DOI:
10.1039/C8QM00570B
(Review Article)
Mater. Chem. Front., 2019,
3, 181-192
Cyclodextrin-based sustained gene release systems: a supramolecular solution towards clinical applications
Received
6th November 2018
, Accepted 25th November 2018
First published on 26th November 2018
Abstract
Recently, sustained release vectors, especially cyclodextrin (CD) based supramolecular carriers, have been reported to play an important role and have spurred interesting developments as novel gene delivery systems. This review will summarize the recent designs of CD-based supramolecular structures as sustained gene release systems in terms of CD-based graft copolymers, polyrotaxanes or polypseudorotaxanes, and supramolecular hydrogels; the advantages of these CD-based supramolecular carriers in terms of biocompatibility, gene transfection ability and controllable release rate by tuning the de-threading of CDs; and the applications of these CD-based supramolecular sustained gene release systems in disease treatments by taking advantage of their increase of the therapeutic gene's treatment period, thereby improving the gene transfection efficiency and avoiding repeated administration to increase patient compliance. Last but not least, the further endeavour towards CD-based supramolecular structures as more precise sustained gene release systems will also be discussed.
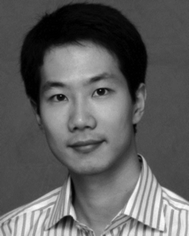
Yun-Long Wu
| Yun-Long Wu is currently an Associate Professor at the School of Pharmaceutical Sciences, Xiamen University (China). He received his BS degree in materials science from University of Science and Technology of China in 2005 and PhD degree in Bioengineering from National University of Singapore in 2011. After his postdoctoral fellow training at Nanyang Technological University (Singapore), he joined Xiamen University as an assistant professor in 2012 and was promoted to be an associate professor in 2014. His research focuses on supramolecular polymeric structures or functional polymeric materials with potential applications in drug delivery, gene delivery, and cancer therapy. |
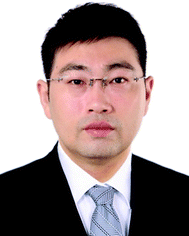
Zibiao Li
| Li Zibiao obtained his PhD from National University of Singapore (NUS) in 2014. Currently, he is working as a research scientist at the Institute of Materials Research and Engineering (IMRE), A*STAR, Singapore. He is the deputy department head of the Soft Materials Department in IMRE. His research interests are focused on design of biodegradable and functional polymeric materials, structural property investigations and formulations for healthcare and consumer care applications. |
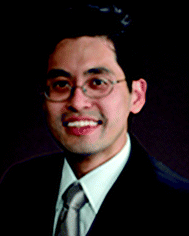
Xian Jun Loh
| Xian Jun Loh is a polymer chemist working in the inter-disciplinary field of biomaterials. He is a senior scientist and currently the department head of the Soft Materials at the Institute of Materials Research and Engineering (IMRE) and a faculty member of the National University of Singapore (NUS). He is the current Vice President of the Singapore National Institute of Chemistry and a fellow of the Royal Society of Chemistry. His main research interests are in the design of supramolecular and stimuli-responsive polymers and hydrogels for biomedical and personal care applications. |
1. Introduction
Though gene therapy has shown promising therapeutic effects over the past few years, its clinical trials still face a number of challenges, especially in terms of achieving sustained and continuous gene expression in targeted cells or tissues to meet the clinical demand.1–14 Recently, non-viral sustained release gene or drug delivery vectors, which aim to provide a persistent concentration of therapeutics to disease sites and to improve the transfection efficiency with low toxicity, have shown attractive applications in treating locoregional diseases such as primary tumors, angiogenesis or reproducible tissue regeneration.15–20 To achieve sustained gene delivery, a variety of non-viral vectors have been designed by taking advantage of natural or synthetic polymers with invisibility to the immune system.21,22 Typically, natural polymers (i.e., agarose,23 hyaluronan,24 chitosan,25 or alginate26) have been proposed as sustained gene delivery vectors at an early stage. Although natural polymers are able to provide a mild environment for genes and can be degraded into the biocompatible components of the extracellular matrix, their gene transfection efficiency is still far from satisfaction. Therefore, an alternative approach by attaching genes to synthetic polymers, such as poly(L-glutamic acid) (PLGA), poly(ethyleneimine) (PEI), poly(L-lysine) (PLL), and poly(2-(dimethylamino) ethyl methacrylate) (PDMAEMA),27 in the form of hydrogels, nanospheres, microspheres, or scaffolds,28,29 has been utilized to achieve the sustained release of genes. It is worth mentioning that there are several advantages of polymer-mediated sustained release systems.30–32 Firstly, the therapeutic gene can be protected from the aggressive environment before release.33 Secondly, the polymers can be modified by various methods to achieve targeting specificity.34 Thirdly, the encapsulated gene can be released from the gene–polymer complexes at a controllable constant rate and transferred into the cell efficiently.35 Last but not least, they can maintain therapeutic gene concentrations in targeted cells or tissues for a long time, and therefore repeated administration is circumvented and patient compliance can be improved.36
Among recently reported sustained gene delivery systems, cyclodextrin (CD) based supramolecular systems have attracted wide interest.37 Cyclodextrin is a general term for a series of biocompatible cyclic oligosaccharides produced by amylose with the assistance of cyclodextrin glucosyltransferase from bacillus, which possess the ability to serve as host molecules to encapsulate guest components to form supramolecular structures in a self-assembly manner.38,39 Interestingly, recent reports showed that CD based supramolecular structures could be designed as sustained gene release systems (Fig. 1). More importantly, these supramolecular architectures exhibited high transfection efficiency, lower carrier cytotoxicity, and a controllable gene release rate by tuning the CD concentration.40 Typically, Lin et al. experimentally revealed that higher CD concentrations might lead to slower gene release rates.41 In short, CD based supramolecular structures, with high biocompatibility, satisfactory gene transfection efficiency, and gene release rate adjustability, show great advantages in sustained gene delivery as well as disease treatment.42 However, a comprehensive summary of the recent advances of CD based supramolecular structures as sustained gene delivery systems as well as their potential therapeutic applications is lacking. Hence, this review covers the recent designs of CD based supramolecular structures, in terms of CD-grafted polymers, polyrotaxanes or polypseudorotaxanes, and supramolecular hydrogels, as well as their unique properties in therapeutic applications, to provide the frontiers of material chemistry in CD and its various self-assembly forms for sustained gene delivery as well as precision medicine.
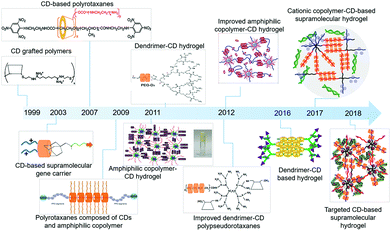 |
| Fig. 1 The advances of cyclodextrin-based sustained gene delivery systems in recent years. Reproduced with permission.41 Copyright 2016, Elsevier. Reproduced with permission.43 Copyright 1999, American Chemical Society. Reproduced with permission.44 Copyright 2007, American Chemical Society. Reproduced with permission.45 Copyright 2009, Elsevier. Reproduced with permission.46 Copyright 2012, Elsevier. Reproduced with permission.47 Copyright 2011, Royal Society of Chemistry. Reproduced with permission.48 Copyright 2012, Elsevier. Reproduced with permission.49 Copyright 2012, American Chemical Society. Reproduced with permission.50 Copyright 2017, John Wiley and Sons. Reproduced with permission.51 Copyright 2018, John Wiley and Sons. | |
2. Design of cyclodextrin-based structures for sustained gene delivery
2.1 Cyclodextrin-based graft copolymer for gene delivery
Due to the features of bioavailability, biocompatibility and charge density enhancing capability, cyclodextrins (CDs) are connected with cationic polymers to achieve improved gene transfection efficiency. Generally speaking, cyclodextrin-based polymeric structures are formed by linking the cyclodextrin core with cationic copolymer monomers with low cytoxicity.52 The first CD based cationic polymer for gene delivery was reported in 1999, when modified β-cyclodextrin (β-CD) was conjugated with dimethylsulfate (DMS) or dithiobis(succinimidyl propionate) (DSP) to form low cytotoxic polycations, with the ability to compress genes into small size particles and to show similar transfection efficiency to poly(ethylene imine) (PEI).43 Furthermore, their gene delivery capacity or transfection efficiency and cytotoxicity largely depended on the polymeric structure and molecular weight.53 More interestingly, the CD-based polymers could be further cast into a film form to achieve sustained release of the gene. As a typical example, Hu et al. loaded a conjugate of PEI and CD with plasmid deoxyribonucleic acid (PEI–CD/pDNA), and cast them with poly(D,L-lactic acid) (PDLLA) films, which allowed PEI–CD/pDNA to accumulate in the film layers.54 The experimental results showed that the pDNA could be gradually released for 28 h, with degradation of the multilayer film, and exhibited higher gene transfection efficiency than the control group without CD conjugation.
The conventional cationic cyclodextrin-based polymers were designed as linear repeating units, which were linked through the covalent molecular interactions between CDs and polymer monomers. Recently, more dense structures based on conjugating multiple cationic arms onto one CD core to greatly enhance the local charge density were explored. Typically, Yang et al. designed a star shaped α-CD-OEI polymer, in which the α-CD core was linked to many oligomeric ethyleneimine (OEI) arms.56 Experimental results revealed that, compared with high molecular weight branched PEI, the cell toxicity of α-CD-OEI vectors was smaller while the gene expression ability was still high, which served as an ideal strategy to achieve satisfactory gene transfection with low cytotoxicity. Similarly, Xu et al. designed another star-like polymer composed of β-CD cores and short cationic poly(2-(dimethylamino)ethyl methacrylate) (PDMAEMA) arms with further conjugation to poly(ethylene glycol) (PEG).57 Compared with high molecular weight PDMAEMA polymers, this unique structure exhibited much better biocompatibility and higher gene transfection efficiency. It is also worth mentioning that CD-based cationic polymers with a free CD cavity were able to achieve synergistic delivery of genes and small drugs with the ability to serve as guest molecules inside the host CD cavity. For example, as shown in Fig. 2, Ma et al. designed a dendrimer polymer based on the per-6-azido-β-CD (β-CD-(N3)7) and poly(L-lysine) (PLL) dendron for co-delivery of genes and drugs, where the cavity of β-CD could encapsulate hydrophobic anticancer drugs and the cationic PLL groups could bind DNA, exhibiting good gene transfection efficiency and sustained drug release behavior.55
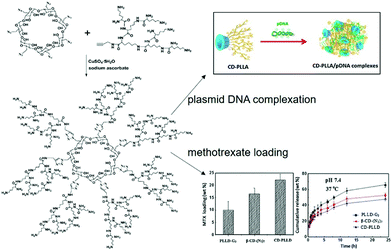 |
| Fig. 2 Design of cationic dendrimeric polymers based on per-6-azido-β-CD and PLL dendrons for co-delivery of genes and drugs. Reproduced with permission.55 Copyright 2013, Elsevier. | |
Due to the unique hydrophobic cavity of CD, CD-based cationic gene delivery vectors have the ability to achieve surface functionalization or targeting ligand modification by easily forming an inclusion complex, without disturbing particle morphology or polycation/DNA interactions. As a typical case, Bellocq et al. synthesized a cancer cell targeting CD-based gene delivery vehicle made of an inclusion complex between a cationic CD derivative polymer to condense pDNA and transferrin (Tf) as well as adamantyl end-terminated PEG.58 The adamantly group could serve as a guest molecule to form an inclusion complex with the host CD molecule by self-assembly, which introduced the PEG group into the CD derivative cationic polymer for stability or biocompatibility increase and the Tf targeting group to allow the complex to recognize cancer cells with abundant transferrin receptors (TfRs). It represented a popular approach to modify CD based vectors to achieve targeted delivery of the therapeutic gene by host–guest self-assembly, including transferrin for TfR recognition,59,60 gold (Au) nanoparticles for targeted photo-thermal treatment,61 and peptides for fibroblast growth factor receptor (FGFR) targeting.62
2.2 Cyclodextrin-based polyrotaxanes and polypseudorotaxanes for sustained gene release
In recent years, supramolecular polyrotaxanes and polypseudorotaxanes have received increasing attention owing to their exceptional architecture and low material toxicity, and they are widely applied in drug and gene delivery.63,64 Polyrotaxane is a supramolecular complex formed by cyclic molecules threaded onto a linear polymeric chain, whose ends are blocked by large capping molecules to prevent the cyclic component leakage and to maintain its stability. Different from polyrotaxane, polypseudorotaxane has no hindered molecules at both ends of the linear chain, where the cyclic molecule can freely dissociate and associate from the supramolecular architecture. It is worth mentioning that polyrotaxanes or polypseudorotaxanes are composed of multiple rings passing through a linear polymer, which is different from ordinary polymers with covalent linkage of repeating units and limited freedom. Furthermore, the cyclic group can freely rotate or slide on the chain molecule for polyrotaxanes, while the de-threading of macrocycles is stimulated upon cleavage of large terminal groups. Due to this free mobility of the cyclic molecule on the chain polymer, polyrotaxanes or polypesudorotaxanes are favorable structures in facilitating the interaction between the cationic polymer and the anionic gene.65 Hence, a number of reports have investigated the utilization of CD-based polyrotaxanes or polypseudorotaxanes for gene delivery, which exhibited good biocompatibility and excellent gene delivery capabilities.
2.2.1 Cyclodextrin-based polyrotaxanes.
By taking advantage of molecular recognition, cyclodextrin molecules might form supramolecular polyrotaxane structures with linear poly(ethylene glycol) (PEG) or poly(propylene glycol) (PPG) with large terminal groups. Due to size fitting, linear PEG can form supramolecular complexes with the α-CD cavity, as one α-CD molecule might thread onto two –CH2CH2O– repeating units of PEG.66 By taking advantage of this, Ooya et al. designed an α-CD-based polyrotaxane showing good gene delivery efficiency, in which α-CD was modified with cationic N,N-dimethylamino-ethylmethacrylate (DMAEMA) arms to form a polyplex with the anionic gene, while the linear PEG chain passed through the annular cavity of α-CD and was further blocked by larger benzyloxycarbonyl tyrosine caps at both ends.63 More interestingly, as this self-assembly process occurred in a size dependent manner, α-CD with the smallest cavity size could only thread onto poly(ethylene oxide) (PEO) while β-CD or γ-CD exhibited high affinity to poly(propylene oxide) (PPO) with extra side methyl groups.67 For example, Li et al. designed a polyrotaxane-based gene delivery vector by combining the triblock copolymer of PEO–PPO–PEO and the β-CD ring with cationic oligoethyleneimine (OEI) arm grafting.68 In this case, β-CD was threaded on the PPO fragment and might move freely to unbound PEO segments, which increased the flexibility of cyclic components in anionic gene binding. Furthermore, the block copolymer PEO–PPO could also react with cationic OEI conjugated α-CD to form supramolecular polyrotaxanes for gene delivery. In this case, α-CD could form supramolecular complexes with the PEO segment but not the PPO chain, because the volume of the PPO chain was so voluminous that it might not pass through the smaller α-CD inner ring. Such a polyrotaxane structure also allowed the cyclodextrins to freely rotate and move on the chain, thus enhancing the interaction of cationic groups with anionic DNA and improving gene transfection efficiency.44
It is worth mentioning that the polyrotaxane delivery systems were explored not only for transit gene delivery but also sustained or continuous gene release.69 As shown in Fig. 3, Yang et al. synthesized polyrotaxane carriers composed by multiple α-CDs and the amphiphilic triblock copolymer PPO–PEO–PPO.45 After terminal end capping of the copolymer after polyrotaxane formation, short chain cationic OEI molecules were further conjugated to cyclic α-CD to produce high gene transfection efficiency, which could prolong the gene transfection period due to stability increase. Similarly, polyrotaxane formed by threading the β-CD-OEI loop onto a PEO–PPO–PEO triblock copolymer could also exhibit strong DNA binding ability and sustainable gene transfection efficiency.70
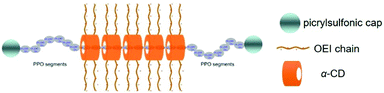 |
| Fig. 3 Design of polyrotaxanes for gene delivery, by threading α-CD with cationic OEI arms onto linear PPO–PEO–PPO triblock copolymers as well as large end group capping. Reproduced with Permission.45 Copyright 2009, Elsevier. | |
2.2.2 Cyclodextrin-based polypseudorotaxanes.
Due to the blockage of terminal capping groups, polyrotaxane systems are still not ideal for sustained gene release vector design. Therefore, more efforts were devoted to synthesizing polypseudorotaxanes to achieve sustained gene release and delivery. Harada et al. firstly used the α-CD macrocycles and the PEG chain to form polypseudorotaxane, while α-CD would detach from the PEG chain when the polypseudorotaxane was dissolved in water.71 Furthermore, Higashi et al. investigated PEGylated proteins and found that this unique protein form could self-assemble into polypseudorotaxane with cyclodextrins and achieve sustained release of protein. Hence, similar ideas were applied to design sustained gene delivery vectors by taking advantage of the polypseudorotaxane structure.72
CD-based polypseudorotaxanes consisting of CDs and PEGylated cationic poly(amidoamine) (PAMAM) dendrimer were firstly utilized for sustained gene delivery by H. Arima's group.73 In detail, PAMAM dendrimers were used for gene binding, while PEG groups could form supramolecular polypseudorotaxanes with either α-CD with single PEG chain threading ability or γ-CD with two PEG segment threading capacity. This unique polypseudorotaxane was reported to serve as a gene delivery vector which could slowly release the encapsulated gene for more than 2 days. Furthermore, the gene release rate could be slowed down by the reduction of the dissolution medium volume. More interestingly, α-CD conjugated dendrimers were reported to show improved transfection capacity and negligible cytotoxicity when compared to the unfunctionalized dendrimers.74 Hence, Motoyama et al. synthesized a hybrid polypseudorotaxane by attaching α-CD to PEGylated PAMAM dendrimer as the core and then interacting with α-CD or γ-CD via supramolecular recognition between CD and PEG (as shown in Fig. 4a), which showed efficient and sustained in vitro pDNA transfection capability for at least 72 h.
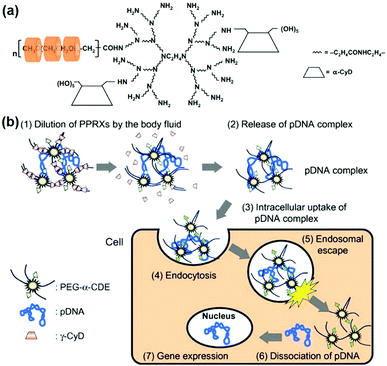 |
| Fig. 4 (a) Design of PEG-α-CDE and CD based polypseudorotaxane; (b) gene release mechanism of polypseudorotaxane after injection into the body. Reproduced with permission.46 Copyright 2012, Elsevier. | |
It should be highlighted that the gene release mechanism of polypseudorotaxanes was largely associated with the shedding of CD from the linear polymer backbone, while the de-threading rate of CD was proportional to the volume of the dissolution medium, as shown in Fig. 4b.46 When injected into the body, the body fluid might gradually dilute the polypseudorotaxane which led to CD fall-off. In this case, the released genes/polymer complexes could be absorbed by surrounding cells through endocytosis. This dilution process might be modulated by adjusting injection sites or changing the molecular weight of the polymeric backbone, while CD might finally be circulated or experience kidney clearance with the ability to reduce the undesired side effects in clinical trials.
2.3 Cyclodextrin-based hydrogels for sustained gene release
Among the currently reported CD-based sustained gene delivery systems, the most successful attempt was the design of supramolecular hydrogels, made of CDs as non-covalent linkers and the corresponding polymer chains. Due to their excellent biocompatibility, reversibility, low irritation, and proper elasticity or viscosity, hydrogels received extensive attention in the biomedical field.75,76 Interestingly, supramolecular hydrogels have been successfully designed as novel delivery vehicles owing to the controlled stability, good biocompatibility, high drug loading and controllable release behaviour.77,78 One salient feature of the novel hydrogels is the absence of cross-linking agents. Unlike conventional chemically crosslinked hydrogels with undesired cytotoxicity concerns due to the utilization of toxic chemical crosslinkers or photo-initiators, supramolecular hydrogels utilized biocompatible CDs with less cytotoxicity as physical crosslinkers due to their unique topological structures and have become popular designs for drug or gene delivery.79,80
In recent years, a series of inclusion complexes between a polymer backbone and CDs were explored to form reversible supramolecular hydrogels with controllable swelling characteristics.81 More interestingly, therapeutic genes could be dispersed into the matrix of these hydrogels to achieve gene delivery.82 Because these CD-based hydrogels were crosslinked by the physical interactions between polymers and their complex with CDs, these unique hydrogels had low cytotoxicity and good biocompatibility, in comparison with chemically cross-linked polymeric hydrogels which involved the usage of chemical crosslinkers or photo-initiators with potential toxicity. Another important feature of CD based supramolecular hydrogels lies in their ability of in situ formation of solid state hydrogels, upon the stimulus of shearing force, temperature or pH changes. Furthermore, a number of studies demonstrated that CD-based supramolecular hydrogels had good sustained release capabilities of encapsulated cargos.83,84 Therefore, attempts were made to use CD-based supramolecular hydrogels to achieve sustained gene release and delivery.
2.3.1 Supramolecular systems based on cyclodextrins and dendrimers for sustained gene delivery.
The formation of polymeric hydrogels required a complex polymeric network, where polymeric dendrimers or higher generation repeated branched molecules represented a good candidate. As a typical example, Deng et al. developed a novel hydrophilic hydrogel matrix to encapsulate plasmid DNA for sustained gene delivery, which was formed by mixing PEGylated polyamidoamine (PAMAM) dendrimer with α-CD, as shown in Fig. 5a. In this design, PEG, which was ratified for in vivo injection by the U.S. Food and Drug Administration (FDA) due to its biocompatibility and low immunogenicity,85 was incorporated to form an inclusion complex with α-CD by self-assembly and physically crosslinked to form a supramolecular hydrogel, while the cationic PAMAM dendron was used for binding with plasmid deoxyribonucleic acid (pDNA) and condensing pDNA into nanoparticles.47 The DNA release profile showed that pDNA polyplexes exhibited sustained release behavior for 5 days without quick release, because the gene release was due to the gradual degradation and destruction of this supramolecular hydrogel. Furthermore, it was revealed that the gene release from the hydrogel was in the form of a polyplex (the nanocomplex between pDNA and polymers) rather than in naked pDNA form, which could protect pDNA from rapid elimination.
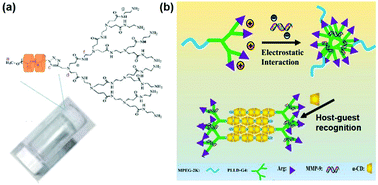 |
| Fig. 5 (a) Structure of the supramolecular hydrogel made of an inclusion complex between PEGylated PAMAM dendrimer and α-CD. Reproduced with permission.47 Copyright 2011, Royal Society of Chemistry. (b) Schematic illustration of the MPEG–PLLD–Arg dendrimer and α-CD based supramolecular hydrogel with anionic gene loading. Reproduced with permission.41 Copyright 2017, Elsevier. | |
In another study, Lin et al. utilized monofunctionalized methyl ether PEG (MPEG) to combine with the arginine-functionalized poly(L-lysine) PLL dendrimer (PLLD–Arg), which further interacted with α-CD by host–guest interactions to form a supramolecular hydrogel, as shown in Fig. 5b.41 In this case, cationic PLL dendrimer was used to carry the anionic gene by electrostatic forces due to its excellent biocompatibility and gene transfection ability, while arginine was utilized to modify MPEG–PLLD copolymers because arginine was reported to increase the gene transfection efficiency of non-viral polymeric vectors. This unique system could achieve sustained gene release continuously for more than 6 days. Furthermore, experimental results revealed that the hydrogel strength could be adjusted by varying the amount of α-CD. In detail, higher α-CD amount led to more rapid gel formation and increased hydrogel strength; higher strength might slow down the rate of hydrogel erosion or degradation and reduce the release rate of the encapsulated gene. In vitro cellular model evaluation showed that this hydrogel had satisfactory gene transfection efficiency, indicating its great potential in future sustained therapeutic gene release applications.
2.3.2 Supramolecular systems based on cyclodextrins and block copolymers for sustained gene delivery.
Besides dendrimers, linear block copolymers could also serve as important backbones for supramolecular hydrogels. Typically, Pluronic (or block copolymer of PEO–PPO–PEO) triblock polymers have been extensively studied to form hydrogels with CDs, with great potential for drug and gene loading. Li et al. firstly reported a novel supramolecular hydrogel composed of triblock copolymer PEO–PPO–PEO and CDs owing to the threading of cyclic CD molecules onto linear polymer chains, which could be easily prepared at room temperature.86 Further study by Ni et al. systematically explored the kinetics, rheology and formation mechanism of this supramolecular hydrogel based on α-CD and PEO–PPO–PEO, and experimental results demonstrated that the α-CD/PEO–PPO–PEO hydrogel with reversibility and thixotropic properties was formed due to inclusion complex formation between α-CD and the PEO group by self-recognition as well as hydrophobic interactions between PPO segments.87
By taking advantage of this, PEO–PPO–PEO was used in subsequent studies to achieve sustained gene delivery. As shown in Fig. 6a, Ma et al. designed a novel polypseudorotaxane based hydrogel to carry plasmid DNA, in which the block copolymer was composed of PEO–PPO–PEO and PLL.48 PEO–PPO–PEO was incorporated to interact with α-CD through supramolecular interactions, and cationic polymer PLL might be combined with anionic plasmid DNA through electrostatic interactions for gene encapsulation. Since heparin sodium might bind to cationic polymers and disrupt the polymer–DNA interactions,88 this PEO–PPO–PEO–PLL/α-CD/gene supramolecular hydrogel's in vitro gene release profile could be obtained upon heparin sodium treatment as well as later electrophoresis analysis. Experimental results showed that pDNA was released from the hydrogel matrix during formulation of the pDNA/PLL complex, and this hydrogel could sustainably release the embedded gene for more than 72 h. More interestingly, the increase of PEO–PPO–PEO–PLL or α-CD content could slow down pDNA release, because the elevated amount of PEO–PPO–PEO–PLL or α-CD might increase the density and strength of supramolecular hydrogels. In short, the results indicated that supramolecular hydrogels with good biocompatibility could be used for the potential sustained delivery and continuous release of genes.
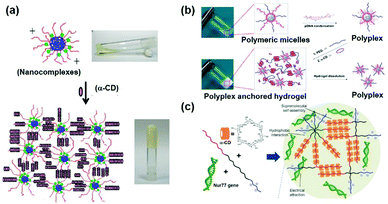 |
| Fig. 6 Cyclodextrin-based hydrogels for sustained gene release. (a) Synthetic routine of the PEO–PPO–PEO–PLL/α-CD/gene supramolecular hydrogel. Reproduced with permission.48 Copyright 2011, Elsevier. (b) Structure of the MPEG–PCL–PDMAEMA/α-CD/PEG/gene supramolecular hydrogel. Reproduced with permission.49 Copyright 2012, American Chemical Society. (c) Schematic illustration of the MPEG–PCL–PEI/α-CD supramolecular hydrogel with sustained gene release ability. Reproduced with permission.50 Copyright 2017, John Wiley and Sons. | |
Besides the copolymer of PEO–PPO–PEO, amphiphilic poly(ethylene glycol)–poly(ε-caprolactone) (PEG–PCL) block copolymers have also been utilized to form supramolecular structures with CDs.108 Further studies showed that PEG–PCL conjugated with cationic segments such as poly(2-(dimethylamino)ethyl methacrylate) (PDMAEMA) could exhibit excellent pDNA binding ability.89 By considering these advantages, Li et al. incubated the MPEG–PCL–PDMAEMA triblock copolymers with an α-CD and PEG mixture solution to form stable supramolecular polypseudorotaxane hydrogels as a sustained gene release vector (Fig. 6b).49 In this hydrogel system, PCL segments with high hydrophobicity could partially aggregate to form the interior of the micelle, while the cationic PDMAEMA segment could bind with DNA. And the hydrophilic and biocompatible MPEG chain could crosslink with α-CD to form a supramolecular network. This rheological hydrogel was successfully utilized as a gene carrier, while the embedded gene was released upon hydrogel erosion. Experimental results showed that this hydrogel could achieve more than 6 days’ continuous gene complex release, while the naked gene released from the α-CD/PEG hydrogel only lasted for up to 3 days. Furthermore, as shown in Fig. 6c, Liu et al. synthesized a supramolecular hydrogel which was formed by the combination of cationic MPEG–PCL–PEI with anionic pDNA and α-CD, owing to the threading of CDs onto PEG chains.50 Experimental results demonstrated that this supramolecular hydrogel could release the gene for up to 7 days in a sustained manner, and showed better biocompatibility and higher gene transfection ability in comparison with PEI alone, indicating its immense potential as a sustained gene delivery vector.
3. Potential therapeutic applications of cyclodextrin-based sustained gene release vectors
Gene therapy has shown excellent clinical effects and great potential in treating hereditary diseases or cancers, while its success heavily relies on the design of carriers to safely deliver therapeutic genes to targeted cells or organs.90–99 Compared with the common gene delivery vectors with only transient transfection ability, vectors with sustained gene release capacity might ensure gene transfection for a longer period of time which could be beneficial for high transfection efficiency or effective disease treatments. In detail, if the gene is delivered and continuously released in a carrier-encapsulated manner, it can effectively prolong the half-life of the therapeutic gene in the body and avoid rapid gene clearance, thereby maintaining high transfection efficiency, and clinically reduce the number of administrations, which can improve patient compliance.100 In the following section, the therapeutic applications of CD-based sustained gene release systems are summarized.
3.1 Advantages of cyclodextrin-based sustained gene release vectors
The sustained release of genes which can avoid repeated administration has far-reaching implications for clinical applications such as cancer treatment, tissue regeneration, or neurological repair.101 Although the most widely used gene vectors in current clinical trials are still viral vectors, such as the lentiviral vector,102 γ-rotavirus (γ-RV),103 or the adeno-associated virus (AAV) vector,104 they might exhibit very high integration efficiency and might cause the body to produce an immune response with unpredictable consequences. Non-viral vectors are superior to viral vectors in terms of biocompatibility. Therefore, different formulations of non-viral vectors were designed to achieve continuous delivery of genes, including nanospheres, scaffolds, etc. For example, Jeon et al. demonstrated that the addition of pDNA-containing poly(lactic-co-glycolic acid) (PLGA) microspheres to PLGA scaffolds enabled sustained release of genes for two months and maintained long-term transfection efficiency.105 Furthermore, Tahara et al. used chitosan modified PLGA nanospheres for gene delivery.106 The transfection efficiency of modified and unmodified nanospheres was compared and it was found that chitosan-modified PLGA nanospheres exhibited small interfering ribonucleic acid (siRNA) sustained delivery capacity (Fig. 7a) and higher transfection efficiency (Fig. 7b). Last but not least, as shown in Fig. 7c and d, Peng et al. continuously delivered DNA-binding protein inhibitor Id1-targeted small interfering ribonucleic acid (siRNA) to tumor cells by using a poly(ethyleneimine) (PEI)/collagen hydrogel, which showed sustained siRNA release ability and a better tumor suppressing effect.107
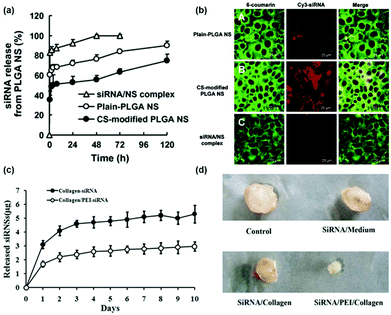 |
| Fig. 7 (a) Release curve of small interfering ribonucleic acid (siRNA) from PLGA-nanoparticles; (b) the laser scanning confocal microscope image represents the uptake of siRNA by A549 cells in different PLGA nanoparticles, where the siRNA is labeled with Cy3. Reproduced with permission.106 Copyright 2010, Elsevier. (c) Cumulative release of siRNA from collagen hydrogels with or without the addition of PEI to modulate siRNA release; (d) the inhibitory effect of siRNA in different formulations on tumor growth. Reproduced with permission.107 Copyright 2016, Springer. | |
Due to the good biocompatibility of CDs, CD-based gene delivery systems might be less toxic than other non-viral cationic gene delivery vectors. Furthermore, CD-based gene delivery systems had high transfection efficiencies and were able to control gene release rates by altering the composition of the delivery system. Hence, the CD-based sustained gene release system had great significance and application prospects in gene therapy. As typical examples, CD-based polypseudorotaxanes or supramolecular hydrogels could serve as gene delivery vehicles with the ability to continuously but not suddenly release therapeutic genes, upon slow dissociation or degradation of supramolecular structures. For instance, Motoyama et al. combined CD with PEG–PAMAM dendrimer to form polypseudorotaxane, which could control gene release by adjusting the dissolution medium volume and achieved sustained release of the gene for up to 72 h.73 Similarly, supramolecular hydrogels based on MPEG–PCL–PDMAEMA and α-CD could also be utilized to in vitro release embedded genes slowly for up to 6 days, as shown in Fig. 8.49 These experimental results showed that the gene release of CD-based hydrogels was mainly controlled by the depolymerization of CDs and the degradation or erosion of supramolecular hydrogels.
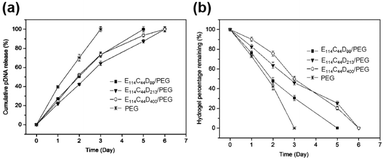 |
| Fig. 8 (a and b) In vitro release profile (a) of encapsulated pDNA from MPEG–PCL–PDMAEMA/CD/pDNA anchored supramolecular hydrogels and dissociation profile (b) of these supramolecular hydrogels as a function of incubation time at pH 7.4 and 37 °C. Reproduced with permission.49 Copyright 2012, American Chemical Society. | |
Since the release rate of genes in the CD based supramolecular system was affected by the strength of the system, a major feature of the CD-based sustained gene release system was that the gene release rate might be adjusted by changing the composition concentration within supramolecular systems. For example, the supramolecular system made of Pluronic F-68 and cationic poly(L-lysine) block copolymer F-68-PLL as well as α-CD could exhibit a tunable gene release rate, by adjusting the concentrations of α-CD or F-68-PLL (Fig. 9a).48 The experimental results showed that high concentrations of α-CD or F-68-PLL would slow down the rate of gene release, which might be due to stronger gelation upon increasing α-CD or F-68-PLL concentrations (Fig. 9b).
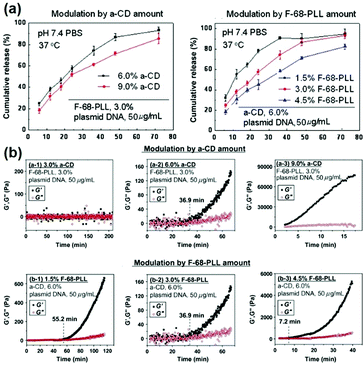 |
| Fig. 9 (a) In vitro release profiles for encapsulated plasmid DNA released from the in situ forming supramolecular hydrogels with different compositions in pH 7.4 phosphate buffer saline (PBS) at 37 °C; (b) the time dependence of elastic modulus (G′) and viscous modulus (G′′) for supramolecular complex systems containing F-68-PLL, α-CD and plasmid DNA. Reproduced with permission.48 Copyright 2012, Elsevier. | |
3.2 Cyclodextrin-based sustained gene release systems for DNA delivery
Gene therapy plays a very important role in cancer treatment. The delivery of anti-proliferation plasmid DNA or pDNA to tumor cells, by using non-viral gene carriers instead of viral vectors with limited inside cavity space which might not be suitable for pDNA with high molecular weight, represents a good idea in cancer treatment. As a typical example, Suzuki et al. used a non-viral gene carrier to deliver the interleukin 12 gene (IL-12) and allowed the overexpression of IL-12 proteins in tumor cells, which could effectively inhibit the cancer cell growth by immunomodulation.108 In the other case, Li et al. utilized a star shaped cationic polymer to deliver a tumor suppressor p53 gene into the animal body and significantly slowed down tumor progression, indicating the good prospects of pDNA based gene therapy for cancer treatment.109
It is also worth mentioning that, due to the transient and low transfection efficiency of many gene vectors as well as poor biocompatibility, the widespread use of pDNA based gene therapy has been limited. Hence, CD-based sustained gene delivery vectors might exhibit unique advantages. As a typical example, Liu et al. designed and synthesized a supramolecular hydrogel based on MPEG–PCL–PEI and α-CD to continuously deliver apoptosis resistant B-cell lymphoma-2 (Bcl-2) conversion gene (nuclear receptor Nur77 gene) in the form of pDNA to cancer cells as shown in Fig. 10a.50 The detailed mechanism involved mitochondria targeting by nuclear orphan receptor Nur77 and apoptosis induction due to the interaction of Nur77's ligand binding domain (LBD) and Bcl-2 protein, which resulted in Bcl-2's conformation change, exposure of the apoptotic BH3 domain, and transformation of Bcl-2 from a growth-promoting protein to a pro-apoptotic protein.110 More interestingly, this MPEG–PCL–PEI/α-CD supramolecular hydrogel was capable of releasing the Nur77 gene for up to one week (Fig. 10b) and exhibited higher gene transfection efficiency than branched PEI only. This supramolecular hydrogel/Nur77 gene complex was used to treat HepG2/Bcl-2 drug resistant tumors (Bcl-2 overexpressed HepG2 cell xenografted tumor) and exhibited more significant tumor growth inhibition capability than the chemotherapeutic treatment group alone. More importantly, the result showed that, when compared with the MPEG–PCL–PEI/Nur77 group, the MPEG–PCL–PEI/α-CD/Nur77 group had higher tumor inhibition capability against Bcl-2 drug-resistant cells, indicating higher gene transfection efficiency when the gene was continuously delivered in the hydrogel system, in comparison with polymer solution alone (Fig. 10c). In this unique sustained delivery system, the therapeutic gene was not released quickly and could be maintained at a high transfection concentration for a long time, which might increase the probability of gene transfection or expression integration and promote the apoptosis of tumor cells. These results indicated the great potential of CD-based sustained release systems in clinical applications and the “enemy into friend” strategy might be propitious for precise cancer therapy.
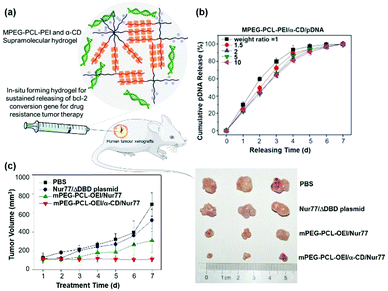 |
| Fig. 10 (a) Schematic diagram of the MPEG–PCL–PEI and α-CD based supramolecular hydrogel for the treatment of Bcl-2 expression elevated drug-resistant tumors. (b) In vitro gene release profile of MPEG–PCL–PEI/α-CD/pDNA supramolecular complexes at different weight ratios. (c) In vivo drug resistant tumor growth inhibition upon treatment of sustained gene release systems. Reproduced with permission.50 Copyright 2017, John Wiley and Sons. | |
In order to increase the selectivity of vectors or reduce side effects in sustained release systems, it might be valuable to modify the vectors with targeting ligands with the ability to recognize disease cells or tissues. For cancer cells, folic acid (FA) represents a popular ligand as the folate receptor (FR) is highly expressed on the membrane of most tumor cells.111 Studies have shown that attaching the FA group to the gene carriers might significantly increase tumor treatment efficiency. Therefore, Liu et al. further designed and synthesized a supramolecular hydrogel based on MPEG–PCL–PEI–FA and α-CD for sustained delivery of the Nur77 gene (Fig. 11a), in which the presence of FA groups endowed the polyplex with cancer cell targeting ability even with peritumoral injection of supramolecular hydrogels.51 As shown in Fig. 11b, in vivo experiment showed that this MPEG–PCL–PEI–FA/α-CD supramolecular hydrogel with FA targeting ligand modification could load and continuously release both Nur77 pDNA and chemotherapeutic paclitaxel in the form of a polyplex with a significant inhibitory effect on Bcl-2 drug-resistant tumor cells, indicating the great potential of targeted CD-based sustained gene release systems in tumor treatments.
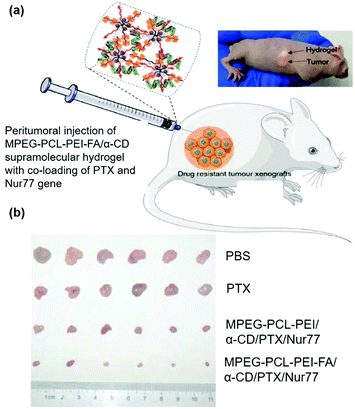 |
| Fig. 11 (a) Schematic diagram of the MPEG–PCL–PEI–FA and α-CD based supramolecular hydrogel with co-loading of PTX and Nur77 gene for the treatment of drug-resistant tumors; (b) in vivo tumor growth inhibition effect under different treatments. Reproduced with permission.51 Copyright 2018, John Wiley and Sons. | |
3.3 Cyclodextrin-based sustained gene release systems for RNA delivery
Last but not least, the cyclodextrin-based supramolecular hydrogel could not only be used to deliver pDNA, but also be utilized to carry and release small interfering RNA (siRNA) or short hairpin RNA (shRNA) in a sustained manner. On the one hand, RNA interference (RNAi) might be triggered by dsRNA (double-stranded RNA) and could induce a loss of function phenotype by inhibiting gene expression at the post-transcriptional level. On the other hand, shRNA is a hairpin structure consisting of two short inverted repeats joined by a loop and its hairpin structure could be removed to produce antisense siRNA for specific recognition and degradation of target messenger RNA (mRNA) by base complementary pairing, representing an interesting approach for gene therapy. For example, matrix metalloprotein-9 (MMP-9) was reported to be overexpressed in a variety of malignancies and was closely associated with tumor invasion and metastasis,112 while RNA interference technology could be employed to reduce the amount of MMP-9 protein to inhibit tumor growth and invasion.113 As a successful trial, Lin et al. designed a supramolecular system based on MPEG–PLLD–Arg and α-CD for gene delivery, and the synthesized hydrogel was used to combine with the pMMP-9 gene as shown in Fig. 12a.41 This system showed very good biocompatibility compared to the commercial non-viral gene carrier gold standard PEI with a molecular weight of 25 kDa (PEI-25k). In vitro release results indicated that this supramolecular hydrogel could continuously release the MMP-9 shRNA for more than 6 days (Fig. 12b). Western blot results showed that the expression of MMP-9 protein was obviously lower in MPEG–PLLD–Arg/MMP-9 shRNA treated cells than in the PBS treatment group, indicating that MPEG–PLLD–Arg/MMP-9 shRNA did reduce the amount of mRNA expression of MMP-9 in cancer cells. As shown in Fig. 12c, in vivo experiments showed that the tumor volume was significantly reduced in the hydrogel-treated group of MPEG–PLLD–Arg/α-CD/MMP-9 shRNA, indicating that the CD-based sustained gene release system could be utilized for RNA delivery and control its release manner for improved therapeutics.
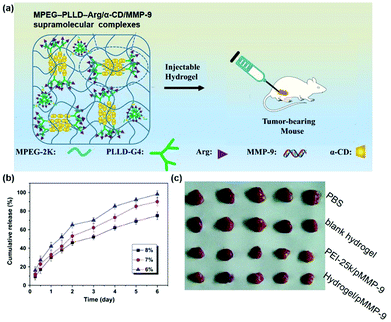 |
| Fig. 12 (a) Schematic diagram of the MPEG–PLLD–Arg/α-CD/MMP-9 shRNA supramolecular hydrogel for the treatment of tumors; (b) in vitro gene release profile of MPEG–PLLD–Arg/α-CD/MMP-9 supramolecular complexes; (c) in vivo tumor growth inhibition effect with various treatments. Reproduced with permission.41 Copyright 2017, Elsevier. | |
4. Conclusion and perspectives
Since gene therapy has shown remarkable therapeutic effects in clinical trials and owing to the importance of carrier design in successful gene therapy, attention has been paid to searching for excellent gene carriers with excellent biocompatibility and gene transfection capacity. Furthermore, the design of delivery systems to achieve sustained gene release and satisfactory gene transfection efficiency might circumvent the repeated administration and reduce side effects. Among the sustained gene delivery systems, evidence suggests that using cyclodextrins (CDs) to construct gene carriers has significant advantages due to their hydrophilic property and biocompatibility. In this review, we summarized recent interesting advances concerning CD-based sustained gene delivery systems. CDs can self-assemble with suitable polymer segments to form supramolecular structures by host–guest interactions, while the most successful attempt was the formation of supramolecular hydrogels. In the case of supramolecular hydrogels, CDs could serve as a physical cross-linker of the polyplex between the cationic polymer and therapeutic gene (either DNA or RNA) and might avoid the usage of chemical cross-linkers or photo-initiators with high toxicity. More importantly, the de-threading of CD cyclic molecules from supramolecular hydrogels could lead to release of the gene in the form of a polyplex rather than in a naked form, which might ensure high transfection efficiency during the sustained release process. More interestingly, the gradual erosion and breakdown of hydrogels allows the gene to be released slowly, while the release speed of the encapsulated gene could be adjusted by modulating the CD concentration and the transfection efficiency might be influenced by utilizing different cationic polymers or changing the polymer length. Last but not least, CD-based delivery systems have the advantages of easy functionalization, because CDs can be further modified by host–guest interactions to form inclusion complexes without affecting the mutuality or particle morphology of the cationic polymer/gene polyplex. In short, CD-based sustained gene delivery systems might show great potential in gene therapy.
In future studies, the CD based structures might be worth exploring for their potential to experience physical modifications for achieving active targeting to disease sites. Furthermore, few CD-based sustained delivery system with stimulus triggered release ability has been reported, in terms of pH, temperature, photo or light stimulus, to achieve on-demand therapeutics. Current reports on applications of CD-based sustained gene release systems have mainly focused on improvement of tumor therapy, and they might also be valuable for other treatments in terms of tissue regeneration, angiogenesis, and genetic defects or anomalies which might lead to more contributions in long term gene therapy.
Conflicts of interest
There are no conflicts to declare.
Acknowledgements
The authors would like to acknowledge the support of the National Natural Science Foundation of China (81773661) and the Fundamental Research Funds for the Central Universities (20720170066), and express gratitude to the A*STAR Grant for support of this project.
References
- Y. Xiang, N. N. L. Oo, J. P. Lee, Z. Li and X. J. Loh, Drug Discovery Today, 2017, 22, 1318–1335 CrossRef CAS PubMed.
- Y. Hu, Y. Li and F. J. Xu, Acc. Chem. Res., 2017, 50, 281–292 CrossRef CAS PubMed.
- Y. Hu, C. Wen, L. Z. Song, N. N. Zhao and F. J. Xu, J. Controlled Release, 2017, 255, 154–163 CrossRef CAS.
- F. J. Xu, Prog. Polym. Sci., 2018, 78, 56–91 CrossRef CAS.
- C. Wen, Y. Hu, C. Xu and F. J. Xu, Acta Biomater., 2016, 32, 110–119 CrossRef CAS PubMed.
- H. Q. Song, W. T. Pan, R. Q. Li, B. R. Yu, W. J. Liu, M. Yang and F. J. Xu, Small, 2018, 14, 1703152 CrossRef PubMed.
- H. Q. Song, Y. Qi, R. Q. Li, G. Cheng, N. Zhao and F. J. Xu, Polym. Chem., 2018, 9, 2281–2289 RSC.
- Q. Dou, X. Fang, S. Jiang, P. L. Chee, T.-C. Lee and X. J. Loh, RSC Adv., 2015, 5, 46817–46822 RSC.
- S. Jiang, D. Kai, Q. Q. Dou and X. J. Loh, J. Mater. Chem. B, 2015, 3, 6897–6904 RSC.
- S. Jiang, Y. Z. Poh and X. J. Loh, RSC Adv., 2015, 5, 71322–71328 RSC.
- C. K. Liu, Q. Dou, S. S. Liow, J. N. Kumar and X. J. Loh, Aust. J. Chem., 2016, 69, 363–371 CrossRef CAS.
- X. J. Loh, T.-C. Lee, Q. Dou and G. R. Deen, Biomater. Sci., 2016, 4, 70–86 RSC.
- X. J. Loh and Y.-L. Wu, Chem. Commun., 2015, 51, 10815–10818 RSC.
- X. J. Loh, Z.-X. Zhang, K. Y. Mya, Y.-l. Wu, C. B. He and J. Li, J. Mater. Chem., 2010, 20, 10634–10642 RSC.
- L. Naldini, Nature, 2015, 526, 351–360 CrossRef CAS PubMed.
- H. Cheng, Z. Wu, C. Wu, X. Wang, S. S. Liow, Z. Li and Y.-L. Wu, Mater. Sci. Eng., C, 2018, 83, 210–217 CrossRef CAS PubMed.
- C. Zheng, H. Gao, D.-P. Yang, M. Liu, H. Cheng, Y.-l. Wu and X. J. Loh, Mater. Sci. Eng., C, 2017, 74, 110–116 CrossRef CAS PubMed.
- S. S. Liow, Q. Dou, D. Kai, Z. Li, S. Sugiarto, C. Y. Y. Yu, R. T. K. Kwok, X. Chen, Y. L. Wu and S. T. Ong, Small, 2017, 13, 1603404 CrossRef PubMed.
- P. Cai, W. R. Leow, X. Wang, Y.-L. Wu and X. Chen, Adv. Mater., 2017, 29, 1605529 CrossRef PubMed.
- Y. L. Wu, X. Chen, W. Wang and X. J. Loh, Macromol. Chem. Phys., 2016, 217, 175–188 CrossRef CAS.
- D. Fischer, T. Bieber, Y. Li, H. P. Elsässer and T. Kissel, Pharm. Res., 1999, 16, 1273–1279 CrossRef CAS.
- H. Lu, Y. Dai, L. Lv and H. Zhao, PLoS One, 2014, 9, e84703 CrossRef PubMed.
- N. J. Meilander, M. K. Pasumarthy, T. H. Kowalczyk, M. J. Cooper and R. V. Bellamkonda, J. Controlled Release, 2003, 88, 321 CrossRef CAS.
- T. Segura, P. H. Chung and L. D. Shea, Biomaterials, 2005, 26, 1575–1584 CrossRef CAS.
- D. Lee, W. Zhang, S. A. Shirley, X. Kong, G. R. Hellermann, R. F. Lockey and S. S. Mohapatra, Pharm. Res., 2007, 24, 157 CrossRef CAS PubMed.
- H. J. Kong, E. S. Kim, Y. C. Huang and D. J. Mooney, Pharm. Res., 2008, 25, 1230–1238 CrossRef CAS PubMed.
- X. J. Loh and Y. L. Wu, Chem. Commun., 2015, 51, 10815–10818 RSC.
- R. M. Raftery, E. G. Tierney, C. M. Curtin, S. A. Cryan and F. J. O'Brien, J. Controlled Release, 2015, 210, 84 CrossRef CAS.
- H. Storrie and D. J. Mooney, Adv. Drug Delivery Rev., 2006, 58, 500–514 CrossRef CAS PubMed.
- C. Ding and Z. Li, Mater. Sci. Eng., C, 2017, 76, 1440–1453 CrossRef CAS PubMed.
- J. Y. Hwang, Z. Li and X. J. Loh, RSC Adv., 2016, 6, 70592–70615 RSC.
- Z. Li, Ther. Delivery, 2017, 8, 597–600 CrossRef CAS PubMed.
- M. L. Hedley, J. Curley and R. Urban, Nat. Med., 1998, 4, 365–368 CrossRef CAS.
- J. P. Nam and J. W. Nah, Carbohydr. Polym., 2016, 135, 153 CrossRef CAS PubMed.
- Y. Yang, H. Zhao, Y. Jia, Q. Guo, Y. Qu, J. Su, X. Lu, Y. Zhao and Z. Qian, Sci. Rep., 2016, 6, 21402 CrossRef CAS PubMed.
- S. S. Lee, P. Hughes, A. D. Ross and M. R. Robinson, Pharm. Res., 2010, 27, 2043–2053 CrossRef CAS.
- X. Fan, X. Wang, M. Cao, C. Wang, Z. Hu, Y.-L. Wu, Z. Li and X. J. Loh, Polym. Chem., 2017, 8, 5611–5620 RSC.
- X. Liu, X. Fan, L. Jiang, X. J. Loh, Y.-L. Wu and Z. Li, J. Mater. Chem. B, 2018, 6, 5488–5498 RSC.
- W. Li, X. Fan, X. Wang, X. Shang, Q. Wang, J. Lin, Z. Hu and Z. Li, Mater. Sci. Eng., C, 2018, 91, 688–695 CrossRef CAS PubMed.
- N. Symens, A. Mendez-Ardoy, A. Diaz-Moscoso, E. Sanchez-Fernandez, K. Remaut, J. Demeester, J. M. G. Fernandez, S. C. De Smedt and J. Rejman, Bioconjugate Chem., 2012, 23, 1276–1289 CrossRef CAS.
- Q. Lin, Y. Yang, H. Qian, G. Zhong, L. Tao, J. Xu, J. Wu, T. B. Kirk, M. Dong and X. Wei, Acta Biomater., 2016, 49, 456–471 CrossRef.
- H. Chen, X. Liu, Y. Dou, B. He, L. Liu, Z. Wei, J. Li, C. Wang, C. Mao and J. Zhang, Biomaterials, 2013, 34, 4159–4172 CrossRef CAS PubMed.
- H. Gonzalez, S. J. Hwang and M. E. Davis, Bioconjugate Chem., 1999, 10, 1068 CrossRef CAS.
- C. Yang, X. Wang, H. Li, S. H. Goh and J. Li, Biomacromolecules, 2007, 8, 3365–3374 CrossRef CAS PubMed.
- C. Yang, X. Wang, H. Li, J. L. Ding, D. Y. Wang and J. Li, Polymer, 2009, 50, 1378–1388 CrossRef CAS.
- K. Motoyama, K. Hayashida, T. Higashi and H. Arima, Bioorg. Med. Chem., 2012, 20, 1425–1433 CrossRef CAS PubMed.
- J. Deng, Y. Luo and L. M. Zhang, Soft Matter, 2011, 7, 5944–5947 RSC.
- D. Ma, H. B. Zhang, D. H. Chen and L. M. Zhang, J. Colloid Interface Sci., 2011, 364, 566–573 CrossRef CAS PubMed.
- Z. Li, H. Yin, Z. Zhang, K. L. Liu and J. Li, Biomacromolecules, 2012, 13, 3162 CrossRef CAS.
- X. Liu, X. Chen, M. X. Chua, Z. Li, X. J. Loh and Y. L. Wu, Adv. Healthcare Mater., 2017, 6, 1700159 CrossRef.
- X. Liu, Z. Li, J. L. Xian, K. Chen, Z. Li and Y. L. Wu, Macromol. Rapid Commun., 2018, 1800117 CrossRef.
- H. Cheng, X. Fan, C. Wu, X. Wang, L.-J. Wang, X. J. Loh, Z. Li and Y.-L. Wu, Macromol. Rapid Commun., 2018, 39, 1800207 CrossRef.
- S. J. Hwang, N. C. Bellocq and M. E. Davis, Bioconjugate Chem., 2001, 12, 280–290 CrossRef CAS PubMed.
- Y. Hu, K. Cai, Z. Luo and R. Hu, Adv. Eng. Mater., 2010, 12, B18–B25 CrossRef.
- D. Ma, H. B. Zhang, Y. Y. Chen, J. T. Lin and L. M. Zhang, J. Colloid Interface Sci., 2013, 405, 305–311 CrossRef CAS PubMed.
- C. Yang, H. Li, S. H. Goh and J. Li, Biomaterials, 2007, 28, 3245–3254 CrossRef CAS PubMed.
- F. J. Xu, Z. X. Zhang, Y. Ping, J. Li, E. T. Kang and K. G. Neoh, Biomacromolecules, 2009, 10, 285 CrossRef CAS PubMed.
- N. C. Bellocq, S. H. Pun, G. S. Jensen and M. E. Davis, Bioconjugate Chem., 2003, 14, 1122–1132 CrossRef CAS PubMed.
- S. H. Pun, F. Tack, N. C. Bellocq, J. Cheng, B. H. Grubbs, G. S. Jensen, M. E. Davis, M. Brewster, M. Janicot and B. Janssens, Cancer Biol. Ther., 2004, 3, 641–650 CrossRef CAS.
- M. E. Davis, J. E. Zuckerman, C. H. J. Choi, D. Seligson, A. Tolcher, C. A. Alabi, Y. Yun, J. D. Heidel and A. Ribas, Nature, 2010, 464, 1067–1070 CrossRef CAS PubMed.
- S. Wang, K. J. Chen, T. H. Wu, H. Wang, W. Y. Lin, M. Ohash, P. Y. Chiou and H. R. Tseng, Angew. Chem., Int. Ed., 2010, 49, 3777–3781 CrossRef CAS PubMed.
- Y. Ping, Q. Hu, G. Tang and J. Li, Biomaterials, 2013, 34, 6482 CrossRef CAS PubMed.
- T. Ooya, H. S. Choi, A. Yamashita, N. Yui, Y. Sugaya, A. Kano, A. Maruyama, H. Akita, R. Ito and K. Kogure, J. Am. Chem. Soc., 2006, 128, 3852–3853 CrossRef CAS PubMed.
- Y. Zhou, H. Wang, C. Wang, Y. Li, W. Lu, S. Chen, J. Luo, Y. Jiang and J. Chen, Mol. Pharmaceutics, 2012, 9, 1067–1076 CrossRef CAS PubMed.
- N. Yui and T. Ooya, Chem. – Eur. J., 2006, 12, 6730–6737 CrossRef CAS PubMed.
- A. Harada, J. Li and M. Kamachi, Macromolecules, 1993, 26, 5698–5703 CrossRef CAS.
- J. Li, A. Harada and M. Kamachi, Bull. Chem. Soc. Jpn., 2006, 67, 2808–2818 CrossRef.
- J. Li, C. Yang, H. Li, X. Wang, S. H. Goh, J. L. Ding, D. Y. Wang and K. W. Leong, Adv. Mater., 2010, 18, 2969–2974 CrossRef.
- A. Yamashita, N. Yui, T. Ooya, A. Kano, A. Maruyama, H. Akita, K. Kogure and H. Harashima, Nat. Protoc., 2006, 1, 2861–2869 CrossRef CAS PubMed.
- C. Yang, X. Wang, H. Z. Li, E. Tan, C. T. Lim and J. Li, J. Phys. Chem. B, 2009, 113, 7903–7911 CrossRef CAS PubMed.
- A. Hashidzume, H. Yamaguchi and A. Harada, Acc. Chem. Res., 2001, 34, 456–464 CrossRef.
- T. Higashi, F. Hirayama, S. Misumi, H. Arima and K. Uekama, Biomaterials, 2008, 29, 3866 CrossRef CAS PubMed.
- K. Motoyama, K. Hayashida and H. Arima, Chem. Pharm. Bull., 2011, 59, 476–479 CrossRef CAS PubMed.
- F. Kihara, H. Arima, T. Tsutsumi, F. Hirayama and K. Uekama, Bioconjugate Chem., 2002, 13, 1211–1219 CrossRef CAS PubMed.
- J. M. Rosiak and F. Yoshii, Nucl. Instrum. Methods Phys. Res., 1999, 151, 56–64 CrossRef CAS.
- F. Ullah, M. B. H. Othman, F. Javed, Z. Ahmad and H. M. Akil, Mater. Sci. Eng., C, 2015, 57, 414 CrossRef CAS PubMed.
- T. Miyata, N. Asami and T. Uragami, Nature, 1999, 399, 766–769 CrossRef CAS PubMed.
- X. Y. Dai, Y. Y. Zhang, L. N. Gao, T. Bai, W. Wang, Y. L. Cui and W. G. Liu, Adv. Mater., 2015, 27, 3566–3571 CrossRef CAS PubMed.
- R. Dong, Y. Su, S. Yu, Y. Zhou, Y. Lu and X. Zhu, Chem. Commun., 2013, 49, 9845–9847 RSC.
- K. Miyata, N. Nishiyama and K. Kataoka, Chem. Soc. Rev., 2012, 41, 2562–2574 RSC.
- X. Zhang, J. Huang, P. R. Chang, J. Li, Y. Chen, D. Wang, J. Yu and J. Chen, Polymer, 2010, 51, 4398–4407 CrossRef CAS.
- J. Li and X. J. Loh, Adv. Drug Delivery Rev., 2008, 60, 1000–1017 CrossRef CAS PubMed.
- I. I. A. Hashim, T. Higashi, T. Anno, K. Motoyama, A. E. H. Abd-Elgawad, M. H. El-Shabouri, T. M. Borg and H. Arima, Int. J. Pharm., 2010, 392, 83–91 CrossRef PubMed.
- J. Xu, X. Li and F. Sun, Acta Biomater., 2010, 6, 486–493 CrossRef CAS PubMed.
- R. Kumar, P. Chaudhary, S. Nimesh and R. Chandra, Cheminform, 2006, 37, 453–456 Search PubMed.
- J. Li, X. Li, Z. Zhou, X. Ni and K. W. Leong, Macromolecules, 2001, 34, 7236–7237 CrossRef CAS.
- X. Ni, A. Cheng and J. Li, J. Biomed. Mater. Res., Part A, 2010, 88, 1031–1036 Search PubMed.
- Y. Xu and F. C. Szoka, Biochemistry, 1996, 35, 5616–5623 CrossRef CAS PubMed.
- W. L. Zhang, J. L. He, Z. Liu, P. H. Ni and X. L. Zhu, J. Polym. Sci., Part A: Polym. Chem., 2010, 48, 1079–1091 CrossRef CAS.
- H. Cheng, X. Fan, X. Wang, E. Ye, X. J. Loh, Z. Li and Y.-L. Wu, Biomacromolecules, 2018, 19, 1926–1938 CrossRef CAS PubMed.
- P. Cai, X. Zhang, M. Wang, Y.-L. Wu and X. Chen, ACS Nano, 2018, 12, 5078–5084 CrossRef CAS PubMed.
- X. Wang, S. S. Liow, Q. Wu, C. Li, C. Owh, Z. Li, X. J. Loh and Y.-L. Wu, Macromol. Biosci., 2017, 17, 1700186 CrossRef PubMed.
- Z. Li, X. Liu, X. Chen, M. X. Chua and Y.-L. Wu, Mater. Sci. Eng., C, 2017, 76, 66–72 CrossRef CAS PubMed.
- X. Fan, H. Cheng, X. Wang, E. Ye, J. L. Xian, Y. L. Wu and Z. Li, Adv. Healthcare Mater., 2017, 7, 1701143 CrossRef PubMed.
- Y.-L. Wu, H. Wang, Y.-K. Qiu and X. J. Loh, RSC Adv., 2016, 6, 44506–44513 RSC.
- Y. L. Wu, H. Wang, Y. K. Qiu, S. S. Liow, Z. Li and X. J. Loh, Adv. Healthcare Mater., 2016, 5, 2679–2685 CrossRef CAS PubMed.
- X. Chen, Y.-K. Qiu, C. Owh, X. J. Loh and Y.-L. Wu, Nanoscale, 2016, 8, 18876–18881 RSC.
- Y.-L. Wu, H. Yin, F. Zhao and J. Li, Adv. Healthcare Mater., 2013, 2, 297–301 CrossRef CAS PubMed.
- Y.-L. Wu, N. Putcha, K. W. Ng, D. T. Leong, C. T. Lim, S. C. J. Loo and X. Chen, Acc. Chem. Res., 2013, 46, 782–791 CrossRef CAS PubMed.
- S. Seth, R. Johns and M. V. Templin, Ther. Delivery, 2012, 3, 245–261 CrossRef CAS.
- P. Philippidou, C. M. Walsh, J. Aubin, L. Jeannotte and J. S. Dasen, Nat. Neurosci., 2012, 15, 1636 CrossRef CAS PubMed.
- A. S. Haceinbey, H. B. Gaspar, J. Blondeau, L. Caccavelli, S. Charrier, K. Buckland, C. Picard, E. Six, N. Himoudi and K. Gilmour, JAMA, 2015, 313, 1550–1563 CrossRef PubMed.
- J. N. Kochenderfer, M. E. Dudley, S. H. Kassim, R. P. Somerville, R. O. Carpenter, M. Stetlerstevenson, J. C. Yang, G. Q. Phan, M. S. Hughes and R. M. Sherry, J. Clin. Oncol., 2015, 33, 540–549 CrossRef CAS PubMed.
- J. W. B. Bainbridge, M. S. Mehat, V. Sundaram, S. J. Robbie, S. E. Barker, C. Ripamonti, A. Georgiadis, F. M. Mowat, S. G. Beattie and P. J. Gardner, N. Engl. J. Med., 2015, 372, 1887 CrossRef PubMed.
- O. Jeon, M. Krebs and E. Alsberg, J. Biomed. Mater. Res., Part A, 2011, 98, 72–79 CrossRef PubMed.
- K. Tahara, H. Yamamoto, N. Hirashima and Y. Kawashima, Eur. J. Pharm. Biopharm., 2010, 74, 421–426 CrossRef CAS PubMed.
- P. Hao, H. Yang, L. Song, Z. Zheng, J. Sun, Y. Du, K. Lu, L. Tao, A. Yin and J. Xu, J. Exp. Clin. Cancer Res., 2016, 35, 1–9 CrossRef PubMed.
- R. Suzuki, E. Namai, Y. Oda, N. Nishiie, S. Otake, R. Koshima, K. Hirata, Y. Taira, N. Utoguchi and Y. Negishi, J. Controlled Release, 2010, 142, 245–250 CrossRef CAS PubMed.
- Y. Li, B. Xu, T. Bai and W. Liu, Biomaterials, 2015, 55, 12–23 CrossRef PubMed.
- B. Z. Lin, S. K. Kolluri, F. Lin, W. Liu, Y. H. Han, X. H. Cao, M. I. Dawson, J. C. Reed and X. K. Zhang, Cell, 2004, 116, 527–540 CrossRef CAS PubMed.
- L. Xu, Q. Bai, Z. Xin and Y. Hu, J. Controlled Release, 2017, 252, 73–82 CrossRef CAS PubMed.
- B. Schmalfeldt, D. Prechtel, K. Härting, K. Späthe, S. Rutke, E. Konik, R. Fridman, U. Berger, M. Schmitt and W. Kuhn, Clin. Cancer Res., 2001, 7, 2396–2404 CAS.
- S. S. Lakka, C. N. Gondi, W. C. Olivero, D. H. Dinh, M. Gujrati and J. S. Rao, Oncogene, 2004, 23, 4681–4689 CrossRef CAS PubMed.
|
This journal is © the Partner Organisations 2019 |