DOI:
10.1039/D4AN01109K
(Minireview)
Analyst, 2024,
149, 5156-5164
Current trends in electrochemical approaches for liver biomarker detection: a mini-review
Received
18th August 2024
, Accepted 20th September 2024
First published on 23rd September 2024
Abstract
Aspartate aminotransferase (AST), alanine aminotransferase (ALT), bilirubin, gamma-glutamyl transferase (GGT), alkaline phosphatase (ALP), and albumin are well-established liver biomarkers with significant physiological functions. Alterations in these liver function tests can be indicative of the presence and progression of acute and chronic liver conditions such as liver cirrhosis, non-alcoholic fatty liver disease, biliary disease, and liver failure. Therefore, accurate and quantitative detection of these biomarkers is crucial for diagnosing and monitoring liver disease. There are several commercially available chemistry analyzers capable of simultaneously detecting all these biomarkers, as well as numerous biosensors designed for individual detection. Various techniques have been employed, including colorimetry, surface-enhanced Raman spectroscopy (SERS), electrochemiluminescence (ECL), fluorescence-based techniques, and electrochemical methods. Among these, electrochemical detection stands out due to its simplicity, cost-effectiveness, low sample volume requirement, label-free detection, high sensitivity, fast response times, miniaturization, and portability. Information on recently developed electrochemical biosensors is summarized through detailed tables and is intended to guide future research and development efforts in this area.
1. Introduction
Liver disease is a challenging health problem that poses a significant burden on individuals, healthcare systems, and economies worldwide.1 Mortality rates, prolonged hospitalization periods, and increasing treatment expenses associated with liver diseases highlight the critical need for improved diagnostic tools and treatment strategies. Therefore, it is crucial to monitor liver biomarkers, which play a significant role in assessing liver function, early diagnosis (and therefore early treatment), and preventing the progression of various hepatic disorders. For the diagnostic process to be effective, biomarker detection must be rapid and cost-effective.
The liver serves as a vital organ responsible for numerous physiological functions, including metabolism, detoxification, and protein synthesis.2 Any deterioration in liver function can have far-reaching consequences for overall health. Common examples include several diseases of diverse aetiology: metabolic dysfunction-associated steatotic liver disease (MASLD), paracetamol overdose, primary biliary cirrhosis and hepatocellular carcinoma among others. All of these require assessment of liver function tests for diagnostic purposes, assessing progression, deciding course of treatment and defining clinical outputs. Proteins and enzymes commonly measured in clinical practice and whose blood levels reflect various aspects of liver function and integrity can be listed as aspartate aminotransferase (AST), alanine aminotransferase (ALT), bilirubin, gamma-glutamyl transferase (GGT), alkaline phosphatase (ALP) and albumin.3
Traditional biomarker detection methods such as enzyme-linked immunosorbent assay (ELISA) and chromatography are often expensive, time-consuming, and labour-intensive. Therefore, researchers have turned to alternative detection strategies such as colorimetry,4,5 surface-enhanced Raman spectroscopy (SERS),6,7 electrochemiluminescence (ECL),8,9 fluorescence-based techniques,10,11 and electrochemical platforms.12,13 Colorimetric detection is based on the measurement of colour change during chemical reactions and offers a simple approach to biomarker measurement.4 SERS enables sensitive and selective detection of bio-analytes by taking advantage of enhanced Raman signals produced by molecules adsorbed to SERS-active substrates, often without any modification or labelling.7 ECL biosensors provide a sensitive sensing platform with low background noise by utilizing electrochemical reactions to produce luminescent signals.8 Fluorescence-based sensing techniques enable rapid and quantitative detection of analytes using their fluorescence properties.10,11 All these techniques have their relative advantages and disadvantages.
Although various techniques have been used for the detection of liver biomarkers, electrochemical methods stand out due to their associated performance advantages which include high sensitivity, fast response times, simplicity, portability, cost-effectiveness, and potential for multiplexing. Basically, electrochemical sensors are systems designed to selectively detect specific chemical components in a sample by converting chemical information into an analytically useful signal. The target analyte interacts with a specific chemical interface layer, resulting in a chemical change. This change is then converted into a readable signal by a physical transducer. The fundamental principle of these sensors is based on electrochemistry,14 a field that studies electron transfer at the solution/electrode surface through oxidation and reduction reactions. These reactions are monitored by either applying a potential difference between the electrodes and measuring the resulting current or by measuring changes in potential under a constant current. In this way, analyte detection and concentration measurements are achieved with minimal sample preparation. In addition, basic electrochemical theory and application has been well covered in these previous review articles.15,16
While electrochemical sensors meet the need for fast and cost-effective diagnostic solutions, enabling the quantitative detection of AST, ALT, GGT, ALP, bilirubin, and albumin with high sensitivity and selectivity, significant progress has primarily focused on individual biomarkers. However, there remains a notable gap in comprehensive assessment tools that can simultaneously monitor multiple biomarkers to provide a holistic view of liver function. Although Song et al. designed an electrochemical sensor that simultaneously detects AST/ALT, bilirubin, and cholesterol,17 existing electrochemical sensors generally focus on a single biomarker, limiting their usefulness in accurately diagnosing hepatic disorders. Addressing this limitation and developing a multiplexed detection platform capable of analysing a panel of liver biomarkers in a single assay requires further research and innovation in electrochemical sensing. Such advances will enable clinicians to obtain rapid and comprehensive information about liver function and disease status, facilitating diagnostic and treatment decisions and improving patient outcomes.
In this review, initially, the roles of common liver biomarkers function and their relationships with various disorders are briefly introduced. Then, information about recently developed electrochemical sensors for these biomarkers and electrode sensors used for detection is presented and summarised.
2. Overview of common liver biomarkers
AST, ALT, GGT, ALP, bilirubin, and albumin provide valuable information regarding liver health and pathology. ALT is an enzyme found primarily in the liver, and to a lesser extent in the kidneys and skeletal muscle,3 and its function as a biological catalyst is to produce pyruvate and glutamate by catalysing the transfer of an amino group from alanine to α-ketoglutarate.18 The normal concentration range of ALT in human blood in homeostasis is between 5 to 35 U L−1 (ref. 18) and abnormal levels have been associated with hepatocellular injury.3
AST, on the other hand, is an enzyme found in various organs including the liver, pancreas, lungs, red blood cells, and muscle tissue.3 Like ALT, AST catalyses the transfer of an amino group from aspartate to α-ketoglutarate, forming oxaloacetate and glutamate.18 The normal range of AST in human blood during homeostasis is 5 to 40 U L−1 (ref. 18) and abnormal levels of it have been associated with hepatocellular injury as well as hemolysis.3 Although it is less specific to the liver compared to ALT, it serves as a significant marker of parenchymal damage.
ALP, another enzyme crucial in liver metabolism, is found in the liver, kidneys, bone, placenta, and intestines3 and its main function is to catalyse the dephosphorylation of phosphate esters in various phosphorylated species.19 The normal range of ALP activity in the blood of adults is between 40 to 190 U L−1,19 and abnormal levels (>190 U L−1) have been linked to cholestatic liver disease including primary sclerosing cholangitis, primary biliary cirrhosis and biliary complications.3
Bilirubin, a yellowish-orange pigment produced by the liver, has a serum concentration of less than 0.3 mg dL−1.20 Increased bilirubin levels in sera can result in jaundice and additional complications (with risk of death from liver failure significantly increased when the total bilirubin level exceeds 20 mg dL−1).20
GGT is an enzyme found in the biliary epithelial cells, pancreas, prostate, and kidney3 and it contributes to glutathione (GSH) and cysteine metabolism by removing gamma-glutamyl group from GSH and similar molecules.21 In homeostasis, GGT in adult blood ranges between 6–50 U L−1 (ref. 22) and its elevation has been associated with biliary disease.3
Finally, albumin which is synthesized by liver hepatocytes and is the most abundant protein in the body, plays a role in maintaining osmotic pressure and transporting various substances such as drugs and hormones.23 Abnormal levels of albumin, whose concentration in blood plasma is approximately 35–50 mg mL−1, have been linked to liver cirrhosis, and liver failure.24
3. Existing electrochemical assays for common liver biomarkers
Examining Table 1 reveals the diversity of electrochemical detection methodologies of liver biomarkers with varying detection limits for common liver biomarkers, various electrode configurations, and electrochemical techniques used. The lowest LODs (limit of detection) obtained for ALT, AST, ALP, bilirubin, albumin, and GGT were recorded as 0.16 U L−1, 20 U L−1, 1.48 mU mL−1, 7.80 fM, 5.4 × 10−10 μg mL−1, and 0.38 U L−1, respectively. It also appears that electrode construction or the electrochemical techniques used do not follow any strict patterns. For instance, ALT detection was successfully performed using four different electrode constructions such as thin-film electrode (TFE), screen printing electrode (SPE), molecularly imprinted polymer (MIP)-based electrode, and composite electrodes, and four different electrochemical techniques such as square wave voltammetry (SWV), cyclic voltammetry (CV), differential pulse voltammetry (DPV), and amperometry. The electrochemical technique to be applied is selected through consideration of a combination of practical constraints, the requirements of the study, and the expertise of the researchers. Each study took advantage of a different electrochemical technique or electrode construction. As a result, various approaches have been adopted for the detection of common liver biomarkers, and some of which have been recently developed are summarized in Table 1.
Table 1 Electrochemical measurement of liver biomarkers
Electrode |
Methods |
Analyte(s) detected |
Sample |
LOD |
Linear range |
Sensitivity |
Ref. |
NR = Not Reported. |
POx/graphene@MXeme/GCE |
SWV |
ALT |
Human serum |
0.16 U L−1 |
0.5–400 U L−1 |
12.7 nA (U L−1 mm2)−1 |
25
|
POx MIP-modified PME |
CV |
ALT |
Human Serum |
2.97 U L−1 |
25–700 U L−1 |
NR |
26
|
Platinum TFE |
DPV |
ALT |
Commercial ALT |
NR |
5–120 U L−1 |
NR |
27
|
Prussian-blue modified screen-printed carbon electrodes |
Amperometry |
ALT |
Commercial ALT |
20.6 U L−1 |
40–1990 U L−1 |
1.53 nA (U L−1 mm2)−1 |
28
|
Screen-printable Ir–C electrode |
Amperometry |
AST |
Human serum |
25.3 U L−1 |
0–250 U L−1 |
18.91 nA (μg mL−1)−1 |
29
|
Multienzyme-modified gold WE, platinum CE, and an external Ag/AgCl RE |
CV |
AST–ALT |
Human plasma |
20 U L−1 for AST |
30–240 U L−1 for AST |
NR |
30
|
25 U L−1 for ALT |
43–480 U L−1 for ALT |
CoOOH/TCPB-DMTA-COF/GCE |
DPV |
ALP |
Commercial ALP |
6.0 × 10−3 U L−1 |
0.01–100 U L−1 |
NR |
31
|
ssDNA-modified gold WE, platinum wire CE, and saturated calomel RE |
DPV |
ALP |
Human serum |
1.48 mU mL−1 |
20–100 mU mL−1 |
NR |
32
|
SPCE/AuNPs/Au-nano Dendroids/GO/anti-ALP |
EIS |
ALP |
Human serum |
9.10 (±0.12) U L−1 |
dynamic range 100–1000 U L−1 |
NR |
33
|
GCE/NC |
CV |
Bilirubin |
Commercial Bilirubin |
0.69 μM |
2–162 μM |
0.2 μA μM−1 cm−2 |
20
|
BOx/GO@PANI/ITO |
Amperometry |
Bilirubin |
Human serum |
0.01 μM |
0.01–250 μM |
0.905 μA μm−1 |
34
|
Enzyme/Ag-NPs/PBs |
CV and LSV |
Bilirubin |
Artificial Human serum |
1 μg mL−1 |
1–9 μg mL−1 |
NR |
35
|
OPD/MWCNT/GCE |
DPV |
Bilirubin |
Infant serum and saliva |
7.80 fM |
12.08 fM to 91.81 fM |
1.05 μA fM−1 |
36
|
TDMAC/DOP/MWCNT |
EIS |
Albumin |
Human serum |
4.3 × 10−8 M |
5.2 × 10−8–1.0 × 10−4 M |
NR |
37
|
TDMAC/DOP/MWCNT |
Potentiometry |
Albumin |
Human serum |
0.8 μM |
1.5 μM to 1.5 mM |
NR |
37
|
CS/CuO NPs/ERGO/GCE |
DPV |
Albumin |
Human serum |
2.6 ng mL−1 |
10–450 ng mL−1 |
NR |
38
|
antiHSA/AuNPs/PpPD/PEDOT:PSS-Fc/SPCE |
DPV |
Albumin |
Human serum and Urine |
5.4 × 10−10 μg mL−1 |
1.0 × 10−9–1.0 × 10−2 μg mL−1 |
NR |
39
|
GE/AuNPs/PTH-MB/MIP |
DPV |
Albumin |
Human urine |
3 × 10−11 g L−1 |
1.0 × 10−10–1.0 × 10−4 g L−1 |
NR |
13
|
GTLPOH probe |
DPV |
GGT |
Whole blood and urine |
0.38 U L−1 |
2–100 U L−1 |
NR |
12
|
GSH modified gold WE, platinum wire CE, saturated calomel RE |
CV and EIS |
GGT |
Commercial GGT |
NR |
NR |
NR |
40
|
Thin-film electrode (platinum WE and CE, Ag/AgCl RE) |
Amperometry |
GGT |
Commercial Human serum |
NR |
35–659 U L−1 |
NR |
41
|
4. Construction and application of liver biomarker-specific electrodes
Various electrode constructions are used in biosensors due to the different requirements of applications such as sensitivity, selectivity, and stability. For example, enzymes, antibodies, or molecularly imprinted polymers are used as recognition elements in biosensors, and each requires specific electrode structures to optimise key performance factors such as background, sensitivity and bioattachment. Moreover, some analytes may require extremely sensitive detection methods, encouraging the use of specialised electrode configurations to increase signal amplification and lower detection limits. Furthermore, interference in samples or contaminants can be reduced through surface modifications, increasing the accuracy and reliability of measurements. Also, stability is critical for long-term biosensor reliability therefore specific electrode materials and designs are selected to provide improved results in longer operating life. Considerations such as miniaturization, cost-effectiveness, and availability of materials also influence the selection of electrode configurations for point-of-care applications. Overall, the use of different electrode configurations allows the development of optimized biosensors for a wide range of analytical tasks and applications, providing reliable and accurate detection in a variety of environments.
Many researchers have tried to develop electrochemical biosensors to measure common liver biomarkers. For instance, Feyzi-barnaji et al.38 and Choosang et al.39 used different electrode configurations to detect HSA (human serum albumin) with anti-HSA, and both obtained results comparable to existing immunosensors developed for HSA. The sensor developed by Quan et al.25 for ALT detection exhibited better LOD, sensitivity, and linear range properties than previously reported electrochemical ALT sensors. Parnianchi et al.36 designed the biosensor with the lowest currently reported LOD value for bilirubin detection with MIP-based electrode (OPD/MWCNT/GCE). In a subsequent study, they conducted another bilirubin detection system with a different electrode configuration (AuFe2O3-GrCNT/GCE) and obtained results in two different linear ranges with acceptable values compared with existing sensors.42 All of these developments highlight the potential of novel electrode designs. In conclusion, electrochemical detection of liver biomarkers continues to develop by seeking improved sensitivity, lower detection limits, and wider linear ranges.
4.1. Liver biomarker-specific composite electrodes
Composite electrodes are built by combining multiple materials with complementary properties. Once these materials are synthesized or functionalized, they are coated on a conductive substrate such as a glassy carbon electrode (GCE) to form the composite electrode. For example, Quan et al.25 first synthesized MXene from titanium aluminum carbide (Ti3AlC2) using an environmentally friendly etching process with lithium fluoride (LiF) and hydrochloric acid (HCl) to design graphene@MXene composite electrode. Then, they combined this MXene with graphene dispersion, followed by ultrasonic treatment to ensure effective integration and prevent self-stacking. GCE was first modified by applying graphene@MXene composite, then the surface was coated with pyruvate oxidase (POx) solution containing bovine serum albumin (BSA) and glutaraldehyde. ALT detection was achieved through two-step enzymatic reaction where ALT catalyzes the conversion of α-ketoglutarate and L-alanine into pyruvate and glutamic acid, with the resulting pyruvate oxidized by POx, generating hydrogen peroxide (H2O2) that was detected electrochemically. The advantages of this construction include high conductivity and stability, which improve the performance and sensitivity of the sensor, while complexity of the manufacturing process is a disadvantage. In another example, Mahato et al.33 designed an electrode (Fig. 1) that was modified with sequential deposition of gold nanoparticles (AuNPs), Au-nano-Dendroids, and graphene oxide (GO) together with the anti-ALP to create a functional sensing platform. Although it contains a biomolecule and nanomaterials, its primary structure and functionality are based on the integration of nanomaterials within a composite framework. The detection of ALP was achieved through a label-free immunosensing mechanism, where anti-ALP antibodies were immobilized on the GO-modified surface, allowing for specific binding with ALP in serum samples. This interaction generated an immuno-complex that alters the charge transfer resistance, which was monitored using electrochemical impedance spectroscopy (EIS). The advantages of this construction include high sensitivity, selectivity, and robustness, enabling accurate detection of ALP levels in clinical samples, as well as a relatively simple fabrication process. However, potential drawbacks may include the complexity of the multi-layered structure, which could introduce challenges in reproducibility and stability over time.
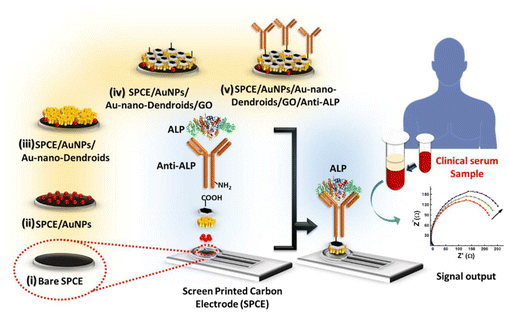 |
| Fig. 1 Illustration of ALP detection on the composite electrode.33 | |
4.2. Liver biomarker-specific molecularly imprinted polymer-based electrodes
MIPs represent an artificial polymer class, with recognition properties close to natural receptors (antibodies, enzymes, nucleic acids etc.) and even better chemical and physical stability than natural receptors.43 First, a polymer film is formed by polymerization which is carried out with a target analyte or a molecular template that mimics the structure of the target analyte.43 The resulting polymer film contains cavities or binding sites that are complementary to the shape, size, and functional groups of the analyte. Then, by depositing this polymer film on the electrode surface, MIP-modified electrodes are obtained.
When the MIP approach is combined with the advantages of electrochemical sensing techniques, interest in MIP-based electrochemical sensors has increased. Wang et al.44 reviewed MIP-based electrochemical sensors focusing on the applied electrochemical techniques and compared the sensor performance with other reported papers. In addition to MIP's features such as being less affected by environmental variations such as temperature, homo- or hetero-shaped cavity design ability, and long lifetime, the issues waiting to be solved are also highlighted in the review.44 In the study conducted by Zhang et al.,13 whose detection scheme is given in Fig. 2, gold electrode (GE) was first modified with AuNPs, and then polythionine-methylene blue (PTH-MB) was added. Finally, the surface was coated with MIPs which were synthesized through electro-polymerization using HSA as the template. HSA detection was achieved through a dual-signal strategy, where the biosensor generates two distinct current signals corresponding to the redox couple Fe(CN)63−/4− and PTH-MB. Upon the binding of HSA to the MIPs, both current signals decrease, allowing for quantification of HSA concentration based on the change in current (ΔI). The advantages of this construction include high sensitivity, a wide detection range, and good selectivity against potential interfering substances, while potential drawbacks may include the need for careful optimization of experimental conditions to ensure reproducibility and stability of the biosensor's performance.
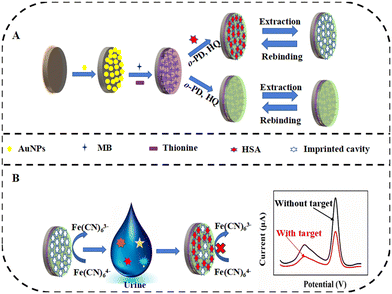 |
| Fig. 2 Schematic of (A) MIP-based sensor construction and (B) electrochemical detection of HSA.13 | |
4.3. Liver biomarker-specific thin-film electrodes
Thin-film electrodes are obtained by depositing a conductive material such as Au or Pt in a thin layer on glass or silicon wafers using techniques such as sputtering or evaporation. The photolithography process is used to produce micron-sized electrodes. The thin layer of conductive material ensures efficient electron transfer between the electrode and the analyte solution, thus achieving reliable and repeatable electrochemical measurements. Saygili et al.27 produced a platinum (Pt) thin-film electrode (Fig. 3) that focuses on integrating an enzymatic reaction with electrochemical sensing to facilitate the detection of ALT. The electrode was constructed using microelectromechanical systems (MEMS) technology which allows for a compact and efficient platform that enhances sensitivity and minimizes sample volume. As described before ALT catalyzes the conversion of L-alanine and α-ketoglutarate to glutamate. Generated glutamate reacts with the glutamate oxidase (GluOx) and is converted to H2O2 which is then electrochemically oxidized at the Pt electrode. This process was monitored using DPV, where the current response correlates with ALT concentration, enabling quantitative analysis. This construction has advantages such as high sensitivity, rapid response times, and the potential for real-time monitoring. However, the need for careful calibration and potential interference from other electroactive species in complex biological samples can be considered as disadvantages.
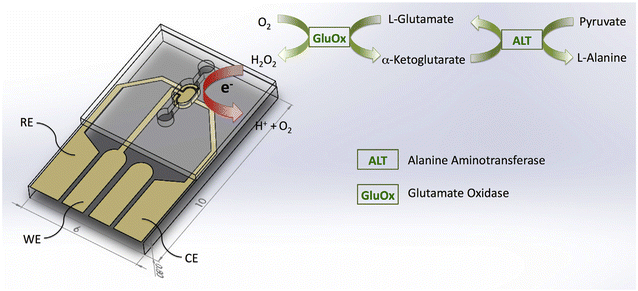 |
| Fig. 3 Illustration of ALT detection on the Pt thin-film electrode.27 | |
4.4. Liver biomarker-specific thick-film screen printing electrodes
Screen-printed electrodes are preferred in many applications with their structure that allows miniaturization and modification. These electrodes are obtained by depositing carbon or metallic inks in a thick film layer on plastic or ceramic substrates.45 While carbon is relatively inexpensive, gold facilitates surface modification with proteins using self-assembled monolayer (SAM) formation. Therefore, gold, carbon-containing graphite, graphene, and carbon nanotubes (CNTs) are generally used for working electrode (WE). While the same inks as WE are generally used in the production of counter electrodes (CE), silver/silver chloride inks are preferred for reference electrode (RE).45 The ink configuration determines the electrochemical properties of the electrode, so inks are selected according to the desired properties. For example, Hsueh et al.29 preferred to use iridium nanoparticles dispersed on carbon ink to improve the electrocatalytic ability of their electrodes. Produced single-use, disposable biosensor platform facilitated the detection of AST through a simple two-step enzymatic reaction that generates H2O2, which was then quantified amperometrically at a low oxidation potential of +0.3 V versus a printed Ag/AgCl reference electrode. While specificity may be a disadvantage of this sensor, its compatibility with clinical spectrophotometric results and ability to detect AST levels significantly above normal limits make it a useful tool for in vitro diagnosis.
4.5. Liver biomarker-specific nanomaterial-modified electrodes
Nanomaterial-modified electrodes are obtained by depositing or immobilizing nanomaterials on a substrate containing a conductive material such as carbon or gold. Nanomaterials such as nanoparticles, nanotubes, and nanosheets are used in cases where it is desired to increase electrochemical performance, such as expanding the surface area of the electrode, improving conductivity or catalytic activity. In the research carried out by Anzar et al.,35 it was determined that after the silver nanoparticle (AgNP) was deposited on the WE surface, the electron transfer kinetics accelerated and thus the current response increased by two-fold. The design strategy of the produced electrode involves the functionalization of paper-based screen-printed electrodes with AgNPs and then immobilization of bilirubin oxidase (BOx). Bilirubin detection was achieved through an electrochemical process where BOx catalyzes the oxidation of bilirubin to biliverdin, resulting in the generation of H2O2, which was monitored by CV and linear sweep voltammetry (LSV). While the electrode structure offers significant advantages like enhanced sensitivity and portability for bilirubin detection, the stability of the enzyme immobilized on the electrode can be a concern, as enzymes can degrade over time, potentially affecting the sensor's performance.
4.6. Liver biomarker-specific biomolecule-modified electrodes
Biomolecule-modified electrodes are obtained by immobilizing biomolecules such as enzymes, aptamers, or antibodies to the electrode surface. These biomolecules are selected according to their ability to selectively recognize and bind to the target analyte and can be immobilized to the surface by physical adsorption or chemical attachment methods. Unlike nanomaterial-based electrodes, the primary aim here is not to increase electrochemical performance but to selectively interact with target analytes. In the study conducted by Choosang et al.,39 target analyte detection (Fig. 4) was carried out with analyte-specific antibodies immobilization. First, screen-printed carbon electrode (SPCE) was modified with poly(3,4-ethylenedioxythiophene):poly(styrenesulfonate) and ferrocene (PEDOT:PSS-Fc), followed by the deposition of poly(para-phenylenediamine) (PpPD) and AuNPs. Thanks to this layered structure, the electrode surface area was increased and the immobilization of anti-HSA onto AuNPs via direct chemisorption was facilitated. Detection of HSA was achieved by measuring the change in the oxidation peak current of ferrocene, which occurs upon the formation of the HSA/anti-HSA immunocomplex, allowing for sensitive quantification of HSA concentrations. While high sensitivity, reusability, and excellent specificity make this construction a suitable choice for clinical diagnostics, the complexity of the manufacturing process and the need to carefully optimize the electrode surface to ensure consistent performance across different samples stand out as disadvantages.
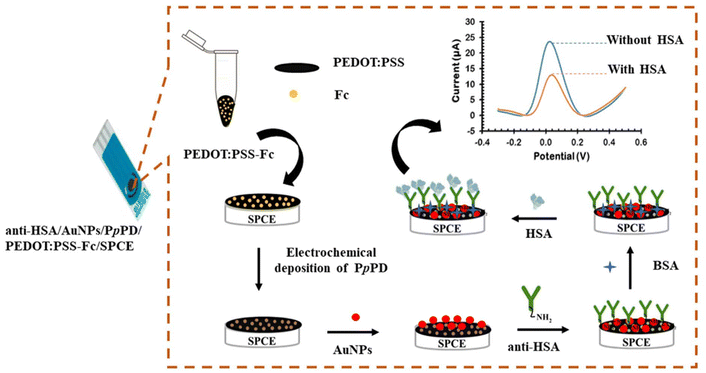 |
| Fig. 4 Illustration of HSA detection.39 | |
4.7. Exploitation of liver biomarker-specific probes
Specific probes are obtained by functionalizing electrode surfaces with molecules or receptors that are highly selective for the target analyte. Although the primary function of biomolecule-modified electrodes is selective target binding, they can also improve electrochemical performance, but specific probes are designed specifically for the selective detection of a single target molecule. For example, Kumaragurubaran et al.12 obtained a high-affinity electrochemical sensing platform with the activity-based electrochemical probe (GTLPOH) they produced for the specific direct targeting of GGT activity. The GTLPOH probe was produced through a synthetic strategy that involves the design of a gamma-glutamyl amide moiety, which is specifically tailored to be hydrolyzed by the GGT. The incorporation of a methylene hydroxyl group in GTLPOH enhanced its hydrophilicity and solubility, which allowed for improved interaction with the enzyme. GGT detection was successfully achieved by the current change that occurred when the probes interacted with GGT. High sensitivity, the ability to directly analyze turbid samples like whole blood and urine without extensive sample preparation, and rapid results make this platform suitable for point-of-care applications. However, there is also a need for disposable electrode strips and minimizing the sampling size.
All these electrode configurations have been developed to improve the effectiveness of biosensing applications. The choice of configuration to use varies depending on the nature of the target analyte and the requirements of the application, such as sensitivity and selectivity. The key advantages and disadvantages of the electrode configurations are briefly summarized in Table 2.
Table 2 Overview of electrode constructions employed for the detection of liver biomarkers
Electrode type |
Electrode modification |
Advantages |
Disadvantages |
Composite electrode |
• POx/graphene@MXeme/GCE25 |
• High sensitivity |
• Complex fabrication process |
• GCE/NC20 |
• Excellent electrocatalytic activity |
• Potential for electrode fouling |
• CoOOH/TCPB-DMTA-COF/GCE31 |
• Potential for performance variation |
• SPCE/AuNPs/Au-nanoDendroids/GO/anti-ALP33 |
• BOx/GO@PANI/ITO34 |
• TDMAC/DOP/MWCNT37 |
• CS/CuO NPs /ERGO/GCE38 |
Molecularly imprinted polymer |
• POx MIP-modified PME26 |
• Selective recognition of target analyte |
• Limited versatility |
• GE/AuNPs/PTH-MB/MIP13 |
• Stable performance |
• Requires careful optimization for each analyte |
• OPD/MWCNT/GCE36 |
Thin-film electrode |
• Platinum TFE27 |
• High stability |
• Limited surface area |
• Good reproducibility |
• May require special deposition techniques |
• Suitable for miniaturization |
Thick-film screen printing |
• Prussian-blue modified screen-printed carbon electrodes28 |
• Cost-effective |
• Limited sensitivity compared to other electrodes |
• Screen-printable Ir–C electrode29 |
• Disposable |
• Easy fabrication |
Specific probe |
• GTLPOH probe12 |
• Highly selective |
• Limited versatility |
• Specific to the target analyte |
• May require a specialized probe design |
Nanomaterial-modified electrode |
• Enzyme/Ag-NPs/PBs35 |
• High surface area |
• Synthesis complexity |
• Improved electron transfer |
• Potential instability of nanomaterials |
• Enhanced sensitivity |
Biomolecule-modified electrode |
• antiHSA/AuNPs/PpPD/PEDOT:PSS-Fc/SPCE39 |
• Selective detection |
• Complex fabrication process |
• Multienzyme-modified gold WE, platinum CE, and an external Ag/AgCl RE30 |
• High sensitivity |
• Limited stability |
• ssDNA-modified gold WE, platinum wire CE, and saturated calomel RE32 |
• Potential for biomolecule degradation |
• GSH modified gold WE, platinum wire CE, saturated calomel RE40 |
5. Conclusions
In conclusion, the use of electrochemical methods for the detection of liver biomarkers enables significant advances in the field of liver disorders detection and monitoring by providing rapid, sensitive, and affordable diagnostic options. However, current electrochemical sensors mostly focus on individual biomarkers, and there remains a need for comprehensive assessment tools that can detect multiple biomarkers simultaneously to provide a holistic assessment of liver function, especially as informative proteomic signatures of different liver diseases emerge from the bioinformatics space. Furthermore, by optimizing the advantages and disadvantages of electrode configurations, progress can be made on the need for point-of-care devices. In summary, by addressing current limitations, electrochemical methods can unlock the full potential of the translational opportunity associated with liver disease detection and pave the way for more effective diagnostic tools and personalized treatment strategies in liver health. In this way, the burden of liver diseases on individuals and healthcare systems worldwide can be reduced.
Data availability
No primary research results, software or code have been included and no new data were generated or analysed as part of this review.
Conflicts of interest
There are no conflicts to declare.
Acknowledgements
This work was supported by the Republic of Turkey Ministry of National Education (MoNE-1416/YLSY).
References
- H. Devarbhavi, S. K. Asrani, J. P. Arab, Y. A. Nartey, E. Pose and P. S. Kamath, J. Hepatol., 2023, 79, 516–537 CrossRef PubMed.
- J. C. Ozougwu, International Journal of Research in Pharmacy and Biosciences, 2017, 4, 13–24 Search PubMed.
- G. Kasarala and H. L. Tillmann, Clin. Liver Dis., 2016, 8, 13–18 CrossRef PubMed.
- X. Liu, X. Mei, J. Yang and Y. Li, ACS Appl. Mater. Interfaces, 2022, 14, 6985–6993 CrossRef CAS PubMed.
- P. E. Resmi, S. S. Kumar, D. Alageswari, P. V. Suneesh, T. Ramachandran, B. G. Nair and T. G. S. Babu, Anal. Chim. Acta, 2021, 1188, 339158 CrossRef CAS PubMed.
- C.-Y. Xi, M. Zhang, L. Jiang, H.-Y. Chen, J. Lv, Y. He, M. E. Hafez, R.-C. Qian and D.-W. Li, Sens. Actuators, B, 2022, 369, 132264 CrossRef CAS.
- W. Zhao, S. Yang, D. Zhang, T. Zhou, J. Huang, M. Gao, X. Zhang, Y. Liu and J. Yang, J. Colloid Interface Sci., 2023, 646, 872–882 CrossRef CAS PubMed.
- B.-Y. Liao, C.-J. Chang, C.-F. Wang, C.-H. Lu and J.-K. Chen, Sens. Actuators, B, 2021, 336, 129710 CrossRef CAS.
- X. Y. Yang, Y. Y. Bai, Y. Y. Huangfu, W. J. Guo, Y. J. Yang, D. W. Pang and Z. L. Zhang, Anal. Chem., 2021, 93, 1757–1763 CrossRef CAS PubMed.
- Y. Cui, L. Zhang, B. Shi, S. Chen and S. Zhao, Sens. Actuators, B, 2021, 344, 130080 CrossRef CAS.
- B. Wang, X.-Q. Zhou, L. Li, Y.-X. Li, L.-P. Yu and Y. Chen, Sens. Actuators, B, 2022, 369, 132392 CrossRef CAS.
- N. Kumaragurubaran, H. T. Tsai, P. Arul, S. T. Huang and H. Y. Lin, Biosens. Bioelectron., 2024, 248, 115996 CrossRef CAS PubMed.
- G. Zhang, Y. Yu, M. Guo, B. Lin and L. Zhang, Sens. Actuators, B, 2019, 288, 564–570 CrossRef CAS.
- A. J. Bard, L. R. Faulkner and H. S. White, Electrochemical methods: fundamentals and applications. John Wiley & Sons, 2022 Search PubMed.
- J. Baranwal, B. Barse, G. Gatto, G. Broncova and A. Kumar, Chemosensors, 2022, 10, 363 CrossRef CAS.
- R. Singh, R. Gupta, D. Bansal, R. Bhateria and M. Sharma, ACS Omega, 2024, 9, 7336–7356 CAS.
- M. J. Song, D. H. Yun, N. K. Min and S. I. Hong, J. Biosci. Bioeng., 2007, 103, 32–37 CrossRef CAS PubMed.
- X.-J. Huang, Y.-K. Choi, H.-S. Im, O. Yarimaga, E. Yoon and H.-S. Kim, Sensors, 2006, 6, 756–782 CrossRef CAS.
- X. Niu, K. Ye, L. Wang, Y. Lin and D. Du, Anal. Chim. Acta, 2019, 1086, 29–45 CrossRef CAS PubMed.
- A. Kumar, G. H. Gupta, G. Singh, N. More, K. M., A. Sharma, D. Jawade, A. Balu and G. Kapusetti, Biosens. Bioelectron.: X, 2023, 13, 100290 CAS.
- H. Liu, F. Liu, F. Wang, R. Q. Yu and J. H. Jiang, Analyst, 2018, 143, 5530–5535 RSC.
-
V. Lala and M. ZubairLiver Function Tests, https://www.statpearls.com/point-of-care/20995/#ref_31628070, (accessed Jun 27, 2024).
- S. Curry, H. Mandelkow, P. Brick and N. Franks, Nat. Struct. Biol., 1998, 5, 827–835 CrossRef CAS PubMed.
- M. Cieplak, K. Szwabinska, M. Sosnowska, B. K. Chandra, P. Borowicz, K. Noworyta, F. D'Souza and W. Kutner, Biosens. Bioelectron., 2015, 74, 960–966 CrossRef CAS PubMed.
- C. Quan, L. Quan, Q. Wen, M. Yang and T. Li, Mikrochim. Acta, 2023, 191, 45 CrossRef PubMed.
- M. A. Samy, M. A.-H. Abdel-Tawab, N. T. Abdel-Ghani and R. M. El Nashar, Chemosensors, 2023, 11, 262 CrossRef CAS.
- E. Saygili, B. Orakci, M. Koprulu, A. Demirhan, E. Ilhan-Ayisigi, Y. Kilic and O. Yesil-Celiktas, Anal. Biochem., 2020, 591, 113538 CrossRef CAS PubMed.
- S. Moed and M. H. Zaman, Biosens. Bioelectron., 2019, 131, 74–78 CrossRef CAS PubMed.
- C. J. Hsueh, J. H. Wang, L. Dai and C. C. Liu, Biosensors, 2012, 2, 234–244 CrossRef CAS PubMed.
- Y. D. Han, S. Y. Song, J. H. Lee, D. S. Lee and H. C. Yoon, Anal. Bioanal. Chem., 2011, 400, 797–805 CrossRef CAS PubMed.
- L. Cui, C.-Y. Zhu, J. Hu, X.-M. Meng, M. Jiang, W. Gao, X. Wang and C.-Y. Zhang, Sens. Actuators, B, 2023, 374, 132779 CrossRef CAS.
- W. Wang, J. Lu, L. Hao, H. Yang, X. Song and F. Si, Anal. Bioanal. Chem., 2021, 413, 1827–1836 CrossRef CAS PubMed.
- K. Mahato, B. Purohit, A. Kumar and P. Chandra, Biosens. Bioelectron., 2020, 148, 111815 CrossRef CAS PubMed.
- N. S. Ahmed, C. Y. Hsu, Z. H. Mahmoud, H. Sayadi and E. Kianfar, RSC Adv., 2023, 13, 36280–36292 RSC.
- N. Anzar, S. Suleman, R. Kumar, R. Rawal, C. S. Pundir, R. Pilloton and J. Narang, Micromachines, 2022, 13, 1845 CrossRef PubMed.
- F. Parnianchi, S. Kashanian, M. Nazari, C. Santoro, P. Bollella and K. Varmira, Microchem. J., 2021, 168, 106367 CrossRef CAS.
- A. H. Kamel, N. H. Ashmawy, T. A. Youssef, M. Elnakib, H. A. El-Naby and H. S. M. Abd-Rabboh, Electroanalysis, 2023, 35, e202200436 CrossRef CAS.
- B. Feyzi-barnaji, B. Darbasizadeh, E. Arkan, H. Salehzadeh, A. Salimi, F. Nili, R. Dinarvand and A. Mohammadi, J. Electroanal. Chem., 2020, 877, 114642 CrossRef CAS.
- J. Choosang, P. Thavarungkul, P. Kanatharana and A. Numnuam, Microchem. J., 2020, 155, 104709 CrossRef CAS.
- G. Chen, S. Ni, S. Zhu, J. Yang and Y. Yin, Int. J. Mol. Sci., 2012, 13, 2801–2809 CrossRef CAS PubMed.
- S. Upadhyay, N. Ohgami, H. Kusakabe and H. Suzuki, Biosens. Bioelectron., 2006, 21, 1230–1236 CrossRef CAS PubMed.
- F. Parnianchi, S. Kashanian, M. Nazari, M. Peacock, K. Omidfar and K. Varmira, Microchem. J., 2022, 179, 107474 CrossRef CAS.
- N. Leibl, K. Haupt, C. Gonzato and L. Duma, Chemosensors, 2021, 9, 123 CrossRef CAS.
- L. Wang, M. Pagett and W. Zhang, Sens. Actuators Rep., 2023, 5, 100153 CrossRef.
- G. Paimard, E. Ghasali and M. Baeza, Chemosensors, 2023, 11, 113 CrossRef CAS.
|
This journal is © The Royal Society of Chemistry 2024 |
Click here to see how this site uses Cookies. View our privacy policy here.