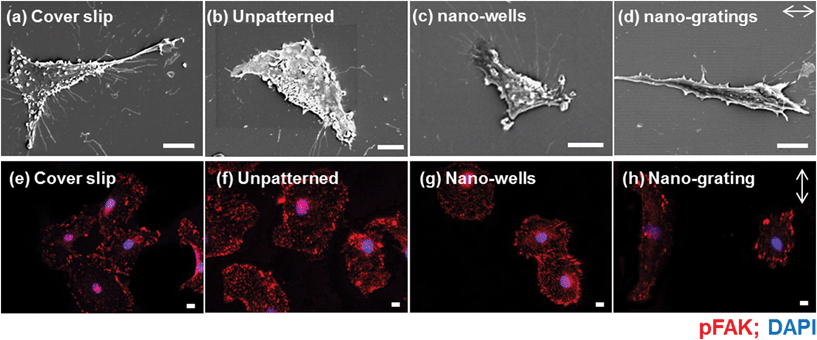 |
| Fig. 7 Scanning electron microscope images of shape of HUVECs on (a) glass cover slip, (b) unpatterned TiO2, (c) TiO2 nano-wells, and (d) TiO2 nano-gratings. Distribution of FAs as visualized by immunofluorescence staining of pFAK on (e) a cover slip control, (f) unpatterned TiO2, (g) TiO2 nano-wells and (h) TiO2 nano-gratings. The arrow shows the direction of the gratings (scale bar = 10 μm). | |
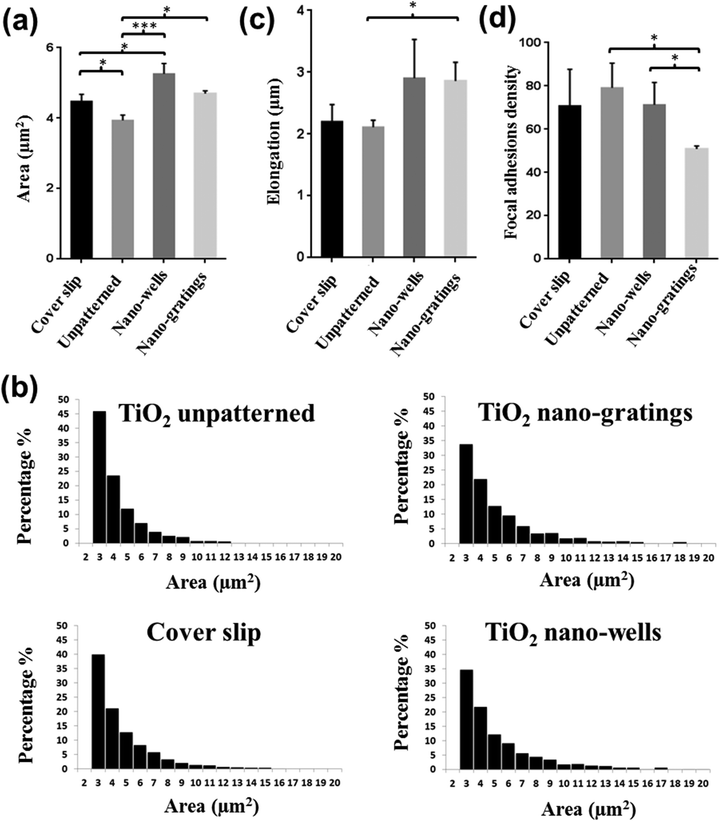 |
| Fig. 8 HUVECs FA size and density modulation by nano-imprinted TiO2. (a) Average FA size on a cover slip, unpatterned TiO2, TiO2 nano-wells and TiO2 nano-gratings. (b) Histogram showing the frequency distribution of FAs vs. the area on a cover slip and unpatterned, nano-wells and nano-gratings. On unpatterned TiO2, over 45% of the FAs were less than 3 μm2 in area with much tighter distribution overall while for patterned surfaces, less than 35% of the FAs were lower than 3 μm2 area with a long tail of distribution. (c) FA elongation on a cover slip and unpatterned, nano-wells and nano-gratings of patterned TiO2. (d) FA density on a cover slip, unpatterned TiO2, TiO2 nano-wells and TiO2 nano-gratings. FA density is defined as the number of FAs per cell. *p < 0.05; **p < 0.01; ***p < 0.005. | |
Fig. 8(d) shows the formation of FAs in terms of the number of FAs found per cell on various substrates. The average number of FAs per cell for TiO2 nano-gratings were found to be significantly lower as compared to that on cover slips, unpatterned TiO2 and TiO2 nano-wells. However, no significant difference in terms of the FA density was found between the cover slips, unpatterned TiO2 and TiO2 nano-wells. These results match with the qualitative observations of FA density made on the basis of pFAK-stained images [Fig. 7(e–h)]. Taken together, the results demonstrate the ability of TiO2 nano-wells to increase the formation of FAs and the degree of maturation as compared to unpatterned TiO2. On the other hand, TiO2 nano-gratings lead the FAs to maturation but the FA density was found to be the lowest among all of the analyzed surfaces. The patterned TiO2 topography seems to play an important role in mediating the formation and maturation of the FAs. Previously, Seo et al. found that the area of FAs and their formation were higher on a patterned square micro-wells topography as compared to that on unpatterned samples.33 However, in this study, we found that the FA area was higher on square nano-wells but the FA density (formation) per cell was slightly lower as compared to that on an unpatterned surface. We have also previously demonstrated that the FA area and elongation can be significantly different on micro-gratings and nano-gratings.34 Here we observed that FAs on TiO2 nano-gratings were elongated along the gratings and usually found on the poles of the cells [Fig. 6(h)]. Overall, the current study shows that a sub-100 nm topography can have a profound effect on FAs as it demonstrates that not only the FA size but also the FA density per cell is strongly influenced by TiO2 sub-100 nm topography. FA size and distribution have been speculated to mediate cell migration. It has been shown that FAs localization, size and density can predict cell migration.35–37 FA localization on poles of the elongated cells on TiO2 nano-gratings could modulate the direction of cell migration geared towards the gratings axis. This polar organization is reminiscent of vascular endothelial cells under a flow direction.38 The polar organization of FAs also increases the speed of cell migration.37 Generally, a smaller FA area and a lower density of FAs is believed to promote cell migration rates.35,36 The vascular endothelial cells in this study were found to have smaller FAs as compared to cells on TiO2 nano-wells. The FAs per cell were also significantly lower as compared to the endothelial cells on TiO2 nano-wells. Endothelial cells with increased cell migration rate may lead to the faster formation of intact endothelium where FAs play an important role in mediating the cell migration rate.37 Based on the findings of previous studies on the link between FAs and cell migration and the current finding of this study about FAs, it can be speculated that endothelial cells on nano-gratings may have an increased cell motility and migration rate which could ultimately enhance wound healing and re-endothelialization processes and in turn, inhibit restenosis as demonstrated previously on micro-gratings.2,39
In summary, our data indicate that the TiO2 resin-based nano-imprinted TiO2 surface layer is biocompatible and hence for the first time, it can be used to study how cells respond to biophysical cues in the form of patterned TiO2 topography in the sub-100 nm range. We have demonstrated that proliferation of HUVECs and SMCs can be modulated by a patterned TiO2 surface layer with optimal surface topographies as biophysical cues. Morphology of the vascular endothelial cells was also shown to be regulated by nano-topography to achieve a more native-like cell shape. Moreover, the FA size and distribution were also modulated by nano-imprinted TiO2. The study also illustrated the regulation of vascular endothelial and smooth cells functions by patterned TiO2 features as small as 70 nm and further studies are needed to explore other topographies in this size regime to achieve a more favourable environment for endothelialization. For example, we have clearly demonstrated the suppression of SMCs proliferation with our chosen topographies while these topographies did not reduce the proliferation of endothelial cells. Further studies could be performed to find a better topographical surface with biophysical cues that not only suppress the SMC proliferation but also enhance the proliferation of endothelial cells. Similarly, TiO2 patterned topography was demonstrated to have a significant effect on the FA density and size, which modulates the cell migration during endothelialization and wound healing processes.
4. Conclusions
In this study, we demonstrated that a biocompatible titanium dioxide (TiO2) surface layer could be patterned at high resolution using nano-imprint lithography. AlamarBlue assay and live/dead assay revealed that the as-prepared TiO2 is not cytotoxic to cells after heat-treatment. Vascular endothelial cells showed similar expression of vWF functional marker on all substrates. Patterned TiO2 nano-gratings significantly inhibited the proliferation of SMCs as compared to a cover slip control and increased the proliferation of endothelial cells as well. Morphometric studies also confirmed that endothelial cells are able to sense patterned TiO2 gratings as small as 70 nm and were found to be elongated along the gratings as measured by cell aspect ratio. Moreover, FA size, density and distribution was also significantly modulated by nano-imprinted TiO2, as the FA density was significantly lower on TiO2 nano-gratings and the FA size was significantly higher on TiO2 nano-gratings and nano-wells as compared to unpatterned TiO2. The study is the first to demonstrate the use of a biocompatible nano-patterned TiO2 layer for cell–topography interaction research to design improved surfaces for better endothelialization.
Acknowledgements
This work was partially funded by Singapore's A*STAR-ANR fund (1122703037) and the National Research Foundation, Singapore, under its Research Center of Excellence programme administered by the Mechanobiology Institute of Singapore. M. S. M. Saifullah acknowledges A*STAR-JST Project No. IMRE/12-2P0802 and the A*STAR Nanoimprint Foundry Project No. IMRE/13-2B0278 for financial support. S. H. Lim acknowledges IMRE-funded core Project no. IMRE:/12-1C0332 and IMRE:/12-1P0901 for financial support. R. Muhammad was supported by the Singapore International Graduate Award by the A*STAR Graduate Academy. S. H. Lim and S. H. Goh were supported by the A*STAR Scientific Staff Development Award Program.
Notes and references
- G. A. Abrams, S. S. Schaus, S. L. Goodman, P. F. Nealey and C. J. Murphy, Cornea, 2000, 19, 57–64 CrossRef CAS PubMed
.
- E. A. Sprague, F. Tio, S. H. Ahmed, J. F. Granada and S. R. Bailey, Circulation, 2012, 5, 499–507 Search PubMed
.
- A. Dibra, A. Kastrati, J. Mehilli, J. Pache, R. von Oepen, J. Dirschinger and A. Schomig, Catheter. Cardiovasc. Interv., 2005, 65, 374–380 CrossRef PubMed
.
- S. Brody, T. Anilkumar, S. Liliensiek, J. A. Last, C. J. Murphy and A. Pandit, Tissue Eng., 2006, 12, 413–421 CrossRef PubMed
.
- S. A. Biela, Y. Su, J. P. Spatz and R. Kemkemer, Acta Biomater., 2009, 5, 2460–2466 CrossRef CAS PubMed
.
- L. E. Dickinson, D. R. Rand, J. Tsao, W. Eberle and S. Gerecht, J. Biomed. Mater. Res., Part A, 2012, 100A, 1457–1466 CrossRef CAS PubMed
.
- E. K. F. Yim, R. M. Reano, S. W. Pang, A. F. Yee, C. S. Chen and K. W. Leong, Biomaterials, 2005, 26, 5405–5413 CrossRef CAS PubMed
.
- S. J. Liliensiek, J. A. Wood, J. Yong, R. Auerbach, P. F. Nealey and C. J. Murphy, Biomaterials, 2010, 31, 5418–5426 CrossRef CAS PubMed
.
- S. Choudhary, K. M. Haberstroh and T. J. Webster, Tissue Eng., 2007, 13, 1421–1430 CrossRef CAS PubMed
.
- D. Khang, J. Lu, C. Yao, K. M. Haberstroh and T. J. Webster, Biomaterials, 2008, 29, 970–983 CrossRef CAS PubMed
.
-
J. Lu, D. Khang and T. J. Webster, 35th Annual Northeast Bioengineering Conference, 2009, 1, 362–363
.
- C. C. Mohan, K. P. Chennazhi and D. Menon, Acta Biomater., 2013, 9, 9568–9577 CrossRef CAS PubMed
.
- C. C. Mohan, P. R. Sreerekha, V. V. Divyarani, S. Nair, K. Chennazhi and D. Menon, J. Mater. Chem., 2012, 22, 1326–1340 RSC
.
- F. Variola, J. B. Brunski, G. Orsini, P. Tambasco de Oliveira, R. Wazen and A. Nanci, Nanoscale, 2011, 3, 335–353 RSC
.
- J. Lu, M. P. Rao, N. C. MacDonald, D. Khang and T. J. Webster, Acta Biomater., 2008, 4, 192–201 CrossRef CAS PubMed
.
- A. E. Jantzen, H. E. Achneck and G. A. Truskey, J. Biomed. Mater. Res., Part A, 2013, 101, 3181–3191 Search PubMed
.
- G. Tepe, J. Schmehl, H. P. Wendel, S. Schaffner, S. Heller, M. Gianotti, C. D. Claussen and S. H. Duda, Biomaterials, 2006, 27, 643–650 CrossRef CAS PubMed
.
- D. Stoeckel, A. Pelton and T. Duerig, Eur. Radiol., 2004, 14, 292–301 CrossRef PubMed
.
- A. R. Pelton, V. Schroeder, M. R. Mitchell, X. Y. Gong, M. Barney and S. W. Robertson, J. Mech. Behav. Biomed. Mater., 2008, 1, 153–164 CrossRef CAS PubMed
.
- S. Shabalovskaya, J. Anderegg and J. Van Humbeeck, Acta Biomater., 2008, 4, 447–467 CrossRef CAS PubMed
.
- S. A. Shabalovskaya, Biomed. Mater. Eng., 1996, 6, 267–289 CAS
.
- M. F. Maitz and N. Shevchenko, J. Biomed. Mater. Res., Part A, 2006, 76, 356–365 CrossRef PubMed
.
- Z. Huan, L. E. Fratila-Apachitei, I. Apachitei and J. Duszczyk, J. Funct. Biomater., 2012, 3, 349–360 CrossRef CAS PubMed
.
- S. H. Lim, M. S. M. Saifullah, H. Hussain, W. W. Loh and H. Y. Low, Nanotechnology, 2010, 21 Search PubMed
, 285303.
- R. Ganesan, J. Dumond, M. S. M. Saifullah, S. H. Lim, H. Hussain and H. Y. Low, ACS Nano, 2012, 6, 1494–1502 CrossRef CAS PubMed
.
-
P. S. Heckbert, Graphics Gems IV, AP Professional, 1994 Search PubMed
.
- R. Ganesan, S. H. Lim, M. S. M. Saifullah, H. Hussain, J. X. Q. Kwok, R. L. X. Tse, H. A. P. Bo and H. Y. Low, J. Mater. Chem., 2011, 21, 4484–4492 RSC
.
- S. O. Marx, H. Totary-Jain and A. R. Marks, Circulation, 2011, 4, 104–111 CAS
.
- M. R. Bennett, S. Anglin, J. R. McEwan, R. Jagoe, A. C. Newby and G. I. Evan, J. Clin. Invest., 1994, 93, 820–828 CrossRef CAS PubMed
.
- R. Singhvi, A. Kumar, G. P. Lopez, G. N. Stephanopoulos, D. I. Wang, G. M. Whitesides and D. E. Ingber, Science, 1994, 264, 696–698 CAS
.
- M. J. Levesque and R. M. Nerem, J. Biomech. Eng., 1985, 107, 341–347 CrossRef CAS
.
- E. K. F. Yim, E. M. Darling, K. Kulangara, F. Guilak and K. W. Leong, Biomaterials, 2010, 31, 1299–1306 CrossRef CAS PubMed
.
- C. H. Seo, K. Furukawa, K. Montagne, H. Jeong and T. Ushida, Biomaterials, 2011, 32, 9568–9575 CrossRef CAS PubMed
.
- B. K. K. Teo, S. T. Wong, C. K. Lim, T. Y. S. Kung, C. H. Yap, Y. Ramagopal, L. H. Romer and E. K. F. Yim, ACS Nano, 2013, 7, 4785–4798 CrossRef CAS PubMed
.
- D.-H. Kim and D. Wirtz, FASEB J., 2013, 27, 1351–1361 CrossRef CAS
.
- M. A. Wozniak, K. Modzelewska, L. Kwong and P. J. Keely, Biochim. Biophys. Acta, 2004, 1692, 103–119 CrossRef CAS PubMed
.
-
G. M. Artmann and S. Chien, Bioengineering in Cell and Tissue Research, Springer, 2008 Search PubMed
.
- S. Li, P. Butler, Y. Wang, Y. Hu, D. C. Han, S. Usami, J. L. Guan and S. Chien, Proc. Natl. Acad. Sci. U. S. A., 2002, 99, 3546–3551 CrossRef CAS PubMed
.
- W. Casscells, Circulation, 1992, 86, 723–729 CrossRef CAS
.
Footnotes |
† Electronic supplementary information (ESI) available. See DOI: 10.1039/c4bm00212a |
‡ Contributed equally to the work. |
|
This journal is © The Royal Society of Chemistry 2014 |
Click here to see how this site uses Cookies. View our privacy policy here.