DOI:
10.1039/C4BM00073K
(Paper)
Biomater. Sci., 2014,
2, 1384-1398
Angiopoietin-1 peptide QHREDGS promotes osteoblast differentiation, bone matrix deposition and mineralization on biomedical materials†
Received
6th March 2014
, Accepted 26th May 2014
First published on 27th June 2014
Abstract
Bone loss occurs as a consequence of a variety of diseases as well as from traumatic injuries, and often requires therapeutic intervention. Strategies for repairing and replacing damaged and/or lost bone tissue include the use of biomaterials and medical implant devices with and without osteoinductive coatings. The soluble growth factor angiopoietin-1 (Ang-1) has been found to promote cell adhesion and survival in a range of cell types including cardiac myocytes, endothelial cells and fibroblasts through an integrin-dependent mechanism. Furthermore, the short sequence QHREDGS has been identified as the integrin-binding sequence of Ang-1, and as a synthetic peptide it has been found to show similar integrin-dependent effects as Ang-1 in the aforementioned cell types. Integrins have been implicated in osteoblast differentiation and bone mineralization, processes critical to bone regeneration. By binding integrins on the osteoblast surface, QHREDGS could promote cell survival and adhesion, as well as conceivably osteoblast differentiation and bone mineralization. Here we immobilized QHREDGS onto polyacrylate (PA)-coated titanium (Ti) plates and polyethylene glycol (PEG) hydrogels. The osteoblast differentiation marker, alkaline phosphatase, peaked in activity 4–12 days earlier on the QHREDGS-immobilized PA-coated Ti plates than on the unimmobilized, DGQESHR (scrambled)- and RGDS-immobilized surfaces. Significantly more bone matrix was deposited on the QHREDGS-immobilized Ti surface than on the other surfaces as determined by atomic force microscopy. The QHREDGS-immobilized hydrogels also had a significantly higher mineral-to-matrix (M/M) ratio determined by Fourier transform infrared spectroscopy. Alizarin Red S and von Kossa staining and quantification, and environmental scanning electron microscopy showed that while both the QHREDGS- and RGDS-immobilized surfaces had extensive mineralization relative to the unimmobilized and DGQESHR-immobilized surfaces, the mineralization was more considerable on the QHREDGS-immobilized surface, both with and without the induction of osteoblast differentiation. Finally, treatment of cell monolayers with soluble QHREDGS was demonstrated to upregulate osteogenic gene expression. Taken together, these results demonstrate that the QHREDGS peptide is osteoinductive, inducing osteoblast differentiation, bone matrix deposition and mineralization.
1. Introduction
Bone loss can result from a traumatic injury or from a variety of diseases including infection, osteoporosis, arthritis, or cancer.1 While biomaterials and medical implant devices are used to restore, maintain, and/or improve the function of lost or damaged bone,2,3 the gold standard for treatment is still autologous bone grafts despite decades of ongoing research.4 Yet, autologous bone is a less than ideal material because it is limited in its availability and bone harvesting can induce donor site morbidity.5,6 These issues have been addressed to a degree by the development of allogeneic cadaveric bone tissue, but this material is itself associated with a number of challenges including infection and/or the host immune response.7,8 Meanwhile, research into biomaterials and medical implant devices has advanced these technologies from simple structural supports to materials that actively promote bone regeneration,1,3 but while they can promote osteoblast attachment, migration and growth, few can stimulate osteoblast differentiation and bone mineralization, i.e. promote bone formation.3
Interactions between the extracellular matrix (ECM) and integrin-type receptors initiate important signaling pathways for osteogenic differentiation and bone formation.9 Hence research is underway to investigate the potential of targeting integrins as a means of increasing bone formation and repair.9 Integrins are heterodimeric transmembrane adhesion receptors composed of α- and β-subunits that mediate the transduction of signals10 and have been identified as important for all stages of bone generation: osteoblast adhesion and differentiation (α1β1, α2β1, α4β1, α5β1, α11β1, and αvβ3), osteoblast survival (α2β1, α11β1), bone mineralization (α5, β1, αvβ3 and α2β1), and in vivo bone formation (α4β1, α5β1).9,11 The integrin ligand angiopoietin-1 (Ang-1)—a 498 amino acid, multi-domain secreted glycoprotein12—has been identified to effectively promote cell adhesion and survival in a variety of cell types, including fibroblasts, skeletal myocytes and cardiac myocytes,13,14 thus exhibiting features that suggest that it has potential as a regulator of bone formation and repair. However, the size and complexity of Ang-1 limit its ability to function in the context of medical device coatings and biomaterials, but opportunely the eponymous 7 amino acid peptide QHREDGS—the integrin-binding site of Ang-1
14—is equally effective as a pro-adhesion, pro-survival molecule in various cell types.15,16 Immobilizing entire proteins onto medical devices presents challenges related to the cost of recombinant growth factor production, as well as the possibility of denaturation during the immobilization procedure or storage. These challenges can be overcome through the use of short, linear peptides. Moreover, the peptide QHREDGS was demonstrated to act through α5β1 and αvβ3 receptors in endothelial cells.15 Given the role of integrins in bone formation—particularly of the β1 subtype, identified to participate in all stages of bone development—and of the ability of QHREDGS to interact with these receptors, we sought to determine whether QHREDGS could promote bone formation, specifically by inducing osteoblast differentiation and bone mineralization.
Medical devices to treat bone loss are usually composed of titanium (Ti) due to its advantageous physical properties and biocompatibility.17 However, Ti can deteriorate upon implantation18 and the extent of bone formation and repair it can achieve remains below the standards for successful treatment.19 Coatings have been developed to improve upon the existing attributes. Polyacrylate (PA) coatings prevent corrosion and reduce metal ion release without compromising the biocompatibility,20 while also allowing for covalent immobilization of bioactive molecules that can improve the osteogenic capacity of the Ti device. The development of biomaterials used to treat bone loss has progressed through the identification of new biocompatible materials as well as the identification of bioactive molecule modifiers that induce bone growth.21,22 But these too have had limited success because the quantity of growth factors required to induce bone formation vastly exceeds physiological levels and local application is required to avoid complications such as ectopic bone formation, necessitating very controlled release of these factors.23 We therefore sought to determine whether QHREDGS could be used to improve the success of both medical implant devices and biomaterials to treat bone loss by investigating the effectiveness of the QHREDGS peptide in inducing bone formation while being immobilized onto PA-coated Ti medical plates or inert polyethylene glycol (PEG) hydrogels. PA-coated Ti medical plates were used since they are an example of clinically used materials, while inert PEG hydrogels were used to isolate the effect of the immobilized peptide on the cells, as PEG hydrogels are inherently non-adhesive and non-fouling.
2. Materials and methods
MG-63 human osteosarcoma cells (ATCC, Manassas, VA). Uncoated and polyacrylate-coated titanium plates (a gift from Covalon Technologies Ltd, Mississauga, ON). Custom peptide DGQESHR (Biomatik Corporation, Cambridge, ON). Custom peptide RGDS (Biomatik Corporation, Cambridge, ON). Custom peptide NH2-QHREDGS-CONH2 (Institute for Biomolecular Design, Edmonton, AB). Custom Peptide FITC-aminohexanoic acid-QHREDGS-CONH2 (Institute for Biomolecular Design, Edmonton, AB). Poly(ethylene glycol)diacrylate, Mn 700 (Sigma Aldrich, Oakville, ON). Acrylate-PEG-NHS Ester MW 3500 (Jenkem Technologies, Allen, TX). GelBond PAG Film (Lonza, Basel, CH). 22 × 22 mm Vinyl coverslips (Bel-Art Scienceware, Wayne, NJ). 2-Hydroxy-2-methyl-propiophenone (Sigma Aldrich, Oakville, ON). Dulbecco's phosphate-buffered saline without Ca2+ and Mg2+ (Gibco/Life Technologies, Burlington, ON).
2.1. Peptide immobilization onto polyacrylate (PA)-coated titanium (Ti) plates
To quantify the amount of QHREDGS peptide conjugated to the Ti plates (5 mm × 5 mm × 0.3 mm), uncoated or PA-coated Ti plates were placed in a solution containing the conjugating reagents ethyl(dimethylaminopropyl) carbodiimide (EDC; Thermo Scientific, Rockford, IL) and sulfo-N-hydroxysuccinimide (NHS; Thermo Scientific) in a 1
:
2.5 molar ratio in ddH2O for 15 min with shaking at 700 rpm at 20 °C. To determine whether QHREDGS interacted non-specifically with the PA-coating, PA-coated Ti plates were also incubated with NHS in the absence of EDC. After 15 min, the Ti plates were incubated in a solution of 150 μg FITC-labeled QHREDGS (FITC-QHREDGS, reconstituted in phosphate buffered saline, PBS) in ddH2O for 3 h with shaking at 700 rpm at 20 °C, and then placed to wash in excess ddH2O overnight at 4 °C. The reaction and wash solutions were read in a SpectraMax GeminiXS fluorescent plate reader with the SoftMax Pro 3.2.1 software (Molecular Devices, Sunnyvale, CA) and the amount of FITC-QHREDGS therein was determined from a standard curve. The amount of FITC-QHREDGS conjugated to the plate was determined by subtracting the sum of the amounts of peptide in the solutions from the original amount of the peptide added. Direct measurement of the Ti plates was not possible because of fluorescence quenching by the non-transparent substrate.
For experiments, uncoated and PA-coated Ti plates were incubated with EDC/NHS as outlined above, and then incubated in solutions of PBS with or without 320 μM peptide (QHREDGS, RGDS or DGQESHR, reconstituted in PBS) for 3 h with shaking at 700 rpm at 20 °C. The plates were washed, as above, and then placed into low attachment 24-well culture plates (Corning Inc., Corning, NY) in 70% ethanol overnight under UV in a biosafety cabinet. The sterilized plates were washed twice with sterile PBS and then left to air dry for 5 minutes prior to cell seeding.
2.2. Peptide immobilization onto polyethylene glycol (PEG) hydrogels
To quantify the amount of QHREDGS peptide conjugated to the PEG hydrogels, the FITC-QHREDGS peptide was incubated with 4.0 mg acrylate-PEG-NHS in a 100 μL solution of 50 mM Tris pH 8.5 containing 0 μL, 10 μL, 20 μL, or 40 μL of 50 mM FITC-QHREDGS solution (reconstituted in PBS) for 3 h with shaking at 700 rpm at 20 °C. After 3 h, the reaction solutions were diluted to 1 mL with ddH2O and dialyzed extensively against ddH2O. The dialyzed reaction solutions were lyophilized and resuspended in 80 μL ddH2O. The amount of FITC-QHREDGS contained in the reconstituted solution was determined from a standard curve using a SpectraMax GeminiXS fluorescence plate reader and the SoftMax Pro 3.2.1 software.
For experiments, the peptides were incubated with 4.0 mg acrylate-PEG-NHS in a 100 μL solution of 50 mM Tris pH 8.5 with or without 10 μL of 50 mM peptide (QHREDGS, RGDS or DGQESHR, reconstituted in PBS) for 3 h with shaking at 700 rpm at 20 °C. After 3 h, the reaction solutions were diluted with ddH2O to 1 mL and dialyzed extensively against ddH2O. The dialyzed reaction solutions were lyophilized and resuspended in ddH2O, to which polyethylene glycol diacrylate (PEGDA) was added in a ratio of 50 mol PEGDA to 1 mol acrylate-PEG-NHS, containing 0.5% w/w 2-hydroxy-2-methyl-propiophenone in a final volume of 80 μL. For each hydrogel, 25 μL of this solution was pipetted onto a 22 × 22 mm square of the GelBond PAG film and a vinyl coverslip of equal size was gently placed on top. The hydrogel was placed under UV light for 10 min to polymerize, then placed into a low attachment 6-well culture plate (Corning Inc., Corning, NY), washed in PBS for 10 min on a shaker and then incubated at 4 °C for 1 h. After 1 h, the coverslip was gently peeled off the hydrogel with tweezers and the hydrogel was placed in 70% ethanol under UV light for 1 h to sterilize. Sterilized hydrogels were incubated in DMEM/10%FBS medium overnight prior to seeding with cells.
2.3. Cell culture
MG-63 human osteosarcoma cells were grown to confluence in T-25 flasks in DMEM (Gibco) with 10% FBS (Gibco) and 10% Penstrep (Gibco). All experiments were performed on cell passages 5–8. To seed the Ti plates, the cells were washed with PBS, dissociated with 0.05% trypsin (Gibco), centrifuged for 5 min at 2000 rpm and then resuspended in a minimum volume of culture medium. The cells were counted and 3–5 μL of cell suspension was pipetted onto the centre of the 25 mm2 Ti plate at a density of 3200 cells per 25 mm2 plate (day 0). The seeded plates were then incubated at 37 °C for 30 min to allow the cells to adhere, at which point 1 mL of media was added to each well gently. As controls, media was added to wells in the absence of Ti plates with or without an equal volume of cell suspension. The conditioned media was collected from the wells every other day and replaced with fresh media. Beginning on day 12, the media was supplemented with 50 μg mL−1 α-ascorbic acid, 10 mM β-glycerophosphate and 100 nM dexamethasone (osteogenic media) and cultured for a total of 34 days. The conditioned media samples were aliquoted and stored at −20 °C until needed. Additionally, Ti plates were seeded with 200
000 cells per 25 mm2 plate and cultured for 22 days in DMEM/10% FBS media without any supplementation (non-osteogenic media).
The PEG hydrogels were seeded as described above, but with some modifications. The cells were counted and 100 μL of cell suspension was pipetted onto the centre of the 484 mm2 hydrogel at a density of 62
000 cells (day 0) such that the seeding cell density was the same for the Ti plates and PEG hydrogels. After incubation at 37 °C for 30 min, 4 mL of media was added to each well gently. Beginning on day 15, the hydrogels were switched to the osteogenic media and cultured for a total of 41 days. On day 41, cells bound to the hydrogel were determined by washing with PBS, dissociating with 0.05% trypsin (Gibco), centrifuging for 5 min at 2000 rpm, resuspending the cells and performing 6 separate counts per hydrogel using a hemocytometer.
For dose response experiments, MG-63 cells were seeded into 24-well polystyrene tissue culture plates in DMEM with 10% FBS in the presence of 5, 50 or 500 μM soluble QHREDGS or equivolume PBS (1% volume). Once cells were confluent, media was supplemented with 50 μg mL−1 α-ascorbic acid, 10 mM β-glycerophosphate and 100 nM dexamethasone (osteogenic media) and cells were cultured for an additional 22 days. Over the course of the culture period, the media was changed every other day and additional QHREDGS/PBS was added with each media change.
2.4. Alizarin Red S and von Kossa staining
The seeded Ti plates were fixed on day 22 or 34 in 4% paraformaldehyde (PFA) for 15 min at 20 °C. The plates were washed once with ddH2O and then incubated with a stain solution of 1% Alizarin Red S (Sigma Aldrich) in ddH2O for 5 min at 20 °C. Plates were washed twice with ddH2O and then imaged using an Olympus CKX41 inverted microscope at 10× magnification, with an Olympus SC30 Digital Camera and the Olympus cellSense Digital Imaging Software (Olympus America Inc., Center Valley, PA). Following Alizarin Red S staining and imaging, the plates were subjected to von Kossa staining. The plates were washed thrice in ddH2O, incubated with a stain solution of 5% silver nitrate in ddH2O for 30 min, washed thrice, developed with a freshly made solution of 5% sodium carbonate in 2.5% formaldehyde in ddH2O for 5 min, washed thrice, fixed with a solution of 5% sodium thiodisulphate for 2 min, washed thrice, and then re-imaged.
To quantify the amount of Alizarin Red S bound to the various surfaces, images were analyzed using the ImageJ software (NIH, Bethesda, MD). The images were first split into red, green and blue channels, and the red channel images were used for all further analysis. For the Ti plates cultured in osteogenic medium, the mineralization was too extensive on some surfaces to distinguish mineral deposits, therefore the raw integrated density (sum of pixel values) of the images was measured. For the Ti plates cultured in non-osteogenic medium and the hydrogels, the red channel images were thresholded and the percent area of staining was measured.
2.5. Alkaline phosphatase activity assay
The alkaline phosphatase (ALP) activity of the conditioned medium samples collected from the seeded Ti plates was measured using the Alkaline Phosphatase Yellow Liquid p-nitrophenol phosphate (pNPP) substrate for ELISA (Sigma Aldrich) as previously described24 with some modification. Briefly, all samples and the pNPP substrate were brought to room temperature before beginning the assay, then 10 μL of sample was added to wells of a 96-well plate, to which 100 μL of the pNPP substrate was added. Immediately after addition of the substrate, the plate was read at 405 nm every 10 seconds for 30 min in a Molecular Devices VersaMax Tunable microplate reader (Molecular Devices, Sunnyvale, CA). The ALP activity (IU L−1) was calculated from the formula:
2.6. Glucose consumption assay
The glucose content of the conditioned medium samples collected from the seeded Ti plates was measured using a QuantiChrom Glucose Assay Kit (BioAssay Systems, Hayward, CA), performed according to the supplier's instructions.
2.7. Cytotoxicity assay
The lactate dehydrogenase (LDH) content of the conditioned medium samples collected from the seeded Ti plates was measured using a LDH Cytotoxicity Assay Kit (Cayman Chemical Company, Ann Arbor, MI), performed according to the supplier's instructions.
2.8. Quantitative PCR
MG-63 monolayers were grown in a 24-well polystyrene tissue culture plate in the presence of 5, 50 or 500 μM soluble QHREDGS or equivolume PBS in osteogenic media for 22 days. RNA was isolated every four days, reverse transcribed into cDNA and qPCR was performed, as previously described,25 using primers for bone sialoprotein, osteocalcin, collagen type I and β-actin as previously described26 and alkaline phosphatase, as previously described.27 Expression levels were normalized to the housekeeping gene β-actin.
2.9. Attenuated total reflectance (ATR) Fourier transform infrared (FTIR) spectroscopy
On day 41, seeded hydrogels were fixed in 4% PFA, washed with ddH2O, and then analyzed by ATR-FTIR spectroscopy. On day 34, seeded Ti plates were fixed in 4% PFA, washed extensively over a period of weeks with ddH2O to remove the mineral and then analyzed by ATR-FTIR spectroscopy. Transmission spectra were obtained using an ATR top-plate accessory coupled to a Spectrum One FTIR spectrometer with a fast recovery deuterated triglycine sulfate detector (PerkinElmer, Inc., Waltham, MA). The spectra were recorded in the region between 4000 and 650 cm−1. For each Ti plate or hydrogel, 5–11 randomly selected regions were measured and for each spectrum, at least 4 scans were averaged. The peak areas were determined from baseline-corrected absorbance spectra using the SPECTRUM software (PerkinElmer). The collagen (matrix) concentration of the bone matrix was determined by combining the areas of the absorbance peaks in the regions of 1720 (amide I), 1640 (amide II) and 1250 cm−1 (amide III). The phosphate (mineral) content of the bone matrix was determined from the areas of the absorbance peaks in the region of 900–1200 cm−1. The ratio of mineral-to-matrix (M/M ratio) was determined by dividing the phosphate concentration by the collagen concentration.
2.10. Atomic force microscopy (AFM)
Titanium plates seeded with 3200 cells per 25 mm2 plate, cultured in osteogenic medium for a total of 34 days and then fixed in 4% PFA. The plates were washed extensively over a period of weeks with ddH2O to remove the mineral and then subjected to contact mode AFM. Force spectroscopy measurements, yielding force–distance curves, were performed in ddH2O using a JPK NanoWizard II AFM (JPK Instruments AG, Berlin, Germany). Measurements were conducted at ambient temperature and the samples were conscientiously maintained in a hydrated state. The cantilevers used were V-shaped silicon nitride cantilevers with a spring constant of 0.1 N m−1 (Bruker, Camarillo, CA). The cantilever deflection sensitivity was obtained by indenting the tip against a glass substrate and the tip radius was measured to be 26 nm. Three to six sections of the surface were measured and a 10 μm × 10 μm area was scanned per section, with probe velocities of 1–5 μm s−1. To determine the Young's modulus, a Hertzian model was applied to the extension force–displacement curves with the appropriate fit range manually determined for each curve using the JPK Image Processing Software (JPK Instruments AG, Berlin, Germany). In our calculations, we assumed the tip to be a paraboloidal indenter.
2.11. Environmental scanning electron microscopy (ESEM)
The Ti plates seeded at a density of 200
000 cells per 25 mm2 and cultured for 22 days in non-osteogenic media were fixed for 30 min in 4% PFA/1% glutaraldehyde, washed with ddH2O and imaged using a Hitachi VP-SEM S3400N Variable Pressure SEM (Hitachi High Technologies America, Inc., Roslyn Heights, NY) under low vacuum (70 Pa) with an accelerating voltage of 15 kV. Images were obtained with two magnifications using the backscatter (BSE) detector.
2.12. Statistical analysis
Analyses of two groups were performed with Student's t-test. Analyses of more than two groups were performed using a one-way ANOVA with Student's–Newman–Keuls (SNK) multiple-comparison test for normally distributed data and a Kruskal–Wallis one-way ANOVA on ranks with Dunn's post-hoc analysis for data that failed the normality test using the SigmaPlot 12 software (Systat Software Inc, San Jose, CA) with P < 0.05 considered to be significant.
3. Results
3.1. QHREDGS peptide can be immobilized onto polyacrylate (PA)-coated titanium (Ti) and polyethylene glycol (PEG)-based hydrogels using ethyl(dimethylaminopropyl)carbodiimide (EDC)- and N-hydroxysulfosuccinimide (NHS)-based chemistry
The initial experiments were performed to demonstrate that the small peptide QHREDGS could be immobilized onto PA-coated Ti plates and PEG hydrogels using basic EDC/NHS-based immobilization chemistry. Since the functionality of the peptide is not orientation-dependent, a specific conjugation method was not required and EDC/NHS chemistry was chosen for its frequent usage in such applications. We quantified the amount of peptide that was immobilized using a FITC-labeled variant (FITC-QHREDGS). Peptides do not readily react with titanium unless the surface has been modified to facilitate covalent immobilization; herein titanium plates were coated with polyacrylate, which has reactive carboxylic groups to which peptides can be conjugated. Both the uncoated Ti plates incubated with the fluorescently labeled peptide in the presence of both NHS and EDC and the PA-coated Ti plates incubated with NHS in the absence of EDC had a detectable amount of FITC-QHREDGS associated with them (14.7 ± 6.1 μg and 16.3 ± 6.7 μg of FITC-QHREDGS, respectively; Fig. 1A); however, the amount of FITC-QHREDGS associated with the plates under these conditions did not differ significantly (P > 0.05, n = 3; Fig. 1A). Thus, the peptide can physically absorb equally well onto both uncoated and PA-coated Ti, indicating that coating Ti with polyacrylate does not promote non-specific, non-covalent binding. In the presence of EDC, an additional 18.7 ± 5.2 μg of FITC-QHREDGS was specifically and covalently immobilized onto the PA-coated Ti plate resulting in an immobilized peptide surface concentration of 0.33 ± 0.09 μg mm−2 (0.27 ± 0.08 nmol mm−2), a significant increase in the amount of peptide relative to either the uncoated Ti (P = 0.02, n = 3; Fig. 1A) or the PA-coated Ti plate in the absence of EDC (P = 0.03, n = 3; Fig. 1A). The efficiency of the FITC-QHREDGS immobilization onto PA-coated Ti was 15.61 ± 3.0% (Fig. 1B), which was significantly higher than that for the uncoated Ti plate (P = 0.005, n = 3; Fig. 1B) or the PA-coated Ti plate in the absence of EDC (P = 0.02, n = 3; Fig. 1B).
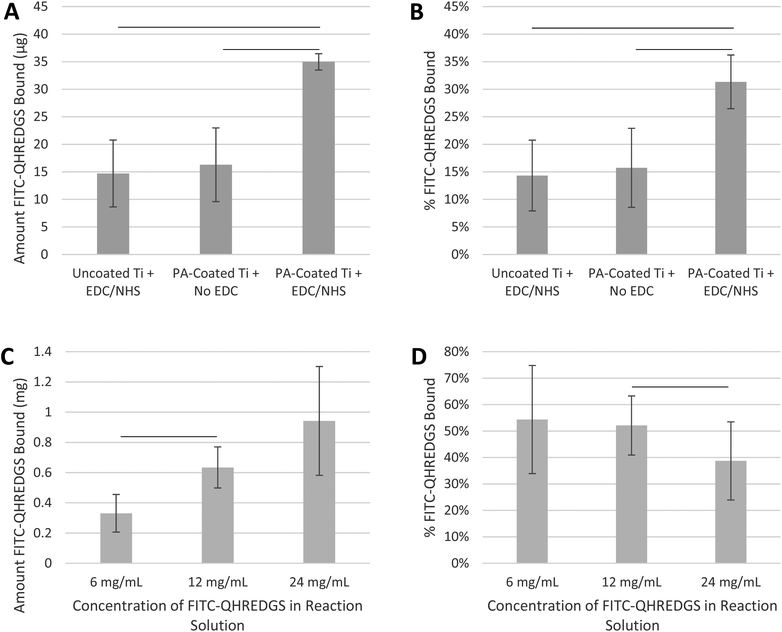 |
| Fig. 1 Covalent immobilization of QHREDGS onto PA-coated Ti plates using EDC/NHS chemistry and onto PEG hydrogels using acrylate-PEG-NHS. Uncoated or PA-coated Ti plates were placed in a reaction mixture containing FITC-QHREDGS and the conjugating reagent NHS in the presence or absence of EDC: (A) the amount of FITC-QHREDGS peptide bound to the Ti plate; (B) the percentage of FITC-QHREDGS bound to the Ti plate of the initial amount added to the reaction solution. The PEG-derivative acrylate-PEG-NHS was incubated in a reaction mixture containing different concentrations of FITC-QHREDGS: (C) the amount bound to the PEG-derivative in the hydrogel; (D) the percentage of FITC-QHREDGS bound to the PEG-derivative of the initial amount of peptide added to the reaction solution. The data presented are the mean ± the SEM (n = 3) and the lines indicate statistical significance (P < 0.05; one-way ANOVA and Student–Newman–Keuls post-hoc analysis; n = 3). | |
Similarly, FITC-QHREDGS was conjugated to PEG hydrogels by incubating the peptide with a bifunctional PEG derivative, acrylate-PEG-NHS, which was then incorporated into the hydrogel. Increasing the concentration of FITC-QHREDGS incubated with the acrylate-PEG-NHS resulted in an increased amount of FITC-QHREDGS bound to the PEG derivative producing hydrogels with FITC-QHREDGS concentrations of 4.1 ± 1.6 mg mL−1 (3.4 ± 1.3 nM) for the 6 mg mL−1 reaction solution, 7.9 ± 1.7 mg mL−1 (6.5 ± 1.4 nM) for the 12 mg mL−1 solution and 11.7 ± 4.5 mg mL−1 (9.7 ± 3.7 nM) for the 24 mg mL−1 solution (Fig. 1C). The immobilization efficiency ranged from 34 to 75% for the 6 mg mL−1 FITC-QHREDGS reaction solution, 41 to 63% for the 12 mg mL−1 solution, and 24 to 53% for the 24 mg mL−1 solution (Fig. 1D). Increasing the reaction solution concentration from 6 mg mL−1 to 12 mg mL−1 resulted in a significant increase in the amount of FITC-QHREDGS that was immobilized onto the PEG derivative (P = 0.003, n = 3; Fig. 1C), but did not significantly improve the efficiency (P > 0.05, n = 3; Fig. 1D). Increasing the reaction solution concentration from 12 mg mL−1 to 24 mg mL−1 did not significantly increase the amount of immobilized FITC-QHREDGS (P > 0.05, n = 3; Fig. 1C) but significantly reduced the immobilization efficiency (P = 0.04, n = 3; Fig. 1D).
3.2. QHREDGS immobilization onto PA-coated Ti accelerates osteoblast differentiation
Alkaline phosphatase (ALP), a marker of osteoblast differentiation, is a cell surface enzyme located on the outer plasma membrane of osteoblasts28 where it catalyzes the hydrolysis of phosphomonoesters (R-O-PO3) releasing inorganic phosphate;29 a process that promotes bone mineralization. MG-63 osteoblast-like cells were grown to confluence on Ti plates with variable coatings (with and without peptides) and induced to differentiate on day 12 by culturing the cells in the osteogenic medium. On day 18, the ALP activity detected in the conditioned media did not differ significantly between the various treatment groups (Fig. 2). By day 22, the amount of ALP activity detected in the conditioned media of the cells grown on PA-coated Ti plates with immobilized QHREDGS was significantly more than that detected in the conditioned media of the cells grown on any other surfaces (P < 0.05; n = 3; Fig. 2). The amount of ALP activity detected in the conditioned media of MG-63 cells grown on polystyrene tissue culture plastic or on uncoated Ti did not change significantly over the course of the assay (P > 0.05, n = 3; Fig. 2). Conversely, the amount of ALP activity detected in the conditioned media of cells grown on any of the various PA-coated Ti surfaces did significantly increase with maxima on day 22 for the QHREDGS-immobilized surface, on day 26 for the RGDS-immobilized and on day 34 for both the PA-coated Ti plate (without any peptide) and DGQESHR-immobilized surfaces (Fig. 2); however, the ALP activity maxima did not vary significantly (P > 0.05, n = 3). The amount of ALP activity detected in the conditioned medium of cells grown on the DGQESHR-immobilized surface on day 34 was found to be inconsistent with wide ranging values; however, it did not differ significantly from the negligible amount of activity detected from the conditioned medium of cells grown on the low-attachment polystyrene surface (0.25 ± 0.12 IU L−1 and 0.0079 ± 0.0079 IU L−1, respectively; P > 0.05, n = 3; Fig. 2).
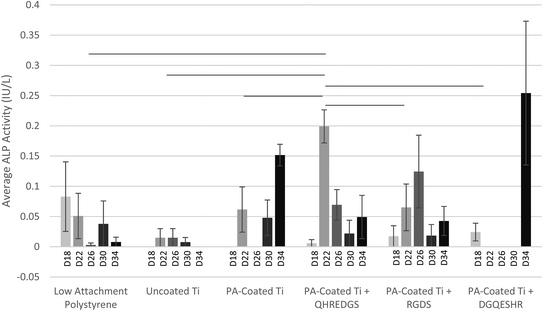 |
| Fig. 2 Alkaline phosphatase (ALP) activity measured in conditioned medium collected on the indicated day (D) of osteoblasts grown on low attachment polystyrene tissue culture plastic or Ti plates without PA-coating (Uncoated Ti), with PA-coating but no peptide conjugated (PA-coated Ti), or with PA-coating and either QHREDGS, RGDS or DGQESHR (scrambled) peptide conjugated. Data presented are the mean ± SEM and the lines indicate statistical significance (P < 0.05; one-way ANOVA and Student–Newman–Keuls post-hoc analysis; n = 3). | |
The significant increase in ALP activity on day 22 in the conditioned media of cells grown on the QHREDGS-immobilized surface cannot be attributed to increased metabolic activity as there was no significant difference in glucose consumption at this time point for the QHREDGS-immobilized surface relative to any other surface (P > 0.05, n = 3; Fig. S1†). While there was a 40% increase on average in glucose consumption between day 18 and day 22 for the cells grown on the QHREDGS-immobilized surface, the increase was non-significant and these glucose consumption values did not differ significantly from those measured for the other surfaces (P > 0.05, n = 3; Fig. S1†). Similarly, the amount of cell death—as determined by the lactate dehydrogenase (LDH) activity in the conditioned medium—decreased 40% on average between day 18 and day 22 for the QHREDGS-immobilized surfaces, however this decrease was not significant, nor did the LDH activities on either day 18 or day 22 differ significantly from that measured for the other surfaces (Fig. S2†). The amount of cell death did not change significantly over the culture period for any of the peptide-immobilized surfaces (P > 0.05; n = 3; Fig. S2†) and the amount of cell death on the QHREDGS-immobilized surface did not differ significantly from the other surfaces (P > 0.05; n = 3; Fig. S2†). Neither glucose consumption (Fig. S1†) nor LDH activity (Fig. S2†) varied significantly between the surfaces over the duration of the culture period, indicating that the surface modifications did not adversely or advantageously affect cellular metabolic activity or cell death.
3.3. Soluble QHREDGS promotes upregulation of osteogenic genes
In order to determine the effect of treatment with the soluble QHREDGS peptide, MG-63 cells were grown to confluence on tissue culture plastic and treated with the soluble peptide for a 22-day period of culture in osteogenic medium. At various time points, the cells were lysed, RNA was extracted and qPCR was performed to determine the effect of increasing QHREDGS peptide concentration on the gene expression of ALP, osteocalcin, collagen type I and bone sialoprotein. Relative to the control equivolume PBS treatment, the concentration of 5 μM QHREDGS added to the media resulted in a notable increase in ALP gene expression on day 10, and a QHREDGS concentration of 50 μM resulted in increased ALP gene expression on days 6–14 relative to the control (Fig. 3A and Fig. S3A†). Interestingly, treatment with 500 μM QHREDGS did not increase ALP gene expression relative to the control. Osteocalcin gene expression was significantly upregulated by 50 μM treatment on day 6 (P = 0.05, n = 3; Fig. 3B and Fig. S3B†) but was significantly downregulated by 500 μM QHREDGS treatment on day 14 (P = 0.03, n = 3; Fig. 3B and Fig. S3B†) relative to the control. Collagen type I gene expression was also significantly upregulated on day 10 with 5 μM QHREDGS treatment (P = 0.05, n = 3; Fig. 3C and Fig. S3C†) and on day 14 by 50 μM QHREDGS (P = 0.002, n = 3; Fig. 3C and Fig. S3C†), and significantly downregulated by 500 μM QHREDGS treatment on day 22 (P = 0.01, n = 3; Fig. 3C and Fig. S3C†), relative to the control. Finally, bone sialoprotein gene expression was significantly increased on days 6 and 10 with 5 μM QHREDGS treatment (P = 0.03, P = 0.04, respectively, n = 3; Fig. 3D and Fig. S3D†) and on day 14 by 50 μM QHREDGS (P = 0.05, n = 3; Fig. 3D and Fig. S3D†), whereas 500 μM QHREDGS treatment did not have a significant effect on expression.
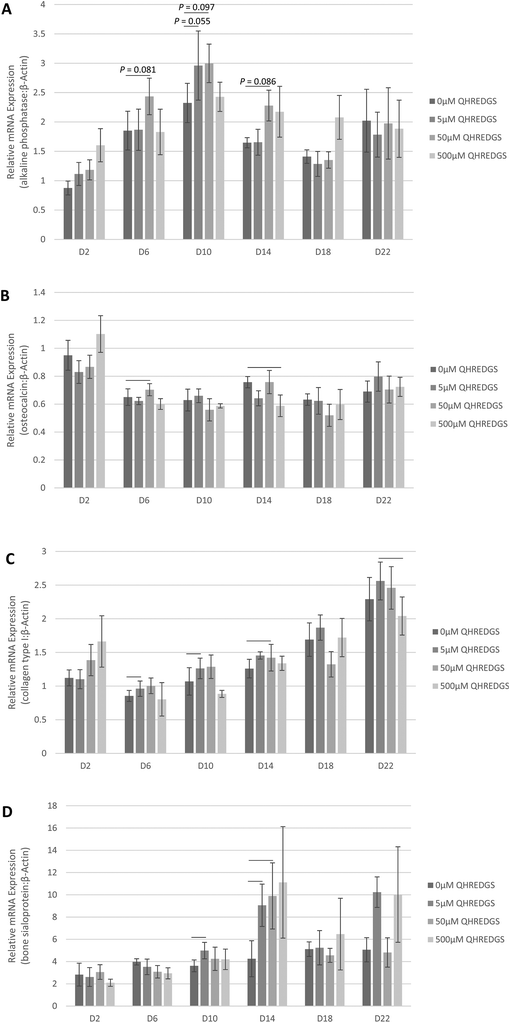 |
| Fig. 3 Osteogenic gene expression of osteoblasts grown on tissue culture plastic in the presence or absence of soluble QHREDGS on the indicated day (D): (A) alkaline phosphatase, (B) osteocalcin, (C) type I collagen and (D) bone sialoprotein. Gene expression was normalized by dividing by the expression of the housekeeping gene β-actin. Data presented are the mean ± SEM (n = 3) and the lines indicate statistical significance (P < 0.05, unless noted, n = 3). | |
3.4. QHREDGS immobilization onto PA-coated Ti promotes collagen production
Bone is a connective tissue that is predominantly comprised of an extracellular matrix (ECM) consisting of collagen, non-collagenous proteins, minerals (primarily hydroxyapatite) and water.30,31 The bone ECM produced by the MG-63 osteoblast-like cells grown on the variously coated Ti plates was assessed by Fourier transform infrared (FTIR) spectroscopy and by atomic force microscopy (AFM). The surface composition of the demineralized bone was determined by FTIR. The surface collagen concentration of the bone matrix produced on the QHREDGS-immobilized PA-coated Ti plates was significantly higher than that produced by cells grown on the uncoated Ti plates (P < 0.05, n = 4; Fig. 4A), but not statistically different from that of the other PA-coated Ti plates (P > 0.05, n = 4; Fig. 4A). The total amount of demineralized ECM produced by the cells grown on the various Ti plates was determined by calculating the elastic (Young's) moduli of the demineralized bone surfaces by AFM, with a smaller elastic modulus indicating the presence of more demineralized ECM. The average elastic modulus of the QHREDGS-immobilized PA-coated Ti surface was lower than that for all other surfaces investigated, and significantly lower than all surfaces except the uncoated and DGQESHR (scrambled)-immobilized PA-coated Ti surface (P < 0.05; n ≥ 9; Fig. 4B). Notably, the bottom 8% of elastic modulus values were derived exclusively from measurements of regions on QHREDGS-immobilized plates, values that account for approximately one-third of the total QHREDGS-immobilized plate measurements (Fig. 4B).
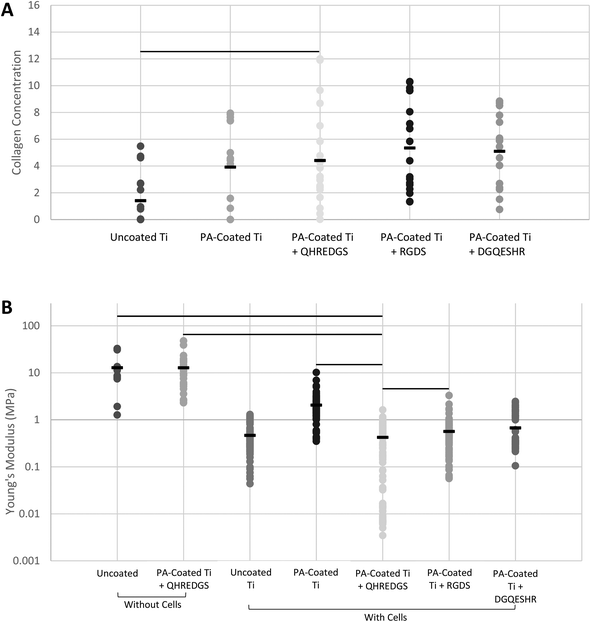 |
| Fig. 4 Bone extracellular matrix production measurements. (A) The collagen concentration of the demineralized bone matrix was determined from FTIR spectra (650–4000 cm−1) for osteoblasts grown on Ti plates without the PA-coating (Uncoated Ti), PA-coated but without any peptide conjugated (PA-coated Ti), or PA-coated with QHREDGS, RGDS or DGQESHR (scrambled) peptide conjugated. (B) The elastic (Young's) modulus of Ti plates with or without peptide immobilized and without any cells seeded on them (Without cells), and of the demineralized bone matrix laid down by the osteoblasts seeded on the various surfaces (With cells) was determined by AFM. The data presented are the individual measurements with the mean denoted by the black dash. The lines indicate statistical significance (P < 0.05; Kruskal–Wallis one-way ANOVA on ranks and Dunn's post-hoc analysis; n = 3–7). | |
3.5. QHREDGS immobilization onto PA-coated Ti and onto PEG hydrogels promotes bone mineralization
Successful bone formation is evaluated by matrix mineralization. Mineralization can be assessed by calcium and phosphate staining, with Alizarin Red S and von Kossa dyes, respectively, and by environmental scanning electron microscopy (ESEM) with a BSE detector, wherein calcium-based minerals appear as bright spots against the surrounding ECM due to their higher atomic number. For seeded Ti plates cultured in osteogenic medium and grown for a total of 34 days, we observed little to no staining on the uncoated Ti surface (Fig. 5A) and a patchy staining pattern on the PA-coated Ti without any peptide immobilized (Fig. 5B). Conversely, the QHREDGS-immobilized plate (Fig. 5C) staining was consistent throughout and more extensive than on either RGDS-immobilized plates (Fig. 5D), wherein the staining was spotty amid larger areas of consistent staining, and the DGQESHR (scrambled)-immobilized PA-coated Ti (Fig. 5E) surface that showed a very patchy pattern of staining. Quantification of the amount of Alizarin Red S stain bound to the surfaces indicated a significant increase in the amount of staining on the QHREDGS-immobilized surface relative to the uncoated, PA-coated Ti without any peptide immobilized and the DGQESHR (scrambled)-immobilized Ti surfaces (P < 0.05; n = 3; Fig. 6), as well as increased staining relative to the RGDS-immobilized surface (Fig. 6). Notably, seeded Ti plates cultured in non-osteogenic medium and grown for 22 days also showed the same pattern of Alizarin Red S and von Kossa staining, albeit mineralization was less extensive on all surfaces (Fig. S4†). Quantification of the amount of Alizarin Red S stain bound to the Ti surfaces again showed that staining was increased on the QHREDGS-immobilized Ti relative to all other surfaces (Fig. S5†). By ESEM imaging, no evidence of mineralization (bright spots) was seen on the uncoated Ti plate surface (Fig. S6A–B†). However, on the QHREDGS-immobilized PA-coated Ti plate mineralization was evident as relatively large regions (Fig. S6C–D†) that were decidedly more numerable than on the RGDS-immobilized surface wherein mineralization was visible as small granules with larger localized regions (Fig. S6E–F†).
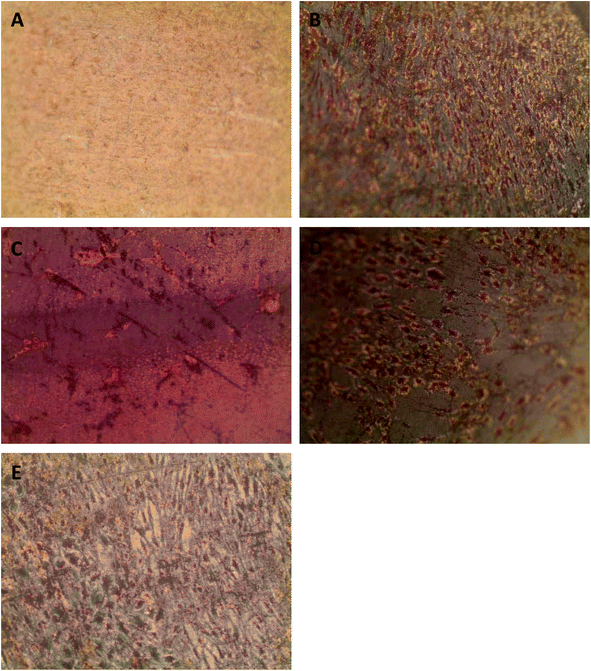 |
| Fig. 5 Representative Alizarin Red S and von Kossa staining images of Ti plates with various coatings seeded with osteoblasts and cultured in osteogenic media for 41 days. (A) Ti plates without the PA-coating. (B) PA-coated Ti plates without any peptide immobilized. (C) PA-coated Ti plates immobilized with the QHREDGS peptide. (D) PA-coated Ti plates immobilized with the RGDS peptide. (E) PA-coated Ti plates immobilized with the DGQESHR (scrambled) peptide. | |
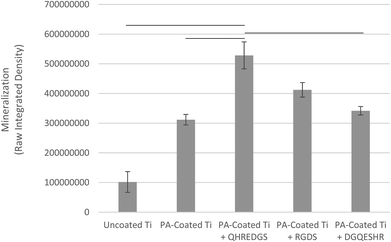 |
| Fig. 6 The amount of Alizarin Red S bound to the surface of Ti plates with various coatings seeded with osteoblasts and cultured in osteogenic media for 41 days, as determined by the sum of the pixel values in the red channel images (raw integrated density). Data presented are the mean ± SEM (n = 3) and the lines indicate statistical significance (P < 0.05; Kruskal–Wallis one-way ANOVA on ranks and Dunn's post-hoc analysis; n = 3). | |
The staining of PEG hydrogels with or without peptides immobilized showed an analogous pattern to the Ti plates for Alizarin Red S and von Kossa staining. Specifically, minimal staining was evident on the PEG hydrogel without the peptide (Fig. S7A†), while staining on the QHREDGS-immobilized hydrogels (Fig. S7B†) appeared more extensive than on either the RGDS- (Fig. S7C†) or DGQESHR (scrambled)-immobilized hydrogels (Fig. S7D†). On both the RGDS- and QHREDGS-immobilized hydrogels staining was punctate, but the stained regions on the QHREDGS-immobilized hydrogel appeared to be more closely clustered relative to the RGDS-immobilized hydrogel. Quantification of the amount of Alizarin Red S bound to the surfaces showed that the QHREDGS-immobilized surface had significantly more stain bound than both the hydrogels without any peptide immobilized and the DGQESHR (scrambled)-immobilized hydrogel surface (P < 0.05; n = 3; Fig. S8†), whereas it had a similar amount of stain bound as the RGDS-immobilized surface (Fig. S8†).
The surface composition of the bone ECM on the various PEG hydrogels was determined by FTIR and quantification of the extent of bone mineralization was achieved by calculating the mineral-to-matrix ratio (M/M ratio).32–35 The surface collagen (matrix) concentration of the bone ECM generated by the cells on the hydrogels was found to be lower on average for the QHREDGS-immobilized hydrogel relative to all other hydrogels but not significantly different (P > 0.05; n = 4; Fig. 7A). Conversely, the phosphate (mineral) concentration on the hydrogel surface was higher on average for the QHREDGS-immobilized hydrogel than for any other hydrogel surface but once again this difference was not statistically different (P > 0.05; n = 4; Fig. 7B). However the M/M ratio of the QHREDGS-immobilized hydrogel significantly increased relative to all other surfaces (P < 0.05; n = 4; Fig. 7C). The significant increase in bone mineralization was not a consequence of the increased cell number because while the number of cells on the QHREDGS-immobilized hydrogel was more than that on both the PEG hydrogel without any peptide immobilized and the DGQESHR-immobilized hydrogel, it was less than on the RGDS-immobilized hydrogel (P > 0.05; n = 4; Fig. S6†), and yet bone mineralization was more extensive for the QHREDGS-immobilized hydrogel than any other surface.
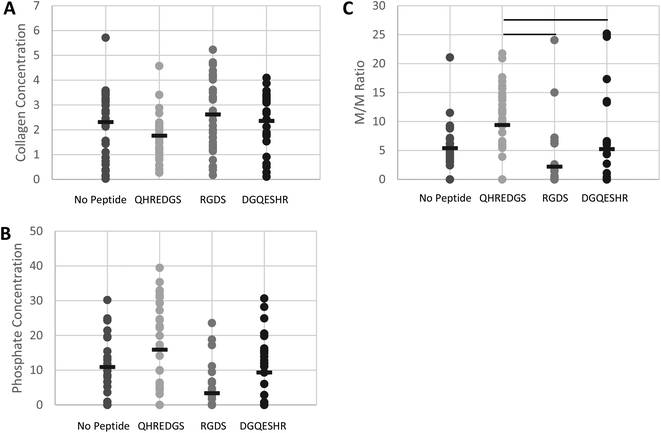 |
| Fig. 7 The composition of the bone matrix produced by osteoblasts cultured on PEG-based hydrogels without peptide conjugated (No peptide), or with either the QHREDGS, RGDS or DGQESHR (scrambled) peptide conjugated determined by FTIR. (A–B) The collagen (matrix) and phosphate (mineral) concentrations of the bone matrix were determined from the FTIR spectra (650–4000 cm−1). (C) The mineral-to-matrix (M/M) ratio was also calculated. The data presented are the individual measurements with the mean denoted by the black dash. The lines indicate statistical significance (P < 0.05; Kruskal–Wallis one-way ANOVA on ranks and Dunn's post-hoc analysis; n = 4). | |
4. Discussion
With the use of standard treatment techniques, there still remains a substantial fraction of bone defects that do not heal properly.7 Small animal models have demonstrated the potential of gene therapy and stem/progenitor cell transplantation; however major safety and efficacy concerns need to be addressed before their clinical application.36,37 Thus, much effort has been directed alternatively in designing biomaterials for the controlled delivery of osteoinductive factors in order to produce a highly localized tissue response.4 Herein we have demonstrated that the small Ang-1-derived peptide QHREDGS can be covalently immobilized onto both PA-coated Ti and PEG hydrogels through EDC/NHS-based chemistry (Fig. 1), thereby localizing the effect of the peptide to the surface of the medical device/biomaterial. The use of QHREDGS in bone therapy applications is advantageous in that its conjugation is amenable to different biomedical materials, which we demonstrated by covalently immobilizing QHREDGS to two very different surface types using the ubiquitous chemistry of EDC/NHS. Additionally, since the functionality of the QHREDGS peptide is not dependent upon any particular conformation or orientation, there is flexibility in the conjugation methods that can be used for immobilization, further expanding the variety of contexts in which QHREDGS can be used. Interestingly, the effective concentration of QHREDGS seems to be relatively low since high concentrations (500 μM) were observed to downregulate the osteogenic genes, collagen type I and osteocalcin (Fig. 3 and S3†). Finally, as a small 7-amino acid peptide, QHREDGS can be easily and cost-effectively synthesized, and can withstand a higher degree of manipulation and a wider range of conditions than a full-length protein.
Moreover, we have demonstrated that QHREDGS is osteoinductive, promoting osteoblast differentiation along with both bone matrix deposition and mineralization. Osteoblast differentiation is oftentimes marked by the activity of ALP, an enzyme secreted in increasing amounts during the second phase of osteoblast differentiation, matrix deposition,38 with levels peaking before the cells transition into the third stage of differentiation, matrix mineralization.39 The ALP activity of osteoblast-like cells cultured on QHREDGS-immobilized Ti plates peaked 4–12 days earlier than on the unimmobilized, DGQESHR (scrambled)- or RGDS-immobilized surfaces, with all the surfaces ultimately achieving the same maximum ALP activity (Fig. 2). This is indicative of an accelerated rate of osteoblast differentiation on the QHREDGS-immobilized surface but not of increased differentiation overall. Similarly, we also observed that treatment of osteoblasts with soluble QHREDGS induced the upregulation of osteogenic genes (Fig. 3 and Fig. S3†). The time required for bone regeneration is a critical factor for successful bone therapy; furthermore decreased healing time reduces a patient's recovery time, i.e. their discomfort, stress and economic costs, but also reduces the risk of complications.40 Hence, by accelerating the rate of osteoblast differentiation, QHREDGS may also improve bone regeneration.
Much like the ALP activity, the bone composition can be used to make assumptions about the formation of bone. Bone strength is determined by both the bone quantity and quality;41 and among the factors that dictate the bone quality are toughness, the domain of the collagen fibers tasked with providing the properties of ductility and energy absorption, as well as stiffness, determined by the mineral phase.42 Osteoblast-like cells cultured on the QHREDGS-immobilized Ti produced a bone matrix that when demineralized did not differ significantly in the relative surface composition among the various PA-coated Ti surfaces (Fig. 4A) but had a significantly lower elastic modulus than produced on the other surfaces (Fig. 4B), indicating that QHREDGS-immobilization produced quantitatively more bone matrix compared to the other surfaces. The elastic modulus of demineralized bone is positively correlated with the bone strength, work-to-fracture and toughness;43 however, in this context the significant reduction in local elastic modulus denotes a change from the stiff Ti surface to a surface coated with extracellular matrix proteins. Importantly, this change in elastic modulus describes a change in localized mechanical properties, whereas if a bulk test of mechanical properties were to be performed, the elastic modulus would still be governed by the Ti plate itself. Currently, Ti-based scaffolds are being designed with elastic moduli approximating that of bone,44 a value that varies from 4 to 30 GPa depending on the type of bone measured and the measurement direction.45 This is because a biomechanical mismatch wherein the implant has a higher stiffness than bone can result in “stress shielding”—the inhibition of stress transfer to the adjacent bone—that can result in bone resorption around the implant and ultimately in implant loosening and failure.46 In order to take advantage of the mechanical strength of the device while at the same time allowing the effective communication of the cells with their surroundings, it is thought that an ideal implant would precisely combine high strength with a low modulus closer to the bone.47 As such, based on our findings, QHREDGS-immobilization can generate a low elastic modulus environment on the implant surface that promotes osteoblast differentiation and bone production without affecting the overall strength of the device, and thereby has the potential to reduce the risk of implant failure.
The relative surface composition of the mineralized bone matrix produced on the QHREDGS-immobilized PEG hydrogels was measured to be lower in collagen content than the other hydrogel surfaces (Fig. 7A). While seemingly contradictory, an inverse relationship between the collagen content and the degree of mineralization has been reported,48 and in fact we observed that the QHREDGS-immobilized hydrogel surface also had more mineral content than the other hydrogel surfaces as this inverse relationship would predict (Fig. 7B). Moreover, it is quite likely that the collagen content on the QHREDGS-immobilized surface was measured to be lower because the matrix was covered by a mineral layer and FTIR is a technique that measures the surface composition, as IR light penetration is on the order of micrometers during ATR measurements. Given the high mineral and low collagen (matrix) measurements for the QHREDGS-immobilized surface, it is unsurprising that the resulting mineral-to-matrix (M/M) ratio was significantly higher for the QHREDGS-immobilized surface than for the other surfaces (Fig. 7C). The M/M ratio by definition indicates the relative amount of the mineral and matrix, and is used as a measure of bone mineralization.32 Notably, there is a positive correlation between the M/M ratio and total bone mineral density, load (force or moment) to failure and bone stiffness.41 Thus, QHREDGS-immobilization induces bone mineralization to a greater degree than the other surfaces. This fact was also evident in our imaging data wherein both the QHREDGS- and RGDS-immobilized surfaces had extensive mineral staining that was quantified to be increased on the QHREDGS-immobilized Ti surface, both when osteoblasts were induced to differentiate (Fig. 5 and 6) and when they were not (Fig. S4–5†), and quantified to be equivalent on the PEG-immobilized surfaces (Fig. S7–8†). Taken together, these results demonstrate that QHREDGS-immobilization induces matrix deposition and its subsequent mineralization, and suggest that QHREDGS can induce the production of better quality bone.
While QHREDGS is a relatively new bioactive molecule, and an entirely novel factor for bone regenerative therapy, the RGD peptide has long been investigated in the context of both titanium49–51 and hydrogels.52,53 Herein we have demonstrated the improved effectiveness of QHREDGS over RGDS with respect to osteoblast differentiation, bone matrix deposition and mineralization. This indicates the potential for improved therapeutic results with the QHREDGS peptide over the RGD peptide, and for pushing forward research into this novel factor by substitution of one peptide by the other in existing therapies given the similarities between RGD and QHREDGS. Although, it is unlikely that QHREDGS will improve bone regeneration success rates to the required levels on its own, its improved osteoinductive properties can provide an incremental step forward. As new medical devices/biomaterials and approaches are developed the QHREDGS peptide can be used to supplement these new technologies with minimal necessary adjustments given its manipulability and flexibility.
In terms of a possible mechanism, QHREDGS has been reported to bind to β1-type integrins with specific interactions noted between the peptide and α5β1 and αvβ3 integrin receptors;15 and β1-integrins have been identified as playing a significant role in osteoblast differentiation, mineralization in vitro and bone formation in vivo.9,11 Herein, we demonstrate that the QHREDGS peptide affects the in vitro processes of matrix deposition and mineralization, and osteoblast differentiation. This may suggest that QHREDGS affects these processes through a β1-integrin-mediated pathway. Recently, knockdown of the β1-integrin binding partner integrin-linked kinase (ILK) was shown to result in increased differentiation and bone mineralization by downregulating c-JUN-dependent transcription.54 Conversely, activation of the extracellular signal-related kinase (ERK1/2) pathway downstream of ILK has been reported to promote differentiation and bone mineralization.55,56 The precise molecular mechanisms involved in improved bone mineralization by QHREDGS is therefore unclear and will be the subject of future work.
5. Conclusions
We have demonstrated for the first time that the small Ang-1-derived peptide QHREDGS accelerates osteoblast differentiation and promotes both bone matrix deposition and mineralization more effectively than the common integrin-binding peptide RGDS, when immobilized onto either titanium medical devices or biomaterials. The acceleration of the processes of osteoblast differentiation, matrix deposition and bone mineralization is an important factor influencing bone therapy as the time needed to regenerate bone affects not only the ultimate success of the treatment but also the recovery time of the patient. Additionally, the ability of QHREDGS-immobilization to modify the localized mechanical properties of implant devices without affecting their global mechanical properties has the potential to reduce the risk of implant failure. Finally, we have demonstrated that the quality of the bone produced by the QHREDGS-immobilized surfaces was improved relative to the other surfaces investigated. Hence, the novel peptide QHREDGS may be a suitable candidate for tests in preclinical models.
Abbreviations
Ang-1 | Angiopoietin-1 |
PA | Polyacrylate |
Ti | Titanium |
PEG | Polyethylene glycol |
M/M | Mineral-to-matrix |
EDC | Ethyl(dimethylaminopropyl) carbodiimide |
NHS | Sulfo-N-hydroxysuccinimide |
ALP | Alkaline phosphatase |
LDH | Lactate dehydrogenase |
ECM | Extracellular matrix |
FTIR | Fourier transform infrared spectroscopy |
AFM | Atomic force microscopy |
ESEM | Environmental scanning electron microscopy |
ILK | Integrin-linked kinase |
ERK1/2 | Extracellular signal-related kinase. |
Acknowledgements
To Michael Sefton, a great leader, visionary and a truly outstanding mentor.
This work was funded by grants from the Natural Sciences and Engineering Research Council of Canada (NSERC) Strategic Grant (STPGP 381002-09), Ontario Research Fund–Global Leadership Round 2 (ORF-GL2), the Canadian Institutes of Health Research (CIHR) Operating Grant (MOP-126027), NSERC-CIHR Collaborative Health Research Grant (CHRPJ 385981-10), NSERC Discovery Grant (RGPIN 326982-10), NSERC Discovery Accelerator Supplement (RGPAS 396125-10), National Institutes of Health Grant (2R01 HL076485) and Heart and Stroke Foundation Grant (T6946). Electron microscopy was performed at the University of Toronto, Faculty of Medicine, Microscopy Imaging Lab with the help of Battista Calvieri. FTIR was performed at the University of Toronto, Analytical Lab for Environmental Science Research and Training (ANALEST) facility. The graphical abstract was designed by Boyang Zhang.
Notes and references
- R. J. Miron and Y. F. Zhang, J. Dent. Res., 2012, 91, 736–744, DOI:10.1177/0022034511435260.
- D. G. Anderson, J. A. Burdick and R. Langer, Science, 2004, 305, 1923–1924, DOI:10.1126/science.1099987.
- W. Singhatanadgit, Bone Tissue Regener. Insights, 2009, 2, 1–11 CAS.
- P. S. Lienemann, M. P. Lutolf and M. Ehrbar, Adv. Drug Delivery Rev., 2012, 64, 1078–1089, DOI:10.1016/j.addr.2012.03.010.
- E. D. Arrington, W. J. Smith, H. G. Chambers, A. L. Bucknell and N. A. Davino, Clin. Orthop. Relat. Res., 1996, 329, 300–309 Search PubMed.
- R. C. Sasso, J. I. Williams, N. Dimasi and P. R. Meyer Jr., J. Bone Joint Surg. Am., 1998, 80, 631–635 CrossRef CAS PubMed.
- G. B. Bishop and T. A. Einhorn, Int. Orthop., 2007, 31, 721–727, DOI:10.1007/s00264-007-0424-8.
- M. Geiger, R. H. Li and W. Friess, Adv. Drug Delivery Rev., 2003, 55, 1613–1629 CrossRef CAS PubMed.
- P. J. Marie, Nat. Rev. Endocrinol., 2013, 9, 288–295, DOI:10.1038/nrendo.2013.4.
- Y. Takada, X. Ye and S. Simon, Genome Biol., 2007, 8, 215, DOI:10.1186/gb-2007-8-5-215.
- G. B. Schneider, R. Zaharias and C. Stanford, J. Dent. Res., 2001, 80, 1540–1544 CrossRef CAS PubMed.
- S. Davis, T. H. Aldrich, P. F. Jones, A. Acheson, D. L. Compton, V. Jain, T. E. Ryan, J. Bruno, C. Radziejewski, P. C. Maisonpierre and G. D. Yancopoulos, Cell, 1996, 87, 1161–1169 CrossRef CAS.
- T. R. Carlson, Y. Feng, P. C. Maisonpierre, M. Mrksich and A. O. Morla, J. Biol. Chem., 2001, 276, 26516–26525, DOI:10.1074/jbc.M100282200.
- S. M. Dallabrida, N. Ismail, J. R. Oberle, B. E. Himes and M. A. Rupnick, Circ. Res., 2005, 96, e8–e24, DOI:10.1161/01.RES.0000158285.57191.60.
- J. W. Miklas, S. M. Dallabrida, L. A. Reis, N. Ismail, M. Rupnick and M. Radisic, PLoS One, 2013, 8, e72956, DOI:10.1371/journal.pone.0072956.
- F. Rask, S. M. Dallabrida, N. S. Ismail, Z. Amoozgar, Y. Yeo, M. A. Rupnick and M. Radisic, J. Biomed. Mater. Res., Part A, 2010, 95, 105–117, DOI:10.1002/jbm.a.32808.
- L. Mazzola, E. Bemporad, C. Misiano, F. Pepe, P. Santini and R. Scandurra, J. Nanosci. Nanotechnol., 2011, 11, 8754–8762 CrossRef CAS PubMed.
- D. G. Olmedo, D. R. Tasat, G. Duffo, M. B. Guglielmotti and R. L. Cabrini, Acta Odontol. Latinoam., 2009, 22, 3–9 Search PubMed.
- R. Dimitriou, E. Jones, D. McGonagle and P. V. Giannoudis, BMC Med., 2011, 9, 66, DOI:10.1186/1741-7015-9-66.
- E. De Giglio, D. Cafagna, M. A. Ricci, L. Sabbatini, S. Cometa, C. Ferretti and M. Mattioli-Belmonte, J. Bioact. Compat. Polym., 2010, 25, 374–391, DOI:10.1177/0883911510372290.
- R. H. Li and J. M. Wozney, Trends Biotechnol., 2001, 19, 255–265 CrossRef CAS.
- J. O. Hollinger, J. M. Schmitt, D. C. Buck, R. Shannon, S. P. Joh, H. D. Zegzula and J. Wozney, J. Biomed. Mater. Res., 1998, 43, 356–364 CrossRef CAS.
- E. J. Carragee, E. L. Hurwitz and B. K. Weiner, Spine J., 2011, 11, 471–491, DOI:10.1016/j.spinee.2011.04.023.
- R. Ravichandran, C. C. Ng, S. Liao, D. Pliszka, M. Raghunath, S. Ramakrishna and C. K. Chan, Biomed. Mater., 2012, 7, 015001, DOI:10.1088/1748-6041/7/1/015001.
- S. S. Nunes, J. W. Miklas, J. Liu, R. Aschar-Sobbi, Y. Xiao, B. Zhang, J. Jiang, S. Masse, M. Gagliardi, A. Hsieh, N. Thavandiran, M. A. Laflamme, K. Nanthakumar, G. J. Gross, P. H. Backx, G. Keller and M. Radisic, Nat. Methods, 2013, 10, 781–787, DOI:10.1038/nmeth.2524.
- W. Zhang, Z. Li and B. Peng, J. Endod., 2010, 36, 1978–1982, DOI:10.1016/j.joen.2010.08.038.
- H. K. Kim, M. G. Kim and K. H. Leem, Food Funct., 2014, 5, 573–578, 10.1039/c3fo60509d.
- G. Harrison, I. M. Shapiro and E. E. Golub, J. Bone Miner. Res., 1995, 10, 568–573, DOI:10.1002/jbmr.5650100409.
- K. M. Holtz and E. R. Kantrowitz, FEBS Lett., 1999, 462, 7–11 CrossRef CAS.
- R. Fujisawa and M. Tamura, Front. Biosci., 2012, 17, 1891–1903 CrossRef CAS PubMed.
- C. Nicolaije, M. Koedam and J. P. van Leeuwen, J. Cell. Physiol., 2012, 227, 1309–1318, DOI:10.1002/jcp.22841.
- E. P. Paschalis, E. DiCarlo, F. Betts, P. Sherman, R. Mendelsohn and A. L. Boskey, Calcif. Tissue Int., 1996, 59, 480–487 CrossRef CAS.
- E. P. Paschalis, R. Mendelsohn and A. L. Boskey, Clin. Orthop. Relat. Res., 2011, 469, 2170–2178, DOI:10.1007/s11999-010-1751-4.
- S. Takata, A. Shibata, H. Yonezu, T. Yamada, M. Takahashi, A. Abbaspour and N. Yasui, J. Med. Invest., 2004, 51, 133–138 CrossRef.
- P. Weiss, S. Bohic, M. Lapkowski and G. Daculsi, J. Biomed. Mater. Res., 1998, 41, 167–170 CrossRef CAS.
- J. Fischer, A. Kolk, S. Wolfart, C. Pautke, P. H. Warnke, C. Plank and R. Smeets, J. Craniomaxillofac. Surg., 2011, 39, 54–64, DOI:10.1016/j.jcms.2010.03.016.
- R. E. Guldberg, J. Bone Miner. Res., 2009, 24, 1507–1511, DOI:10.1359/jbmr.090801.
- B. Kulterer, G. Friedl, A. Jandrositz, F. Sanchez-Cabo, A. Prokesch, C. Paar, M. Scheideler, R. Windhager, K. H. Preisegger and Z. Trajanoski, BMC Genomics, 2007, 8, 70, DOI:10.1186/1471-2164-8-70.
- G. S. Stein, J. B. Lian and T. A. Owen, FASEB J., 1990, 4, 3111–3123 CAS.
- G. Victoria, B. Petrisor, B. Drew and D. Dick, Indian J. Orthop., 2009, 43, 117–120, DOI:10.4103/0019-5413.50844.
- S. Takata, H. Yonezu, A. Shibata, T. Enishi, N. Sato, M. Takahashi, S. Nakao, K. Komatsu and N. Yasui, J. Med. Invest., 2011, 58, 197–202 CrossRef.
- S. Viguet-Carrin, P. Garnero and P. D. Delmas, Osteoporos. Int., 2006, 17, 319–336, DOI:10.1007/s00198-005-2035-9.
- X. Wang, X. Shen, X. Li and C. M. Agrawal, Bone, 2002, 31, 1–7 CrossRef.
- J. Li, H. Yang, H. Wang and J. Ruan, Mater. Sci. Eng., C. Mater. Biol. Appl., 2014, 34, 110–114, DOI:10.1016/j.msec.2013.08.043.
- J. L. Katz, Nature, 1980, 283, 106–107 CrossRef CAS.
- D. R. Sumner, T. M. Turner, R. Igloria, R. M. Urban and J. O. Galante, J. Biomech., 1998, 31, 909–917 CrossRef CAS.
- M. Geetha, A. K. Singh, R. Asokamani and A. K. Gogia, Progress Mater. Sci., 2009, 54, 397–425 CrossRef CAS PubMed.
- J. Aerssens, S. Boonen, G. Lowet and J. Dequeker, Endocrinology, 1998, 139, 663–670 CAS.
- D. M. Ferris, G. D. Moodie, P. M. Dimond, C. W. Gioranni, M. G. Ehrlich and R. F. Valentini, Biomaterials, 1999, 20, 2323–2331 CrossRef CAS.
- H. Schliephake, D. Scharnweber, M. Dard, S. Rossler, A. Sewing, J. Meyer and D. Hoogestraat, Clin. Oral Implants Res., 2002, 13, 312–319 CrossRef.
- B. Elmengaard, J. E. Bechtold and K. Soballe, J. Biomed. Mater. Res., Part A, 2005, 75, 249–255, DOI:10.1002/jbm.a.30301.
- E. Alsberg, K. W. Anderson, A. Albeiruti, R. T. Franceschi and D. J. Mooney, J. Dent. Res., 2001, 80, 2025–2029 CrossRef CAS PubMed.
- J. A. Burdick and K. S. Anseth, Biomaterials, 2002, 23, 4315–4323 CrossRef CAS.
- J. El-Hoss, A. Arabian, S. Dedhar and R. St-Arnaud, Gene, 2014, 533, 246–252, DOI:10.1016/j.gene.2013.09.074.
- Y. H. Choi, Y. M. Gu, J. W. Oh and K. Y. Lee, Biochem. Biophys. Res. Commun., 2011, 415, 472–478, DOI:10.1016/j.bbrc.2011.10.097.
- O. Wanachewin, K. Boonmaleerat, P. Pothacharoen, V. Reutrakul and P. Kongtawelert, BMC Complement. Altern. Med., 2012, 12, 71, DOI:10.1186/1472-6882-12-71.
Footnote |
† Electronic supplementary information (ESI) available. See DOI: 10.1039/c4bm00073k |
|
This journal is © The Royal Society of Chemistry 2014 |
Click here to see how this site uses Cookies. View our privacy policy here.