DOI:
10.1039/D2MA00410K
(Review Article)
Mater. Adv., 2022,
3, 6125-6141
Injectable macromolecule-based calcium phosphate bone substitutes
Received
11th April 2022
, Accepted 22nd June 2022
First published on 28th June 2022
Abstract
Injectable bone substitutes (IBS) represent compelling options for bone regenerative medicine as they can be used to optimally fill a complex bone defect through minimally invasive intervention. Since their discovery, calcium phosphate (CaP) based IBS have never stopped evolving to match the diverse clinical needs. The main challenge is to combine the desired physico-chemical and handling properties of the IBS to an optimal induced biological response. This cannot unfortunately be achieved with CaP biomaterials alone, hence a growing use of polymers and organic macromolecules as additives. To properly understand the ins and outs, a didactic classification of IBS is proposed in this review, which compiles the past, present and future developments of IBS. Class I IBS, taking advantage of ceramic particles or granules as the support for bone formation, are already commercialized and widely employed in clinics. In contrast, Class II IBS, where cements serve as a stiff matrix for the development of mineralized tissues, associated with polymers, are still in their early stages but have shown significant improvements versus Class I products. These innovative Class II IBS will be the second focal point of this review.
1. Introduction
Despite its inert appearance, bone is a highly dynamic organ that exerts important mechanical (e.g., a supportive frame, locomotion, and protection), metabolic (e.g., homeostasis), and synthetic (e.g., haematopoiesis) functions.1 Unlike skin and other soft organs, bone has the innate ability to regenerate without scarring by means of complex biological cascades.2,3 However, bone maintenance and/or healing can be impaired as a result of age,4,5 lifestyle,6–8 pathological conditions,9–11 medical treatment,12–14 and injury.15–17 To help bone regeneration, strengthen skeletal integrity, stabilize an implanted bone prosthesis, or relieve joint pain, surgical strategies that are often coupled to bone grafting have been developed. As such, bone represents the second most frequently transplanted tissue after blood,18,19 with musculoskeletal pathologic conditions affecting more than 100 and 120 million adults in the U.S.20 and Europe,21 respectively, (i.e. ≈50% and 25%, respectively, of the adult populations). To date, the vast majority of bone grafting procedures have been performed with autologous bone (i.e., harvesting of the patient's own bone from a healthy site), with well-known associated limitations: additional burden for patients whose health is already compromised, infection risk, morbidity, graft resorption, weakness of the harvesting site, variable quality, limited availability, etc.22,23 To improve the overall care of patients, most research has been geared toward the development of alternative treatments. Therefore, in the past 20 years, there has been increasing interest in synthetic bone substitutes designed for a given medical indication24–29 or even a specific patient(s). Among these substitutes, injectable bone substitutes (IBS) have garnered considerable attention due to their tailorability and consequently a wide range of potential clinical applications.24 Unlike the most common synthetic substitutes (i.e., calcium phosphate porous blocks and granules), IBS have significant advantages of being fully adapted for minimally invasive procedures,27,28 to perfectly fill complex defects and to self-set in situ, to eventually display “biomimetic” features, and to eventually include active molecules of cells in their formulations.
Most of the commercial IBS currently in clinical use are based on calcium phosphate (CaP) ceramic particles or sintered microporous granules often blended with the viscous solution of macromolecules (Table 1). Unfortunately, these formulations (referred hereafter as Class I) suffer from poor mechanical properties and can leak into the trabecular bone or outside the defect, as documented in the informative recent reviews of Lodoso-Torrecilla et al.20 and Schröter et al.24 To tackle these issues, extensive research has been carried out on composite self-setting, coupling inorganic cementitious phases (e.g., calcium phosphates, CaP) to innovative biopolymers, which we refer to as Class II. Very few Class II materials are currently in clinical use (Table 1), although the number of preclinical and research studies is increasing as a result of the use of “biomimetic” cement phases.
Table 1 Class I and Class II injectable and extrudable commercialized materials currently on the market (TCP = tricalcium phosphate, HA = hydroxyapatite, CaP = calcium phosphate, CDHA = calcium deficient hydroxyapatite, HPMC = hydropropylmethylcellulose, rhBMP = recombinant human bone morphogenetic protein)
Commercial name and manufacturer |
Material class |
Inorganic phase |
Organic phase |
Application |
Characteristics |
Clinical trial number and reference |
MBCP-Gel/In'Oss™ (Biomatlante) |
I |
CaP granules |
HPMC polymer solution |
Filling osseous defects of various origins |
Ready to use, cohesivity/putty |
NCT00740311; (ref. 30) |
ExcelOs-inject (CGBIO) |
I |
Beta TCP granules |
Biodegradable hydrogel with or without rhBMP-2 |
Spinal fusion |
Ready to use, injectable, cohesivity |
NCT02714829; (ref. 31) |
Mastergraft (Medtronic) |
I |
β-TCP + HA |
Bovine collagen matrix |
Spinal fusion |
Putty |
NCT01491542; (ref. 32) |
Vitoss (Stryker) |
I |
β-TCP granules |
Bone marrow aspirate |
Spinal pathologies |
Putty ultra-porous, flexible |
NCT03509480; (ref. 33) |
ChronOS (DePuy Synthes) |
I |
β-TCP granules |
Autologous blood and/or bone marrow |
Tibial plateau fractures |
Putty |
NCT02056834; (ref. 34) |
ChronOS Inject (DePuy Synthes) |
I |
Brushite matrix and (TCP) granules |
|
Proximal tibial fractures |
Injectable, degradable |
NCT02056834; (ref. 35) |
CERAMENT® (Bone Void Filler) |
I |
HA and calcium sulfate cement |
|
Tibial plateau fractures |
Injectable, degradable |
NCT01828905; (ref. 36) |
Norian® Drillable (DePuy Synthes) |
II |
Carbonated apatite cement |
Bioresorbable polylactide/glycolide copolymer fibres |
Tibial fractures |
Mechanical resistance 24 hours after injection |
NCT01132508; (ref. 37) |
Graftys quickset/HBS (Graftys) |
II |
CDHA cement |
Powder of HPMC polymer |
Bone disease, bone fractures |
Porosity |
NCT02575352; (ref. 38) |
In this review, we discriminated Class I and Class II IBS and detailed their specifications and features in the first section. Besides, a particular focus is on Class II IBS and their future developments. IBS improvements achieved through the addition of polymers and macromolecules will specifically be highlighted.
2. Injectable bone substitutes (IBS): classifications
There is currently no classification to rank the existing and developing CaP IBS composites for bone regeneration. There are several classification options:
– The first way to sort them may be by their final formulation, i.e., the composition of the inorganic phases (after setting for hydraulic cements) and the composition of a possible organic phase.
– The second way to classify them relies on their ability to remain in a cohesive viscous state (non-hardening IBS) or to set (hardening IBS); whether or not the organic (reticulation, physicochemical interactions) or/and inorganic phase (acid–base reaction) is responsible for the hardening mechanism.
– The third way may be their interface with the host environment, i.e., the presence or absence of interconnected macropores (i.e., pores >100 μm) in addition to micro- and nano-porosity.
– Finally, the fourth and more clinically oriented way could be based on their practicality in the operating room: ‘ready-to-use’ versus ‘preparation required before use’ composite IBS.
In an effort to harmonize the current nomenclature, we humbly propose the following classification based on the notion of Class I and Class II materials. Class I IBS, which are already in widespread clinical use, include CaP particles and sintered granules (ceramic) embedded in a non-hardening (polymeric liquid viscous solution) or hardening (polymeric viscous solution that becomes a hydrogel or mineral fast resorbable cement) matrix. Often, the matrix tends to be rapidly washed off or biodegraded, leaving only the particles as a scaffold for the development of new bone tissue. Still in preclinical development, Class II IBS associate the calcium phosphate cement (CPC) with organic phases; the cementitious inorganic phase setting in situ provides a mechanically stable and porous environment that becomes replaced by new bone over time. Detailed information about Class I and Class II composite IBS is provided in the sections below, as illustrated in Fig. 1, and their main characteristics are summed up in Table 2.
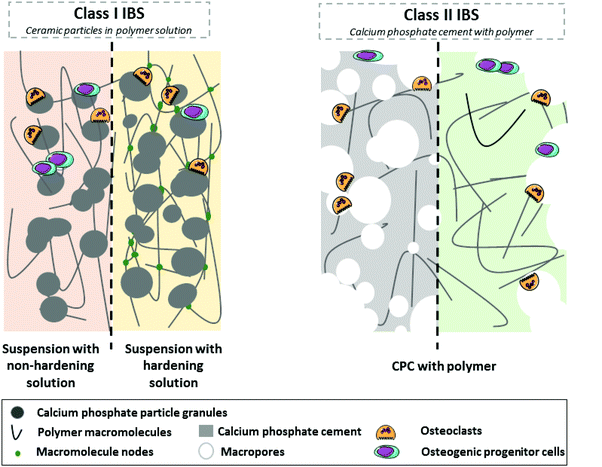 |
| Fig. 1 The two classes of IBS present different osteoconduction mechanisms: for Class I, calcium phosphate supports are made of divided ceramics (thermally-sintered particles, resulting in high density materials); for Class II, calcium phosphate supports are made of bulk cements (soft chemistry route to interlinked particles, resulting in low-density materials showing macropores). | |
Table 2 Advantages and disadvantages of Class I and II injectable bone substitutes
Class |
Class I |
Class II |
Ceramic particles in the polymer solution |
Calcium phosphate cement with polymer |
Associated with… |
Non-hardening matrix |
Hardening matrix |
Polymer (hydrogel or solution) |
Organic matrix |
Resorbable mineral matrix |
Comparison versus pure inorganic cement.
|
Advantagesa |
• ↑ Injectability |
• ↑↑ Injectability |
• ↑ Injectability |
• ↑↑ Injectability |
• ↑ Cell-material interactions |
• ↑ Cell-material interactions |
• No material leakage |
• No material leakage |
• ↑ Cohesivity |
• ↑↑ Cohesivity |
• Stiff bioactive formulations |
• Stiff bioactive formulations |
|
• No material leakage |
• Biodegradation of the matrix |
• ↑↑ Mechanical properties |
|
• Controlled release of substances |
• ↑↑ Mechanical properties |
• ↑↑ Osteoconductivity (e.g., due to macroporosity) |
|
|
|
• ↑ Potential to stimulate bone growth |
|
|
|
• Controlled release of substances |
|
Disadvantagesa |
• Material leakage |
• Limited particle (bio)degradation |
• Low potential to simulate bone |
• Inadequate mechanical properties due to macroporous network |
• Low potential to simulate bone formation |
• Low potential to simulate bone formation |
• Limited particle (bio)degradation |
|
• Limited particle (bio)degradation |
• Poor mechanical properties |
|
|
• Poor mechanical properties |
|
|
|
2.1 Class I IBS: ceramic particles in a matrix
2.1.1 Ceramic particles with a non-hardening organic matrix.
This Class I subclass comprises ceramic granules of calcium phosphate (thermally sintered), which support osteoconduction, associated with a non-hardening organic matrix (polymer solution). The design of these injectable bone substitute (IBS) composites was first reported in early 1995 by Weiss et al.,39 who aimed to combine the relevant biological properties of CaP-sintered granules with the viscous features of a hydrophilic polymer in solution, resulting in an injectable formulation.40 More precisely, biphasic calcium phosphate macroporous granules (hydroxyapatite HAP/β-tricalcium phosphate β-TCP) were included in a hydroxypropyl methylcellulose (HPMC) matrix, thereby providing tailorable IBS (e.g., HAP/β-TCP ratio, %wt polymer).41–43 Once in vitro assays confirmed the biocompatibility of this formulation,44 the biological response induced by this ready-to-use injectable calcium phosphate ceramic suspension (ICPCS) was evaluated in various animal models.26,45–55 Bone developed rapidly within the interconnected macroporous network of granules (as illustrated in Fig. 2), rapidly providing sufficient mechanical properties to this initially weak construct.56 Alternative formulations have been developed, such as by either switching the inorganic filler with another natural version of synthetic calcium phosphate (e.g.), demineralized bone matrix57–59 or by changing the nature of the viscous carrier (e.g., hyaluronic acid,60 gelatin,61 or collagen62). Comparable overall bone regeneration was observed. Despite the interesting osteogenic properties, the main issues with suspension-based CaP particles are the problems related to cohesiveness (with potential material leakage), low mechanical properties, sterilization, injectability, and sedimentation.63
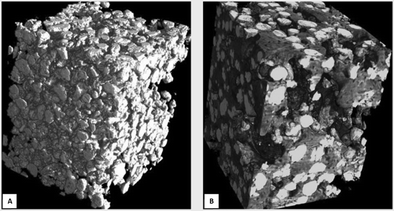 |
| Fig. 2 (A) Reconstructed microtomographic images of IBS Class I BCP (size 40–80 μm) in the hydroxypropyl methylcellulose suspension before implantation and (B) after 8 weeks of implantation in a rabbit femoral defect. Newly formed bone (dark grey) developed extensively in the intergranular macropores between the BCP particles (white).64 | |
2.1.2 Ceramic particles with a hardening matrix.
The Class I subclass comprises ceramic granules of calcium phosphate (thermally sintered), which support osteoconduction, associated with the hardening of an organic or mineral matrix. To overcome the limited initial cohesivity (leakage of the solution) and mechanical properties of non-hardening injectable bone substitute (IBS) composites, CaP sintered particles can be embedded in an organic material that hardens after injection (hydrogels). This hardening matrix can also be a mineral with quick resorption properties (e.g., calcium phosphate65 or calcium sulphate66).
2.1.2.1 Ceramic particles in an organic hardening matrix.
The concept of using self-hardening hydrogels for the formulation of hardening IBS arose in the late 2000s, more particularly with the addition of pH- or temperature-sensitive reactive groups to polymeric macromolecules or modification of their chemistry through physicochemical processes.67 Highly pure preparations of these organic macromolecules can be obtained by synthetic means (e.g., polyethylene glycol) or biosourced (e.g., chitosan, HPMC), with the chain length and modification (e.g., nature, substitution rate) allowing tailored-self-setting hydrogels to be designed according to the intended applications.68 For instance, a “self-hardening injectable calcium phosphate ceramics suspension” (SH-ICPCS) based on BCP-sintered granules associated with silated HPMC (which reticulate at physiological pH) has been developed.39 Similarly, Hofmann et al., in 2007,69 formulated an SH-ICPCS with hydroxyapatite powders mixed with deacetylated chitosan and oxidized starch that forms a putty upon the addition of water. This has a significant advantage that the paste viscosity can be adjusted on the fly by the surgeon as required by the surgical procedure.
2.1.2.2 Ceramic particles in a resorbable mineral matrix.
Calcium phosphate cements (CPCs) are well-known and widely used setting IBS, as described by the informative reviews of Schröter et al.24 The hardening process often occurs within 20 min after mixing the reagents, leading either to a calcium-deficient apatitic phase or to a brushitic phase.70 As such, CPCs can be used as a carrier for the injection of sintered CaP particles and serve as a hardening matrix after implantation (Fig. 3B). In addition to providing a more mechanically stable environment and preventing IBS washout from the filled bone defect, the high bioreactivity of CPCs allows them to serve as a primary anchor for bone formation. Indeed, the CPC matrix is intended to be replaced by bone after a few weeks, leaving sintered CaP granules as scaffolds for the long-term regeneration of the defect. Although minor differences have been observed, this strategy has proven to be clinically relevant with brushite-forming CPCs but failed with apatite-forming CPCs, mainly due to a much lower capacity to be biodegraded.24
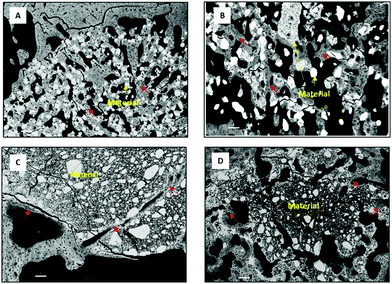 |
| Fig. 3 SEM representative images of IBS implanted in a critical model of rabbit femur after (A) 3 weeks and (B–D) 6 weeks of implantation. Scale bars represent 100 μm (red arrows: newly formed bone). Class I IBS: (A) non-hardening suspension of BCP ceramics in the hydroxypropyl methylcellulose (HPMC) solution (bone formation in contact with BCP particles); (B) hardening suspension of BCP ceramics in a mineral resorbable matrix (MPCP) (bone formation in contact with BCP and the remaining cement).71 Class II IBS: (C) calcium-deficient hydroxyapatite (CDHA) cement (new bone formation occurred in close contact with the surface of the CDHA cement) and (D) macroporous foam of the CDHA cement containing hyaluronic acid hydrogel (the macroporosity and biocompatibility of the material allow its biodegradation and new bone formation). | |
2.2 Class II IBS: calcium phosphate cement associated with polymers
As outlined previously, one of the main issues with Class I IBS relates to the use of calcium phosphate particles, which tend to remain even after several months or even years of implantation, thereby hindering the proper repair of the defect. On the other hand, calcium phosphate cements (CPCs) and especially brushitic cements have shown interesting biological responses, as they appear to biodegrade at a sufficient rate but suffer among other things from a thick consistency before injection (causing injectability and filling issues but also limiting the addition of porogens),72 poor mechanical properties once set (high risk of implant failure),73 and the absence of a macroporous interconnected network (limiting the biological response by acting as a barrier).74
To address this, Class II IBS have been developed, coupling the advantage of CPCs with viscous polymer solutions or self-setting hydrogels (Fig. 4). Viscous-polymer-solution-based Class II IBS appeared in the mid-1990s, such as in the work of Cherng et al.,75 who investigated the injectability and handling of the hydroxyapatite-forming calcium phosphate cement mixed with various “gelling agents” (HPMC, carboxymethyl cellulose CMCs, chitosan acetate, and chitosan lactate). Since then, a large number of substances such as glycerin, silicone gel, polyethylene glycol, liquid paraffin, glycerol, and cellulosic compounds76 have been used to improve the rheological properties and handling of composite CPCs. Concurrently, as the viscosity of such formulations decreases, macroporous injectable CPCs have been developed by the incorporation of porogens in their formulations.72,76–79 However, the generation of a macroporous network in already mechanically weak cements remains a major issue as the possible mechanical benefits induced by the presence of an organic compound are not able to counterbalance the decrease in mechanical properties due to the presence of macropores. To overcome the brittleness of these macroporous Class II IBS, new formulations based on self-setting hydrogels to replace the viscous polymeric solution have recently been developed (for example, with silanized hyaluronic acid, see Fig. 3D). To the author's knowledge, no Class II IBS using reticulating hydrogels are on the market to date, and only a few using a viscous macromolecule solution are commercially available (e.g., Norian® Drillable,37 Graftys® quickset/HBS80).
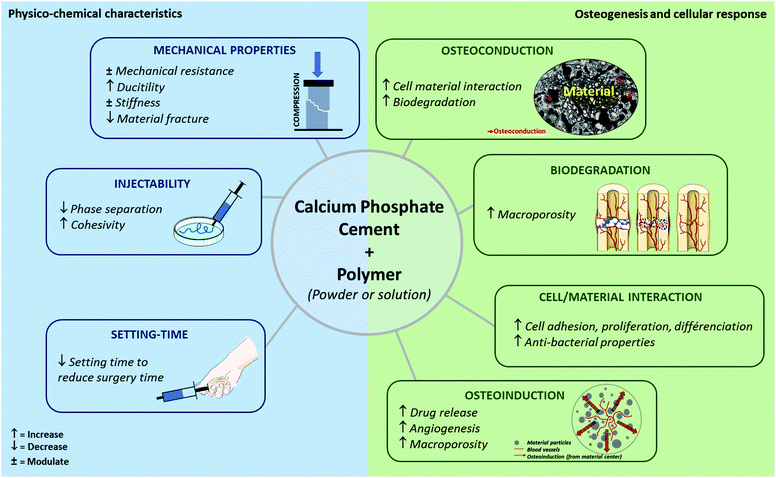 |
| Fig. 4 Modulation of IBS properties by a combination of polymers with calcium phosphate cement (Class II). | |
3. Optimization of calcium phosphate cement Class II by polymer addition
In the following sections, the main research trends are presented, with a specific focus on the benefits resulting from the combination of calcium phosphate cement (CPC) and polymer macromolecules (Fig. 4 and Table 3). Although improvement of IBS properties (e.g., cohesivity and handling) and induction of an in vivo response are essential, it should be noted that sterilization, stability, and storage concerns for these new hybrid formulations are key to their commercialization.
Table 3 Impact of macromolecules or polymeric loading on calcium phosphate bone cement: a few examples
Polymeric phase |
Calcium phosphate cement phase |
Physico-chemical improvement |
Biological improvement |
Ref. |
Collagen microsphere |
Calcium deficient hydroxyapatite; α-tricalcium phosphate-based cement |
|
↑ Macroporosity |
81
|
|
|
↑ Cell accessibility |
|
|
↑ Surface area available for osteoconduction in vivo |
Gelatin |
α-Tricalcium phosphate-based cement |
↓ Setting time |
|
82
|
|
↑ Compressive strength |
|
Gelatin foam |
α-Tricalcium phosphate-based cement |
|
↑Macroporosity |
83
|
|
|
↑ Material degradation in vivo |
Gelatin microsphere |
Rebone biomaterials, Shanghai, China |
↑ Drug release with microsphere charged with rhBMP2 |
↑ Bone mineralization rate |
84
|
Hyaluronic acid |
Tetra-calcium phosphate (TTCP) and dicalcium phosphate anhydrous (DCPA) in an equimolar ratio |
|
↑ Bone formation after implantation in a rat tibial defect |
85
|
Hyaluronic acid microsphere |
α-Tricalcium phosphate-based cement |
↑ Drug release with microsphere charged with platelet lysate |
↓ Epithelial growth |
86
|
|
|
↑ Osteoconduction |
Hydroxypropyl methylcellulose foam |
α-Tricalcium phosphate-based cement |
↑ Injectability |
↑ Macroporosity |
87
|
|
↑ Cohesion |
|
|
↓ Brittleness |
|
Alginate–chitosan complex (microencapsulated MC3T3-E1 cells) |
Brushitic-based cement (β-tricalcium phosphate and monocalcium phosphate monohydrate) |
↓ Compressive strength |
↑ Scaffold remaining |
88
|
|
|
↓ Lamellar-bone-like mineral structure |
|
|
↑ Newly formed collagen |
|
|
↑ Mineralisation rate |
Alginate microbeads |
Chitosan–Calcium phosphate composite |
↑ Drug release with penicillin |
↑ Increase the antibacterial properties |
89
|
|
|
↓ Bacteria activity |
Alginate |
Brushitic cement |
↓ Setting time |
|
90
|
Alginate |
Tetra-calcium phosphate (TTCP) and dicalcium phosphate anhydrous (DCPA) in an equimolar ratio |
↑ Injectability |
|
91
|
|
↑ Cohesion |
|
|
↑ Compressive strength |
|
|
↑ Young's modulus |
|
Chitosan fibres |
α-Tricalcium phosphate-based cement |
↑ Mechanical reinforcement |
|
92
|
Chitosan |
α-Tricalcium phosphate-based cement |
↑Young's modulus |
|
93
|
Chitosan |
Tetracalcium phosphate/a-tri-calcium phosphate |
|
↑ Osteoconduction of the material in mandibular rat model |
94
|
Chitosan with arginine–glycine–aspartate motif |
Tetra-calcium phosphate (TTCP) and dicalcium phosphate anhydrous (DCPA) in a equimolar ratio |
↑ Flexural strength |
↑ New bone volume in Bone defect at femoral condyles of New Zealand white rabbits |
95
|
Strontium-poly(γ-glutamic acid) |
α-Tricalcium phosphate-based cement |
↑ Mechanical resistance |
|
96
|
Poly(lactic-co-glycolic acid) |
α-Tricalcium phosphate-based cement |
|
↑ Macroporosity |
74
|
|
|
↑ Degradation rate |
|
|
↑ Bone formation |
Lactide modified poly(ethylene glycol) dimethacrylate (PEG-PLLA-DMA) |
Brushitic cement |
↓ Compressive strength |
|
97
|
|
↑ Mechanical deformation |
|
Poly(lactic-co-glycolic acid)-b-poly(ethylene glycol)-b-poly(lactic-co-glycolic acid) (PELGA) |
Hydroxyapatite |
↑ Drug release with rhBMP |
↑ Accelerate bone formation |
98
|
|
|
Maintain mechanical integrity of the rate femoral defect |
3.1 Improvement of calcium phosphate cement physico-chemical properties by polymer addition
3.1.1 Injectability.
The capacity of calcium phosphate cement (CPC) systems to remain homogeneous during injection99 may be limited by their injectability. Phase separation during injection can be caused by the size, shape, and distribution of powder grains,100 paste homogeneity, and mechanical extrusion101 (i.e., pressure exerted by the syringe plunger, which causes the liquid phase to drain and the solids to consolidate; suction of the powder network during its flow; or filtration in the needle with the formation of a “carpet” of solids102). These limitations restrict their potential application and especially their use in minimally invasive surgery. These disadvantages that influence phase segregation can be explained by the low viscosity of the liquid phase (generally with a viscosity close to that of water), which flows more readily than the solid phase. Different methods to reduce the phase separation of CPC materials during extrusion have been devised, including increasing the powder/liquid ratio and injection rate, reducing the plastic limit, increasing the viscosity of the liquid component, and optimising the cylinder geometry. Adding a viscous liquid has been shown to reduce phase separation during the injection/extrusion of CaP pastes and cements,99 but an excessive increase in liquid viscosity increases the extrusion force, which may exceed the force that the surgeon (limit of manual injection: 100–300 N78,103) has to apply while still maintaining sufficient control. Modulation of the CPC/polymer association (Fig. 5A) can significantly reduce phase separation.104 Examples of such viscous binders include cellulosic derivatives (cellulose and hydroxypropyl methylcellulose),105 collagen/gelatin, hyaluronic acid,106 chitosan,107,108 and alginate,109 which create strong attractive forces between the polymer and CaP particles. Similarly, the addition of beads (e.g. poly(lactic-co-glycolic) acid (PLGA)) may help to modulate the paste injectability depending on their size and concentration.99,100 More recently, Vojtova et al.110 associated a hydrogel forming copolymer composed of poly(lactic acid)–poly(glycolic acid)–poly(ethylene glycol) with α-TCP-based cement, thereby increasing the viscosity of the composite due to the reticulation of the macromolecule chains. They demonstrated that the pastes that retained a high level of shear stress did not exhibit phase separation during either the rheological or the injection tests.
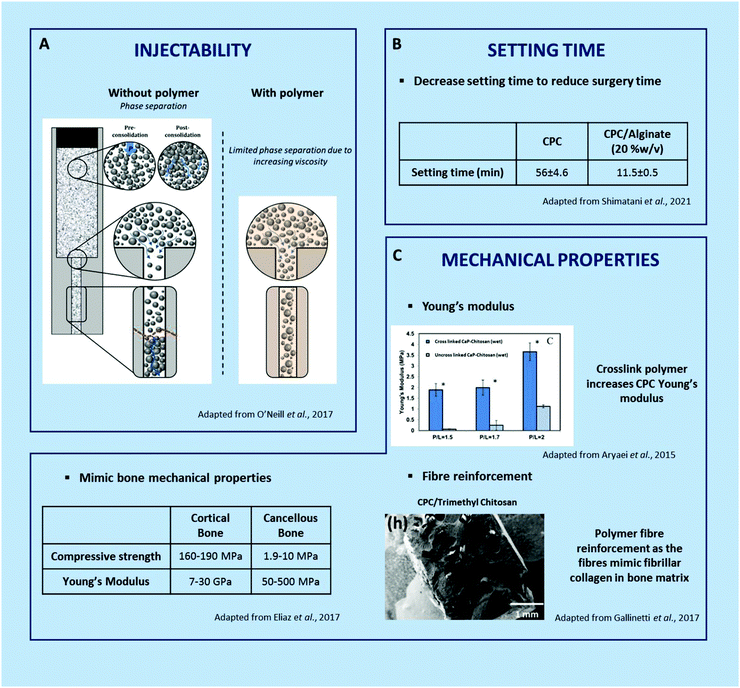 |
| Fig. 5 Improvement of calcium phosphate cement physico-chemical properties by polymer addition.90,92,93,102,111 | |
Caballero et al.9, associated poly(L-lysine) dendrigrafts polyethylene glycol homobifunctionalized with N-hydroxysuccinimide hydrogel with α-TCP-based cement, which had an excellent injectability, with total paste extruded at low force (7 ± 1 N), correlated with the cohesivity improvement.
In short, the addition of macromolecules to hydraulic cements represents a fantastic tool to tailor their injectability. They however affect the physico-chemical features of the IBS such as the setting time. On a purely technical note, this is why (i) injectability studies should not be done independently of setting time studies and (ii) the injectability of a cement should be measured after a time interval related to the setting time.99
3.1.2 Setting time.
In brief, the setting of a hydraulic cement paste results in the dissolution of a reactive inorganic powder and the re-precipitation of a new inorganic phase, providing the final structure and features of the cement. Today, it is well-known that the setting time of such cements, including CPC, can be modulated by (i) the particle size and the crystallinity of the reactive powder, (ii) retarders (e.g., citric acid) or accelerators (e.g., NaH2PO4) as admixtures in the liquid or solid phase, (iii) the setting temperature and surrounding humidity and (iv) the reactive powder to liquid ratio (P/L ratio).100,112
For a given P/L ratio and defined reactive species (both powder and liquid phases), the addition of macromolecules (or polymers) may significantly affect in various ways the cement-forming reaction, from steric hindrance, physico-chemical adsorption onto the reactive powder to bonding with the released ions. Although much remains to be investigated and understood about the effect of macromolecules on CPC setting time, it is evident that their nature, features, and functionalization are key parameters.
For instance, Shimatani et al.90 demonstrated that the setting time of a brushitic cement decreased with the increasing addition of low viscosity sodium alginate (from 56.0 to 11.5 min with 0 and 20% w/v, respectively, Fig. 5B).90 Similarly, the addition of silanized HPMC seemed to reduce the setting time of an apatitic cement (e.g., P/L =1.25), from 26 to 18 min with 0 and 4% w/v Si-HPMC,105 whereas the addition of gelatin tends to increase it (e.g.), P/L = 2.5, from 10 to 25 min with 0 and 10% w/v gelatin.113 It should be noted that it is of prime importance not to modify the reactive powder to liquid ratio to conclude on the influence of a given additive, which may not be that obvious.
Optimizing both setting time and injectability of the formulation can be achieved to match the intended clinical application. In the end, it is essential to prepare an easily injectable IBS with an appropriate setting time so that it sets slowly enough to give the surgeon time to inject it but quickly enough to prevent any material leakage out of the defect and to limit operating time.112
3.1.3 Mechanical properties.
As shown in the clinical study performed by Blattert et al.,114 CPCs still exhibit poor resistance to mechanical loading. Ideally, the CPC mechanical properties should be close to those of natural bone,24 cortical bone (E = 7–30 GPa per compressive strength = 160–190 MPa) or the cancellous bone (E = 50–500 MPa per compressive strength = 1.9–10 MPa).111,115 However, CPCs’ specifications should be adapted to their intended clinical applications, and their final mechanical properties should be modulated as the function of the nature of the bone to treat and its localization (loading versus non-loading site).111,112,114,116
Current research revolves around 3 main focal points, which are (i) the improvement of their resistance to compression, traction and shear stress, (ii) the development of stiff but non-brittle formulations after setting, and (iii) the enhancement of their fracture toughness through the use of macromolecules or polymers as additives.
The addition of water soluble macromolecules is known to affect the setting reaction through a wide range of interactions as explained in Section 3.1.2. As a direct consequence, the cement's nano- to micro-structure, which greatly affects the properties of a set cement, is modulated, as illustrated later on. In addition to the nano- to micro-structural changes of the inorganic phase, the mechanical enhancement could also be achieved through the binding of macromolecular chains to the CaP crystal surface (calcium ions providing a preferential target of many anionic chains).20 However, it is important to note that an excessive concentration of macromolecules could strongly decrease the setting time or even prevent it; hence a suitable balance is required to be found. On the other hand, reinforcing cement with polymeric fibers is a common strategy in other fields;21 the nature of the polymer fiber along with other factors such as the length of the fiber, the volume fraction, the orientation and the fiber/matrix adhesion have a relevant effect.22 Of course, both strategies could be combined to optimize cement mechanical properties.
Gallinetti et al.92 and Gao et al.96 have shown the benefits of adding macromolecules in their CPC formulation to reinforce the material, indeed, with the aim to reinforce the material mechanically. For example, with the addition of trimethyl chitosan fibre reinforced in the CPC,92 when the matrix starts to fissure, fibres bridge the crack to prevent it from opening and propagating any further. Moreover, crack deflection by the fibres extends the distance over which the crack propagates, consuming more energy in newly formed surfaces.117 This can be compared to the cortical bone, where the fibrillar collagen architecture allows the bone to be reinforced. Or, with the addition of strontium-poly(γ-glutamic acid) in the CPC,96 during the setting time, a compact microstructure was created by the surrounding calcium particles and γ-PGA that enhanced the mechanical resistance of the material. Another way to mimic the bone mechanical properties with their fracture resistance is to associate anionic and cationic polymers with a cement to obtain heterogeneous agglomeration in the CPC.88
With the aim to increase young's modulus, Aryaei et al.93 combined a cross-linked tripolyphosphate chitosan with α-TCP-based cement. In the wet solution, Young's modulus increased 2 to 4 times according to the powder/liquid mass ratio. The material reinforcement was greater as the polymeric chain was lengthened. The authors hence suggested that increasing the concentration and the cross-linking time of the polymer increases the modulus value (Fig. 5C).
Finally, another important aspect for the biomaterial implantation in a bone defect is the consideration of the effect of the mechanical stress exerted by the host tissue on the ability of the material to be deformed under the stress. The ductility of the cement is characterized by a higher deformation before rupture. Here, the polymer will allow the cement to support a load better than the CPC alone. With the addition of polymers, the curves of compression are diminished, and the deformation is greater than that for the cement alone, at 30% with PEG-PLLA-DMA/brushite compared to 5% with brushite alone.97 It has been suggested that the crystals can grow more readily and become entangled in the hydrogel network and hence provide high mechanical performance.
3.2 Improvement of induced osteogenesis and cellular response
3.2.1 Anti-bacterial properties.
Bone infection after implantation of biomaterials in an injured site is among the greatest challenges faced in the field.118,119 As an alternative to conventional drug therapies, researchers have tried loading drugs directly into the CPC.120–123 However, their release profile is often suboptimal and may not allow efficient treatment of the infection.124 This is because (i) it may be difficult to reach a sufficient drug load over a relevant time period and (ii) the CPC/drug interactions may inactivate the drugs due to pH, ion binding, or other factors. Therefore, polymers, used as vectors for protection and controlled delivery of active substances, are clinically relevant to topically fight infection. For instance, Wu et al.125 associated penicillin-containing alginate microbeads with a chitosan/CPC composite (Fig. 6A) to increase the antibacterial properties of the chitosan by sustained drug release to inhibit the activity of bacteria (Staphylococcus aureus). It was also shown that the addition of silver ions in the brushitic or apatitic cementitious phase allowed to present antibacterial properties with rapid release according to the nature and the solubility of the CPC.126
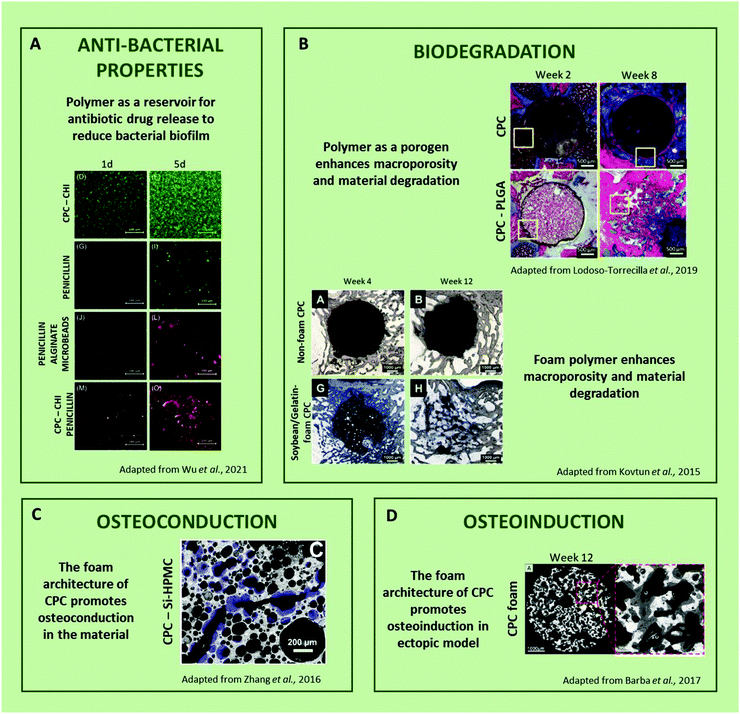 |
| Fig. 6 Improvement of calcium phosphate cement induction of osteogenesis and cellular response (CPC = calcium phosphate cement, CHI = chitosan, Si-HPMC = silanized hydroxypropylméthylcellulose, PLGA = poly(lactic-co-glycolic acid),83,103,127,128). | |
Another attractive alternative to standard drugs may rely on the inherent antibacterial properties of certain polymers. Antimicrobial polymers have been described in terms of their capacity to inhibit or kill bacteria due to their chemical structure (i.e., quaternary nitrogen groups, halamines, and polylysine).129 Fortunately, positively charged amphiphilic polymers do display antibacterial activity due to their ability to penetrate the membrane and kill bacteria.130 For example, N-(2-hydroxypropyl)-3-trimethyl chitosan chloride (HTCC) and the bioadhesive poly(dextran-aldehyde) hydrogels exhibited activity against Gram-positive and Gram-negative bacteria and also promoted the wound healing in a rat model.131 The formulation of IBS coupling CPCs and these hydrogels would be of clinical interest to greatly limit the potential infection after implantation. For instance, several studies have already reported the association of CPC with chitosan, hence little effort may be required to formulate an innovative drug-free antibacterial solution.127,129–134
3.2.2 Host-material interactions and osteoconductivity.
The implantation of a foreign body automatically triggers a reaction of the host biological system.135 Biomaterials, even those deemed 100% biocompatible such as CPCs, are no exception; hence controlling host-material interactions to positively stimulate the formation of a new tissue is the key.136 The biomaterial surface, serving as an interface, presents a wide range of physico-chemical cues (e.g., chemistry, topography, wettability, porosity, electrical charges) which will impact cell recruitment, adhesion, proliferation, and differentiation.137 As inherent features resulting from their formation process, CPCs display a very high contact surface with their in situ environment due to the presence of a micro- and nano-porous network even for bulk samples (at the macro-scale).20 Consequently, they are considered as more “bioactive” than their ceramic counterparts with the same composition by maximizing the exchanges (e.g., the release of ions138,139) with their biological surroundings. This enhanced bioactivity (versus ceramics of comparable composition) could also be attributed to CPC surface topology, often composed of petal or needle-like microstructures which is known to enhance cell adhesion and fate.140 Taking advantage of physico-chemical and biological features of macromolecules could be a relevant strategy to further the biomaterial-induced biological response of CPCs, modulating their biodegradation, osteoconduction and osteoinduction.85,94,133 These specific points will be detailed in the following sections.
Osteoconductivity was determined by the physicochemical characteristics of the material to support tissue ingrowth, osteoprogenitor cell growth, and development for bone formation to occur.141 Due to their chemical composition and micro-nano-structure, CPCs exhibited high osteoconduction which allows for their perfect osteointegration within a bone defect.142 By modulating these features and also bringing their own biological benefits, macromolecules could be combined with CPCs to improve their osteoconductivity. Indeed, Kjalarsdóttir et al.94 injected a chitosan/CPC in a mandibular rat model. After 14 days of implantation, new bone growth formed as an outgrowth from the periosteum, covering the surface of the bone, located along specific areas of the mandible. The authors suggested that the biomechanical weakening of the polymer/cement implant structure stimulates the osteoconduction of the material. Similarly, Cui et al.85 showed that an increase in the hyaluronic acid concentration from 0% to 4% in calcium phosphate cement resulted in more bone formation after 12 weeks of implantation in a rat tibial defect. In this case, they suggested that the incorporation of hyaluronic acid may promote higher osteogenicity by the secretion of stimulatory factors and osteogenic gene expression.
However, as most CPCs are often lacking an interconnected macroporous network, osteoconduction is often still limited to the bone defect/material interface.143 This could also be solved by the addition of macromolecules or polymers to CPCs, serving as a porogen84,86,103,133 or a foaming agent.83,87 This directly affects their ability to be invaded by a newly formed bone tissue up to their core, as well as their ability to be biodegraded.
3.2.3 Biodegradation.
A relevant biodegradation rate of the CPC is clearly an important feature as it both provides free space for the formation of new bone and allows for the release of bioactive ions such calcium (Ca2+) and phosphate (PO43−) which are essential to stimulate bone deposition.138,139 This degradation is not only produced by (i) a passive dissolution of the material, especially in the case of apatite, but it is mainly resorbed by (ii) the biological activity of immune cells and osteoclasts.144,145 The acidic environment produced by these cells allows the local dissolution of the latter.144 However, without a proper macro-architecture, biodegradation of the CPC remains peripheral. That is why, the presence of an interconnected macroporous network had been deemed essential for both material biodegradation146 and bone formation. It has been widely demonstrated that macroporosity greater than 100 μm and interconnections20 are required to allow bone growth.23 Pore interconnection is essential to increase adhesion, cell colonization, and vascularization, as opposed to closed porosity, which only reduces the mechanical properties.147 Furthermore, it has been assumed that the accumulation of phosphate and calcium ions mostly occurs in the core of the materials, in materials with high surface areas, and in concave pores (as opposed to convex pores)148 as this makes these pores a very favourable environment for the cells.
One of the possible ways to create macroporosity in CPCs from polymers is to incorporate sacrificial porogens (particles149 or microspheres86). The porosity is classified by size with micropores (<1 μm), mesopores (1–100 μm) and macropores (>100 μm).146,150 Babo et al.86 associated hyaluronic acid microspheres loaded with platelet lysate with a calcium phosphate cement as an osteointegrative biodegradable system. In this study, after 6 weeks of implantation in bilateral intrabony defects in maxillary first molars of rats, the material resulted in a beneficial decrease in the epithelial growth and overall periodontal healing. Despite these beneficial properties, the mineral phase of the material was degraded too fast compared to the hyaluronic particles and a lack of mechanical properties was observed. This is an interesting example that highlights the importance of controlled and simultaneous biodegradation and osteoconduction to allow consolidated bone regrowth. Lodoso-Torrecilla et al.74 developed CPC/PLGA combined with sucrose particles. The 60 μm microspheres of PLGA and 400 μm microspheres of sucrose improved the macroporosity, increasing the degradation rate and bone formation (20% newly formed bone with the composite compared to 0.1% with the CPC at 8 weeks). PLGA hydrolysis that was supposed to represent the late stage (6–8 weeks) induced as early as 2 weeks after implantation of the second intended degradation of the CPC matrix via local acidification, which may have been beneficial for bone formation.
Another way to generate macroporosity in CPCs relies on the surfactant properties of certain macromolecules. For instance, Zhang et al.87 developed an injectable cement foam, based on a mixture of Si-HMPC hydrogel and α-TCP. After setting, a hydroxyapatite-deficient calcium (CDHA) structure displaying interconnected macropores from 10 to 200 μm in size was observed. As simple ways to introduce macroporosity in injectable systems, forming stable foam is increasingly being studied.87,151,152 The heterogeneity of the porosity and the interconnection created using the foam techniques provide an interesting environment for the cells to adhere, proliferate, differentiate, and migrate by nesting in the material interconnected with macropores (Fig. 3D). Kovtun et al.83 associated gelatin-soybean with a CPC foam. The foams were formed with a manual system and the results revealed a high degree of degradation of the material, with 60% more degraded material than the non-polymer cement. Therefore, the microporosity of materials that allows invasiveness of cells in the material remains one of the most important properties in the development of such injectable materials.
3.2.4 Osteoinduction.
Osteoinduction is the ability of a material to form new bone in an ectopic site.153 Bohner et al.154 described the material prerequisites for intrinsic osteoinduction as (i) material mineralization with a bioactive apatite layer consuming released ions; (ii) a porous material; (iii) pores large enough to allow vessel growth and cell transport (minimum interconnection 50 μm); (iv) a blood supply to maintain physiological concentrations of phosphate and calcium. The architecture of materials could be responsible for the osteoinductive properties. In order to compare and study the osteoinduction of the materials in ectopic sites with different architectures, Barba et al.128 performed intramuscular-implantation in a beagle dog model (Fig. 6D). The calcium phosphate cement (calcium phosphate-deficient hydroxyapatite) foam porosity characteristic compared to a 3D porosity architecture showed a clear difference in terms of an increase in bone growth in the ectopic zone. The high reactivity of the biomimetic CDHA, which is due to its poor crystallinity, nanostructured nature, combined with the concave macroporosity produced by the foaming process, resulted in accelerated osteoinduction compared with conventional sintered BCP ceramics with the same macroporous architecture. This strategy is very promising for the development of an efficient injectable cement. The association of the polymer with a CPC as a foam would allow the creation of an injectable material with the right mechanical strength and macroporosity to promote osteoconduction and osteoinduction of the material.86,87,128,155
Some authors augmented this phenomenon by incorporating growth factors such as bone morphogenic protein (BPM) in the biomaterial. For example, Li et al.84 added rhBPM-2 (recombinant human bone morphogenetic protein) encapsulated in gelatin microspheres associated with calcium phosphate cement. The role of the polymer was to control the release of the factor to repair bone defects. Growth factors should be included in bioactive apatite layers on the surface, and their release is caused by inflammatory cell action on the material surface. Similarly, Zhang et al.98 highlighted that the addition of 400 ng of rhBMP (recombinant human bone morphogenetic protein-2/7 heterodimer) in macroporous cement composed of the degradable amphiphilic polymer PELGA (poly(lactic-co-glycolic acid)-b-poly(ethylene glycol)-b-poly(lactic-co-glycolic acid)) and osteoconductive HA (hydroxyapatite) resulted in significantly higher osteoinduction compared to the material without rhBMP. The addition of rhBMP-2/7 to this material accelerated robust bone formation and achieved the full functional restoration of the mechanical integrity of the rat femoral defect.
In another way, without using osteoinductive molecules, polymers such as chitosan/collagen156 or hyaluronan95,157 have shown osteoinductive properties in ectopic sites and in vitro (e.g. osteogenic differentiation).
The nature, dose and molecular weight of the macromolecule incorporated in the CPC appear to be relevant parameters for osteoinduction. Although this point remains largely unexplored, the hypothetic osteogenic potential of macromolecules represents an additional reason for incorporating them in CPC in addition to all the above benefits.
4. Conclusion
The association of polymers with calcium phosphate cement or granules is increasingly being used in the development of injectable bone substitutes for bone tissue engineering. It is important to establish a classification for the nomenclature of biomaterials that are being used and that are currently under development. The Class I IBS ceramics currently on the market, which tend to remain in place even after several months or even years of implantation, have poor initial mechanical properties and tend to leak out of the defect during injection. On the other hand, Class II calcium phosphate cements have been shown to lead to interesting biological responses, and they appear to biodegrade at a suitable rate, while nevertheless suffering from limitations. In this review, several means are proposed to overcome these limitations to make these calcium phosphate cements better materials for achieving better bone growth. Improved parameters are presented here individually, but the real challenge is being able to combine all the required properties for an injectable material. Presently, it should be pointed out that foaming techniques appear to be very promising, with the potential to create injectable, ductile, macroporous, biodegradable materials that result in the pronounced biological responses required for bone repair.
Conflicts of interest
There are no conflicts to declare.
References
-
R. Florencio-Silva, G. R. D. S. Sasso, E. Sasso-Cerri, M. J. Simões and P. S. Cerri, Biology of Bone Tissue: Structure, Function, and Factors That Influence Bone Cells, BioMed Research International, Hindawi Publishing Corporation, 2015, vol. 2015 Search PubMed
.
- R. Marsell and T. A. Einhorn, The biology of fracture healing, Injury, 2011, 42(6), 551–555 CrossRef PubMed
.
- A. Oryan, S. Monazzah and A. Bigham-Sadegh, Bone injury and fracture healing biology, Biomed. Environ. Sci., 2015, 28, 57–71 CAS
.
- E. Gibon, L. Y. Lu, K. Nathan and S. B. Goodman, Inflammation, ageing, and bone regeneration, J. Orthop. Translat., 2017, 10, 28–35 CrossRef PubMed
.
- A. L. Boskey and R. Coleman, Critical reviews in oral biology & medicine: Aging and bone, J. Dent. Res., 2010, 89, 1333–1348 CrossRef CAS PubMed
.
- A. Karpouzos, E. Diamantis, P. Farmaki, S. Savvanis and T. Troupis, Nutritional Aspects of Bone Health and Fracture Healing, J. Osteoporosis, 2017, 2017, 4218472 Search PubMed
.
- K. L. Troy, M. E. Mancuso, T. A. Butler and J. E. Johnson, Exercise early and often: Effects of physical activity and exercise on women’s bone health, Int. J. Environ. Res. Public Health, 2018, 15, 878 CrossRef PubMed
.
- A. M. Al-Bashaireh, L. G. Haddad, M. Weaver, X. Chengguo, D. L. Kelly and S. Yoon, The Effect of Tobacco Smoking on Bone Mass: An Overview of Pathophysiologic Mechanisms, J. Osteoporosis, 2018, 2018 Search PubMed
.
- V. Sundararaghavan, M. M. Mazur, B. Evans, J. Liu and N. A. Ebraheim, Diabetes and bone health: latest evidence and clinical implications, Ther. Adv. Musculoskeletal Dis., 2017, 9, 67–74 CrossRef CAS PubMed
.
- T. D. Rachner, S. Khosla and L. C. Hofbauer, Osteoporosis: Now and the future, Lancet, 2011, 377, 1276–1287 CrossRef CAS
.
- C. Savvidis, S. Tournis and A. D. Dede, Obesity and bone metabolism, Hormones, 2018, 17, 205–217 CrossRef PubMed
.
- L. J. Poort, J. H. B. Ludlage, N. Lie, R. A. Böckmann, J. C. E. Odekerken and F. J. Hoebers,
et al., The histological and histomorphometric changes in the mandible after radiotherapy: An animal model, J. Cranio-Maxillofacial Surg., 2017, 45(5), 716–721 CrossRef PubMed
.
- R. S. Hardy, H. Zhou, M. J. Seibel and M. S. Cooper, Glucocorticoids and bone: Consequences of endogenous and exogenous excess and replacement therapy, Endocr. Rev., 2018, 39, 519–548 CrossRef PubMed
.
-
P. Vestergaard, Drugs Causing Bone Loss, Handbook of Experimental Pharmacology, Springer Science and Business Media Deutschland GmbH, 2020, pp. 475–497 Search PubMed
.
- L. A. Mills, S. A. Aitken and A. H. R. W. Simpson, The risk of non-union per fracture: current myths and revised figures from a population of over 4 million adults, Acta Orthop., 2017, 88(4), 434–439 CrossRef PubMed
.
- N. G. Lasanianos, N. K. Kanakaris and P. V. Giannoudis, Current management of long bone large segmental defects, Orthop. Traumatol., 2010, 24(2), 149–163 Search PubMed
.
- T. A. DeCoster, R. J. Gehlert, E. A. Mikola and M. A. Pirela-Cruz, Management of posttraumatic segmental bone defects, J. Am. Acad. Orthop. Surg., 2004, 12, 28–38 CrossRef PubMed
.
- A. Van Heest and M. Swiontkowski, Bone-graft substitutes, Lancet, 1999, 353(suppl. 1), 28–29 CrossRef
.
- T. Boyce, J. Edwards and N. Scarborough, Allograft bone: the influence of processing on safety and performance, Orthop. Clin. North Am., 1999, 30(4), 571–581 CrossRef CAS PubMed
.
- I. Lodoso-Torrecilla, J. J. J. P. van den Beucken and J. A. Jansen, Calcium phosphate cements: Optimization toward biodegradability, Acta Biomater., 2021, 119, 1–12 CrossRef CAS PubMed
.
- S. Bevan, Economic impact of musculoskeletal disorders (MSDs) on work in Europe, Best Pract. Res., Clin. Rheumatol., 2015, 29, 356–373 CrossRef PubMed
.
- P. V. Giannoudis and I. Pountos, Tissue regeneration: The past, the present and the future, Injury, 2005, 36(suppl. 4), S2–S5 CrossRef PubMed
.
- G. Hannink and J. J. C. Arts, Bioresorbability, porosity and mechanical strength of bone substitutes: What is optimal for bone regeneration?, Injury, 2011, 42, S22–S25 CrossRef PubMed
.
- L. Schröter, F. Kaiser, S. Stein, U. Gbureck and A. Ignatius, Biological and mechanical performance and degradation characteristics of calcium phosphate cements in large animals and humans, Acta Biomater., 2020, 117, 1–20 CrossRef PubMed
.
- M. Bongio, J. J. J. P. van den Beucken, S. C. G. Leeuwenburgh and J. A. Jansen, Preclinical evaluation of injectable bone substitute materials, J. Tissue Eng. Regener. Med., 2012, 9(3), 191–209 CrossRef PubMed
.
- P. Weiss, P. Layrolle, L. P. Clergeau, B. Enckel, P. Pilet and Y. Amouriq,
et al., The safety and efficacy of an injectable bone substitute in dental sockets demonstrated in a human clinical trial, Biomaterials, 2007, 3295–3305 CrossRef CAS PubMed
.
- P. Guida, R. Ragozzino, B. Sorrentino, A. Casaburi, R. D. D’Amato and G. Federico,
et al., three-in-One minimally invasive approach to surgical treatment of pediatric pathological fractures with wide bone loss through bone cysts: ESIN, curettage and packing with injectable HA bone substitute. A retrospective series of 116 cases, Injury, 2016, 47(6), 1222–1228 CrossRef PubMed
.
- D. A. Colon, B. J. V. Yoon, T. A. Russell, F. P. Cammisa and C. Abjornson, Assessment of the injection behavior of commercially available bone BSMs for Subchondroplasty® procedures, Knee., 2015, 22(6), 597–603 CrossRef PubMed
.
- D. Papadia, F. Calascibetta and L. Bertoldi, Displaced intra-articular calcaneal fractures treated with open reduction and internal fixation and bone void filling with an injectable calcium sulfate/hydroxyapatite bone graft substitute: a series of 18 patients, Arch. Trauma. Res., 2018, 7(1), 2 CrossRef
.
- T. Miramond, P. Corre, P. Borget, F. Moreau, J. Guicheux and G. Daculsi,
et al., Osteoinduction of biphasic calcium phosphate scaffolds in a nude mouse model, J. Biomater. Appl., 2014, 29(4), 595–604 CrossRef CAS PubMed
.
- S. Myoung Lee, Clinical evaluation of efficacy and safety of NOVOSIS-inject containing bmp-2 for socket preservation after extraction of a single-rooted tooth, Clin. Oral Implants Res., 2018, 29, 318 CrossRef
.
- J. D. Smucker, E. B. Petersen, J. V. Nepola and D. C. Fredericks, Assessment of Mastergraft(®) strip with bone marrow aspirate as a graft extender in a rabbit posterolateral fusion model, Iowa. Orthop. J., 2012, 32, 61–68 Search PubMed
.
- F. Westhauser, C. Essers, M. Karadjian, B. Reible, G. Schmidmaier and S. Hagmann,
et al., Supplementation with 45S5 bioactive glass reduces in vivo resorption of the β-tricalcium-phosphate-based bone substitute material vitoss, Int. J. Mol. Sci., 2019, 20(17), 4253 CrossRef CAS PubMed
.
- A. Hanke, M. Bäumlein, S. Lang, B. Gueorguiev, M. Nerlich and T. Perren,
et al., Long-term radiographic appearance of calcium-phosphate synthetic bone grafts after surgical treatment of tibial plateau fractures, Injury, 2017, 48(12), 2807–2813 CrossRef PubMed
.
- C. W. Oh, K. C. Park and Y. H. Jo, Evaluating augmentation with calcium phosphate cement (chronOS Inject) for bone defects after internal fixation of proximal tibial fractures: a prospective, multicenter, observational study, Orthop. Traumatol. Surg. Res., 2017, 103(1), 105–109 CrossRef CAS PubMed
.
- T. Nusselt, A. Hofmann, D. Wachtlin, S. Gorbulev and P. M. Rommens, CERAMENT treatment of fracture defects (CERTiFy): protocol for a prospective, multicenter, randomized study investigating the use of CERAMENTTM BONE VOID FILLER in tibial plateau fractures, Trials., 2014, 15(1), 75 CrossRef PubMed
.
- J. Schauwecker, M. Bock, F. Pohlig, H. Mühlhofer, J. Tübel and R. Von Eisenhart-Rothe,
et al., In vitro Growth Pattern of Primary Human Osteoblasts on Calcium Phosphate- and Polymethylmethacrylate-Based Bone Cement, Eur. Surg. Res., 2017, 58(5–6), 216–226 CrossRef CAS PubMed
.
- C. Mellier, F. Lefèvre, F. Fayon, V. Montouillout, C. Despas and M. Le,
et al., Acta Biomaterialia A straightforward approach to enhance the textural, mechanical and biological properties of injectable calcium phosphate apatitic cements (CPCs): CPC/blood composites, a comprehensive study, Acta Biomater., 2017, 62, 328–339 CrossRef CAS PubMed
.
-
P. Weiss, J. Delecrin, G. Grimandi, N. Passuti and G. Daculsi, Biomaterial Composition and Method for Preparing the Same, europe; EP 0 692 986 B1, 1994 Search PubMed
.
- P. Weiss, O. Gauthier, J. M. Bouler, G. Grimandi and G. Daculsi, Injectable bone substitute using a hydrophilic polymer, Bone, 1999, 67S–70S CrossRef CAS
.
- A. Fatimi, J.-F. Tassin, M. A. V. Axelos and P. Weiss, The stability mechanisms of an injectable calcium phosphate ceramic suspension, J. Mater. Sci.: Mater. Med., 2010, 21(6), 1799–1809 CrossRef CAS PubMed
.
- A. Fatimi, J.-F. Tassin, J. Bosco, R. Deterre, M. A. V. Axelos and P. Weiss, Injection of calcium phosphate pastes: prediction of injection force and comparison with experiments, J. Mater. Sci. Med., 2012, 23(7), 1593–1603 CrossRef CAS PubMed
.
- P. Weiss, M. L. Kowski, R. Z. Legeross, J. M. Bouler, A. Jean and G. Daculsi, Fourier-transform infrared spectroscopy study of an organic-mineral composite for bone and dental substitute materials, J. Mater. Sci.: Mater. Med., 1997, 8, 621 CrossRef CAS PubMed
.
- G. Grimandi, P. Weiss, F. Millot and G. Daculsi, In vitro evaluation of a new injectable calcium phosphate material, J. Biomed. Mater. Res., 1998, 39(4), 660–666 CrossRef CAS PubMed
.
- F. Millot, G. Grimandi, P. Weiss and G. Daculsi, Preliminary in vivo studies of a new injectable bone substitute, Cells Mater., 1999, 9(1), 2 Search PubMed
.
- O. Gauthier, I. Khairoun, J. Bosco, L. Obadia, X. Bourges and C. Rau,
et al., Noninvasive bone replacement with a new injectable calcium phosphate biomaterial, J. Biomed. Mater. Res., Part A, 2003, 47–54 CrossRef CAS PubMed
.
- G. Daculsi, O. Laboux, O. Malard, P. Weiss, D. Guy and L. Olivier,
et al., Current state of the art of biphasic calcium phosphate bioceramics, J. Mater. Sci.: Mater. Med., 2003, 4(14), 195–200 CrossRef PubMed
.
- B. Enkel, C. Dupas, V. Armengol, J. A. Adou, J. Bosco and G. Daculsi,
et al., Bioactive materials in endodontics, Expert Rev. Med. Dev., 2008, Vol. 5, 475–494 CrossRef PubMed
.
- E. Lerouxel, P. Weiss, B. Giumelli, A. Moreau, P. Pilet and J. Guicheux,
et al., Injectable calcium phosphate scaffold and bone marrow graft for bone reconstruction in irradiated areas: an experimental study in rats, Biomaterials, 2006, 27(26), 4566–4572 CrossRef CAS PubMed
.
- D. Boix, P. Weiss, O. Gauthier, J. Guicheux, J. M. Bouler and P. Pilet,
et al., Injectable bone substitute to preserve alveolar ridge resorption after tooth extraction: A study in dog, J. Mater. Sci.: Mater. Med., 2006, 17(11), 1145–1152 CrossRef CAS PubMed
.
- G. Daculsi, P. Weiss, J.-M. M. Bouler, O. Gauthier, F. Millot and E. Aguado, Biphasic calcium phosphate/hydrosoluble polymer composites: a new concept for bone and dental substitution biomaterials, Bone, 1999, 25(2 SUPPL. 1), 99–101 Search PubMed
.
- D. Boix, O. Gauthier, J. Guicheux, P. Pilet, P. Weiss and G. Grimandi,
et al., Alveolar bone regeneration for immediate implant placement using an injectable bone substitute: an experimental study in dogs, J. Periodontol., 2004, 75(5), 663–671 CrossRef PubMed
.
- O. Gauthier, D. Boix, G. Grimandi, E. Aguado, J.-M. Bouler and P. Weiss,
et al., A new injectable calcium phosphate biomaterial for immediate bone filling of extraction sockets: a preliminary study in dogs, J. Periodontol., 1999, 70(4), 375–383 CrossRef CAS PubMed
.
- D. Boix, O. Gauthier, J. M. Bouler, P. Weiss, G. Grimandi and J. Guicheux,
et al., Immediate implantation of titanium dental implants associated to an injectable bone substitute immediately after tooth extraction, Eur. Cells Mater., 2001, 1(SUPPL. 1), S219–S222 Search PubMed
.
- O. Gauthier, E. Goyenvalle, J.-M. Bouler, J. Guicheux, P. Pilet and P. Weiss,
et al., Macroporous biphasic calcium phosphate ceramics versus injectable bone substitute: a comparative study 3 and 8 weeks after implantation in rabbit bone, J. Mater. Sci.: Mater. Med., 2001, 12(5), 385–390 CrossRef CAS PubMed
.
- O. Gauthier, R. Müller, D. Von Stechow, B. Lamy, P. Weiss and J. M. Bouler,
et al., In vivo bone regeneration with injectable calcium phosphate biomaterial: a three-dimensional micro-computed tomographic, biomechanical and SEM study, Biomaterials, 2005, 5444–5453 CrossRef CAS PubMed
.
- J. M. Kim, M. H. Kim, S. S. Kang, G. Kim and S. H. Choi, Comparable bone healing capacity of different bone graft matrices in a rabbit segmental defect model, J. Vet. Sci., 2014, 15(2), 289–295 CrossRef PubMed
.
- H. Zhang, L. Yang, X. G. Yang, F. Wang, J. T. Feng and K. C. Hua,
et al., Demineralized Bone Matrix Carriers and their Clinical Applications: An Overview, Orthop. Surg., 2019, 11(5), 725–737 CrossRef PubMed
.
- P. Tournier, J. Guicheux, A. Paré, A. Maltezeanu, T. Blondy and J. Veziers,
et al., A partially demineralized allogeneic bone graft: in vitro osteogenic potential and preclinical evaluation in two different intramembranous bone healing models, Sci. Rep., 2021, 11(1), 4907 CrossRef CAS PubMed
.
- M. Taz, P. Makkar, K. M. Imran, D. W. Jang, Y. S. Kim and B. T. Lee, Bone regeneration of multichannel biphasic calcium phosphate granules supplemented with hyaluronic acid, Mater. Sci. Eng., C, 2019, 99, 1058–1066 CrossRef CAS PubMed
.
- O. Faruq, B. Kim, A. R. Padalhin, G. H. Lee and B. T. Lee, A hybrid composite system of biphasic calcium phosphate granules loaded with hyaluronic acid-gelatin hydrogel for bone regeneration, J. Biomater. Appl., 2017, 32(4), 433–445 CrossRef CAS PubMed
.
- J. L. Moreau, M. D. Weir and H. H. K. Xu, Self-setting collagen-calcium phosphate bone cement: Mechanical and cellular properties, J. Biomed. Mater. Res., Part A, 2009, 91(2), 605–613 CrossRef PubMed
.
- R. O. Neill, H. O. Mccarthy, E. B. Montufar, M. Ginebra, D. I. Wilson and A. Lennon,
et al., Acta Biomaterialia Critical review: Injectability of calcium phosphate pastes and cements, Acta Biomater., 2017, 50, 1–19 CrossRef PubMed
.
- P. Weiss, L. Obadia, D. Magne, X. Bourges, C. Rau and T. Weitkamp,
et al., Synchrotron X-ray microtomography (on a micron scale) provides three-dimensional imaging representation of bone ingrowth in calcium phosphate biomaterials, Biomaterials, 2003, 4591–4601 CrossRef CAS
.
- A. D. Speirs, T. R. Oxland, B. A. Masri, A. Poursartip and C. P. Duncan, Calcium phosphate cement composites in revision hip arthroplasty, Biomaterials, 2005, 26(35), 7310–7318 CrossRef CAS PubMed
.
- V. Campana, G. Milano, E. Pagano, M. Barba, C. Cicione and G. Salonna,
et al., Bone substitutes in orthopaedic surgery: from basic science to clinical practice, J. Mater. Sci.: Mater. Med., 2014, 25(10), 2445–2461 CrossRef CAS PubMed
.
- K. Flégeau, R. Pace, H. Gautier, G. Rethore, J. Guicheux and C. Le Visage,
et al., Toward the development of biomimetic injectable and macroporous biohydrogels for regenerative medicine, Adv. Colloid Interface Sci., 2017, 247, 589–609 CrossRef PubMed
.
- B. H. Fellah, P. Weiss, O. Gauthier, T. Rouillon, P. Pilet and G. Daculsi,
et al., Bone repair using a new injectable self-crosslinkable bone substitute, J. Orthop. Res., 2006, 24(4), 628–635 CrossRef CAS PubMed
.
- B. Hoffmann, E. Volkmer, A. Kokott, M. Weber, S. Hamisch and M. Schieker,
et al., A new biodegradable bone wax substitute with the potential to be used as a bone filling material, J. Mater. Chem., 2007, 17(38), 4028–4033 RSC
.
- D. Apelt, F. Theiss, A. O. El-Warrak, K. Zlinszky, R. Bettschart-Wolfisberger and M. Bohner,
et al., In vivo behavior of three different injectable hydraulic calcium phosphate cements, Biomaterials, 2004, 25(7–8), 1439–1451 CrossRef CAS PubMed
.
- G. Daculsi, I. Khairoun, R. Z. LeGeros, F. Moreau, P. Pilet and X. Bourges,
et al., Bone Ingrowth at the Expense of a Novel Macroporous Calcium Phosphate Cement, Key Eng. Mater., 2007, 330–332, 811–814 CAS
.
- J. Luo, H. Engqvist and C. Persson, A ready-to-use acidic, brushite-forming calcium phosphate cement, Acta Biomater., 2018, 81, 304–314 CrossRef CAS PubMed
.
- J. Zhang, F. Tancret and J. M. Bouler, Mechanical properties of Calcium Phosphate Cements (CPC) for bone substitution: Influence of fabrication and microstructure, Key Eng. Mater., 2012, 409–414 CAS
.
- I. Lodoso-Torrecilla, N. A. P. van Gestel, L. Diaz-Gomez, E. C. Grosfeld, K. Laperre and J. G. C. Wolke,
et al., Multimodal pore formation in calcium phosphate cements, J. Biomed. Mater. Res., Part A, 2018, 106(2), 500–509 CrossRef CAS PubMed
.
- A. Cherng, S. Takagi and L. C. Chow, Effects of hydroxypropyl methylcellulose and other gelling agents on the handling properties of calcium phosphate cement, J. Biomed. Mater. Res., 1997, 35(3), 273–277 CrossRef CAS PubMed
.
- S. Takagi, L. C. Chow, S. Hirayama and A. Sugawara, Premixed Calcium-Phosphate Cement Pastes, J. Biomed. Mater. Res., Part B, 2003, 67(2), 689–696 CrossRef PubMed
.
- S. Takagi and L. C. Chow, Formation of macropores in calcium phosphate cement implants, J. Mater. Sci.: Mater. Med., 2001, 12(2), 135–139 CrossRef CAS PubMed
.
- W. J. E. M. Habraken, H. B. Liao, Z. Zhang, J. G. C. Wolke, D. W. Grijpma and A. G. Mikos,
et al., In vivo degradation of calcium phosphate cement incorporated into biodegradable microspheres, Acta Biomater., 2010, 6(6), 2200–2211 CrossRef CAS PubMed
.
- J. E. Barralet, L. Grover, T. Gaunt, A. J. Wright and I. R. Gibson, Preparation of macroporous calcium phosphate cement tissue engineering scaffold, Biomaterials, 2002, 23(15), 3063–3072 CrossRef CAS PubMed
.
- M. Le Ferrec, C. Mellier, F. X. Lefèvre, F. Boukhechba, P. Janvier and G. Montavon,
et al., In vivo resorption of injectable apatitic calcium phosphate cements: Critical role of the intergranular microstructure, J. Biomed. Mater. Res., Part B, 2020, 108(2), 367–376 CrossRef CAS PubMed
.
- E. Cuzmar, R. A. Perez, M. C. Manzanares, M. P. Ginebra and J. Franch, In Vivo Osteogenic Potential of Biomimetic Hydroxyapatite/Collagen Microspheres: Comparison with Injectable Cement Pastes, PLoS One, 2015, 10(7), e0131188 CrossRef PubMed
.
- A. Bigi, P. Torricelli, M. Fini, B. Bracci, S. Panzavolta and L. Sturba,
et al., A biomimetic gelatin-calcium phosphate bone cement, Int. J. Artif. Organs., 2004, 27(8), 664–673 CrossRef CAS PubMed
.
- A. Kovtun, M. J. Goeckelmann, A. A. Niclas, E. B. Montufar, M. P. Ginebra and J. A. Planell,
et al., In vivo performance of novel soybean/gelatin-based bioactive and injectable hydroxyapatite foams, Acta Biomater., 2015, 12(1), 242–249 CrossRef CAS PubMed
.
-
M. Li, X. Liu and X. Liu, Calcium Phosphate Cement with BMP-2-loaded Gelatin Microspheres Enhances Bone Healing in Osteoporosis A Pilot Study.
- X. Cui, C. Huang, Z. Chen, M. Zhang, C. Liu and K. Su,
et al., Hyaluronic acid facilitates bone repair effects of calcium phosphate cement by accelerating osteogenic expression, Bioact. Mater., 2021, 6(11), 3801–3811 CrossRef CAS PubMed
.
- P. S. Babo, X. Cai, A. S. Plachokova, R. L. Reis, J. Jansen and M. E. Gomes,
et al., Evaluation of a platelet lysate bilayered system for periodontal regeneration in a rat intrabony three-wall periodontal defect, J. Tissue Eng. Regener. Med., 2018, 12(2), e1277–e1288 CAS
.
- J. Zhang, W. Liu, O. Gauthier, S. Sourice, P. Pilet and G. Rethore,
et al., A simple and effective approach to prepare injectable macroporous calcium phosphate cement for bone repair: syringe-foaming using a viscous hydrophilic polymeric solution, Acta Biomater., 2016, 31, 326–338 CrossRef CAS PubMed
.
- H. J. Lee, B. Kim, A. R. Padalhin and B. T. Lee, Incorporation of chitosan-alginate complex into injectable calcium phosphate cement system as a bone graft material, Mater. Sci. Eng., C, 2019, 94, 385–392 CrossRef CAS PubMed
.
- S. Wu, L. Lei, C. Bao, J. Liu, M. D. Weir and K. Ren,
et al., An injectable and antibacterial calcium phosphate scaffold inhibiting Staphylococcus aureus and supporting stem cells for bone regeneration, Mater. Sci. Eng., C, 2021, 120, 111688 CrossRef CAS PubMed
.
- A. Shimatani, H. Toyoda, K. Orita, Y. Ibara, Y. Yokogawa and H. Nakamura, A bone replacement-type calcium phosphate cement that becomes more porous in vivo by incorporating a degradable polymer, J. Mater. Sci.: Mater. Med., 2021, 32(7), 77 CrossRef CAS PubMed
.
- S. Sprio, M. Dapporto, M. Montesi, S. Panseri, W. Lattanzi and E. Pola,
et al., Novel Osteointegrative Sr-Substituted Apatitic Cements Enriched with Alginate, Mater., 2016, 9(9), 1–17 Search PubMed
.
- S. Gallinetti, G. Mestres, C. Canal, C. Persson and M. P. Ginebra, A novel strategy to enhance interfacial adhesion in fiber-reinforced calcium phosphate cement, J. Mech. Behav. Biomed. Mater., 2017, 75, 495–503 CrossRef CAS PubMed
.
- A. Aryaei, J. Liu, A. H. Jayatissa and A. Champa Jayasuriya, Cross-linked chitosan improves the mechanical properties of calcium phosphate-chitosan cement, Mater. Sci. Eng., C, 2015, 54, 14–19 CrossRef CAS PubMed
.
- L. Kjalarsdóttir, A. Dýrfjörd, A. Dagbjartsson, E. H. Laxdal, G. Örlygsson and J. Gíslason,
et al., Bone remodeling effect of a chitosan and calcium phosphate-based composite, Regener. Biomater., 2019, 6(4), 241–247 CrossRef PubMed
.
- L. Zou, X. Zou, L. Chen, H. Li, T. Mygind and M. Kassem,
et al., Effect of hyaluronan on osteogenic differentiation of porcine bone marrow stromal cells in vitro, J. Orthop. Res., 2008, 26(5), 713–720 CrossRef CAS PubMed
.
- C. Gao, H. Liu, Z. P. Luo, Sajilafu, H. Yang and L. Yang, Modification of calcium phosphate cement with poly(γ-glutamic acid) and its strontium salt for kyphoplasty application, Mater. Sci. Eng., C, 2017, 80, 352–361 CrossRef CAS PubMed
.
- M. Rödel, J. Teßmar, J. Groll and U. Gbureck, Highly flexible and degradable dual setting systems based on PEG-hydrogels and brushite cement, Acta Biomater., 2018, 182–201 CrossRef PubMed
.
- B. Zhang, J. D. Skelly, J. R. Maalouf, D. C. Ayers and J. Song, Multifunctional scaffolds for facile implantation, spontaneous fixation, and accelerated long bone regeneration in rodents, Sci. Transl. Med., 2019, 11(502), 1–13 Search PubMed
.
- M. Bohner and G. Baroud, Injectability of calcium phosphate pastes, Biomaterials, 2005, 26(13), 1553–1563 CrossRef CAS PubMed
.
- J. Zhang, W. Liu, V. Schnitzler, F. Tancret and J. M. Bouler, Calcium phosphate cements for bone substitution: Chemistry, handling and mechanical properties, Acta Biomater., 2014, 10, 1035–1049 CrossRef CAS PubMed
.
- M. J. Patel, S. Blackburn and D. I. Wilson, Modelling of paste flows subject to liquid phase migration, Int. J. Numer Methods Eng., 2007, 72(10), 1157–1180 CrossRef
.
- R. O’Neill, H. O. McCarthy, E. B. Montufar, M. P. Ginebra, D. I. Wilson and A. Lennon,
et al., Critical review: Injectability of calcium phosphate pastes and cements, Acta Biomater., 2017, 50, 1–19 CrossRef PubMed
.
- I. Lodoso-Torrecilla, E. Grosfeld, A. Marra, B. T. Smith, A. G. Mikos and D. J. Ulrich,
et al., Multimodal porogen platforms for calcium phosphate cement degradation, J. Biomed. Mater. Res., Part A, 2019, 107(8), jbm.a.36686 CrossRef PubMed
.
- E. F. Burguera, H. H. K. Xu and M. D. Weir, Injectable and rapid-setting calcium phosphate bone cement with dicalcium phosphate dihydrate, J. Biomed. Mater. Res., Part B, 2006, 77(1), 126–134 CrossRef PubMed
.
- W. Liu, J. Zhang, G. Rethore, K. Khairoun, P. Pilet and F. Tancret,
et al., A novel injectable, cohesive and toughened Si-HPMC (silanized-hydroxypropyl methylcellulose) composite calcium phosphate cement for bone substitution, Acta Biomater., 2014, 10(7), 3335–3345 CrossRef CAS PubMed
.
- M. H. Alkhraisat, C. Rueda, F. T. Mariño, J. Torres, L. B. Jerez and U. Gbureck,
et al., The effect of hyaluronic acid on brushite cement cohesion, Acta Biomater., 2009, 5(8), 3150–3156 CrossRef CAS PubMed
.
- S. Takagi, L. C. Chow, S. Hirayama and F. C. Eichmiller, Properties of elastomeric calcium phosphate cement-chitosan composites, Dent. Mater., 2003, 19(8), 797–804 CrossRef CAS PubMed
.
- H. Liu, H. Li, W. Cheng, Y. Yang, M. Zhu and C. Zhou, Novel injectable calcium phosphate/chitosan composites for bone substitute materials, Acta Biomater., 2006, 2(5), 557–565 CrossRef PubMed
.
- K. Ishikawa, Y. Miyamoto, M. Kon, M. Nagayama and K. Asaoka, Non-decay type fast-setting calcium phosphate cement: composite with sodium alginate, Biomaterials, 1995, 16(7), 527–532 CrossRef CAS PubMed
.
- L. Vojtova, L. Michlovska, K. Valova, M. Zboncak, M. Trunec and K. Castkova,
et al., The effect of the thermosensitive biodegradable PLGA–PEG–PLGA copolymer on the rheological, structural and mechanical properties of thixotropic self-hardening tricalcium phosphate cement, Int. J. Mol. Sci., 2019, 20(2), 391 CrossRef PubMed
.
- N. Eliaz and N. Metoki, Calcium Phosphate Bioceramics: A Review of Their History, Structure, Properties, Coating Technologies and Biomedical Applications, Materials, 2017, 10(4), 334 CrossRef PubMed
.
- M. Bohner, Reactivity of calcium phosphate cements, J. Mater. Chem., 2007, 17(38), 3980–3986 RSC
.
- A. Bigi, B. Bracci and S. Panzavolta, Effect of added gelatin on the properties of calcium phosphate cement, Biomaterials, 2004, 25(14), 2893–2899 CrossRef CAS PubMed
.
- T. R. Blattert, L. Jestaedt and A. Weckbach, Suitability of a calcium phosphate cement in osteoporotic vertebral body fracture augmentation: a controlled, randomized, clinical trial of balloon kyphoplasty comparing calcium phosphate versus polymethylmethacrylate, Spine (Phila Pa 1976), 2009, 34(2), 108–114 CrossRef PubMed
.
- U. G. K. Wegst, H. Bai, E. Saiz, A. P. Tomsia and R. O. Ritchie, Bioinspired structural materials, Nat. Mater., 2015, 14(1), 23–36 CrossRef CAS PubMed
.
- V. Uskoković, S. Ghosh and V. M. Wu, Antimicrobial hydroxyapatite-gelatin-silica
composite pastes with tunable setting properties, J. Mater. Chem. B, 2017, 5(30), 6065–6080 RSC
.
- J. Zhang, W. Liu, V. Schnitzler, F. Tancret and J. M. Bouler, Calcium phosphate cements for bone substitution: chemistry, handling and mechanical properties, Acta Biomater., 2014, 10(3), 1035–1049 CrossRef CAS PubMed
.
- D. J. F. Moojen, S. N. M. Spijkers, C. S. Schot, M. W. Nijhof, H. C. Vogely and A. Fleer,
et al., Identification of orthopaedic infections using broad-range polymerase chain reaction and reverse line blot hybridization, J. Bone Jt. Surg., Am. Vol., 2007, 89(6), 1298–1305 CrossRef
.
- W. P. Saunders and E. M. Saunders, Coronal leakage as a cause of failure in root-canal therapy: a review, Endod. Dent. Traumatol., 1994, 10(3), 105–108 CrossRef CAS PubMed
.
- L. C. Chow, Next generation calcium phosphate-based biomaterials, Dent. Mater. J., 2009, 28(1), 1–10 CrossRef CAS PubMed
.
- H. Chen, H. Yang, M. D. Weir, A. Schneider, K. Ren and N. Homayounfar,
et al., An antibacterial and injectable calcium phosphate scaffold delivering human periodontal ligament stem cells for bone tissue engineering, RSC Adv., 2020, 10(66), 40157–40170 RSC
.
- A. Bigi and E. Boanini, Calcium Phosphates as Delivery Systems for Bisphosphonates, J. Funct. Biomater., 2018, 9(1), 6 CrossRef PubMed
.
- M. P. Ginebra, T. Traykova and J. A. Planell, Calcium phosphate cements: Competitive drug carriers for the musculoskeletal system?, Biomaterials, 2006, 27(10), 2171–2177 CrossRef CAS PubMed
.
- M. F. Di Filippo, L. S. Dolci, B. Albertini, N. Passerini, P. Torricelli and A. Parrilli,
et al., A radiopaque calcium phosphate bone cement with long-lasting antibacterial effect: from paste to injectable formulation, Ceram. Int., 2020, 46(8), 10048–10057 CrossRef CAS
.
- S. Wu, L. Lei, C. Bao, J. Liu, M. D. Weir and K. Ren,
et al., An injectable and antibacterial calcium phosphate scaffold inhibiting Staphylococcus aureus and supporting stem cells for bone regeneration, Mater. Sci. Eng., C, 2021, 120 Search PubMed
.
- A. Ewald, D. Hösel, S. Patel, L. M. Grover, J. E. Barralet and U. Gbureck, Silver-doped calcium phosphate cements with antimicrobial activity, Acta Biomater., 2011, 7(11), 4064–4070 CrossRef CAS PubMed
.
- S. Wu, L. Lei, C. Bao, J. Liu, M. D. Weir and K. Ren,
et al., An injectable and antibacterial calcium phosphate scaffold inhibiting Staphylococcus aureus and supporting stem cells for bone regeneration, Mater. Sci. Eng., C, 2021, 120 Search PubMed
.
- A. Barba, A. Diez-Escudero, Y. Maazouz, K. Rappe, M. Espanol and E. B. Montufar,
et al., Osteoinduction by Foamed and 3D-Printed Calcium Phosphate Scaffolds: Effect of Nanostructure and Pore Architecture, ACS Appl. Mater. Interfaces, 2017, 9(48), 41722–41736 CrossRef CAS PubMed
.
- A. Jain, L. S. Duvvuri, S. Farah, N. Beyth, A. J. Domb and W. Khan, Antimicrobial Polymers, Adv. Healthcare Mater., 2014, 3(12), 1969–1985 CrossRef CAS PubMed
.
- N. F. Kamaruzzaman, L. P. Tan, R. H. Hamdan, S. S. Choong, W. K. Wong and A. J. Gibson,
et al., Antimicrobial polymers: the potential replacement of existing antibiotics?, Int. J. Mol. Sci., 2019, 20, 2747 CrossRef CAS PubMed
.
- J. Hoque, R. G. Prakash, K. Paramanandham, B. R. Shome and J. Haldar, Biocompatible injectable hydrogel with potent wound healing and antibacterial properties, Mol. Pharm., 2017, 14(4), 1218–1230 CrossRef CAS PubMed
.
- A. Yokoyama, S. Yamamoto, T. Kawasaki, T. Kohgo and M. Nakasu, Development of calcium phosphate cement using chitosan and citric acid for bone substitute materials, Biomaterials, 2002, 23(4), 1091–1101 CrossRef CAS PubMed
.
- D. Meng, L. Dong, Y. Yuan and Q. Jiang, In vitro and in vivo analysis of the biocompatibility of two novel and injectable calcium phosphate cements, Regener. Biomater., 2019, 6(1), 13–19 CrossRef CAS PubMed
.
- F. Wu, H. Zhao, J. Shi, L. Long, Z. Yang and H. Jin,
et al., Preparation and evaluation of an injectable curcumin loaded chitosan/hydroxyapatite cement, J. Biomater. Appl., 2021, 885328221991946 Search PubMed
.
- E. Mariani, G. Lisignoli, R. M. Borzì and L. Pulsatelli, Biomaterials: foreign bodies or tuners for the immune response?, Int. J. Mol. Sci., 2019, 20, 636 CrossRef CAS PubMed
.
- C. Montoya, Y. Du, A. L. Gianforcaro, S. Orrego, M. Yang and P. I. Lelkes, On the road to smart biomaterials for bone research: definitions, concepts, advances, and outlook, Bone Res., 2021, 9(1), 1–16 CrossRef PubMed
.
- B. N. Brown and S. F. Badylak, Expanded applications, shifting paradigms and an improved understanding of host-biomaterial interactions, Acta Biomater., 2013, 9(2), 4948–4955 CrossRef CAS PubMed
.
- T. J. Levingstone, S. Herbaj and N. J. Dunne, Calcium Phosphate Nanoparticles for Therapeutic Applications in Bone Regeneration, Nanomater., 2019, 9(11), 1570 CrossRef CAS PubMed
.
- I. Denry and L. T. Kuhn, Design and characterization of calcium phosphate ceramic scaffolds for bone tissue engineering, Dent. Mater., 2016, 32(1), 43–53 CrossRef CAS PubMed
.
- C. Rey, C. Combes, C. Drouet, D. Grossin, G. Bertrand and J. Soulié, 1.11 Bioactive Calcium Phosphate Compounds: Physical Chemistry, Compr. Biomater. II, 2017, 244–290 CAS
.
- R. Z. LeGeros, Properties of osteoconductive biomaterials: calcium phosphates, Clin. Orthop. Relat. Res., 2002, 81–98 CrossRef PubMed
.
- L. C. Chow, Calcium phosphate cements: chemistry, properties, and applications, Mater. Res. Soc. Symp. Proc., 2000, 599, 27–37 CrossRef CAS
.
- P. Weiss, L. Obadia, D. Magne, X. Bourges, C. Rau and T. Weitkamp,
et al., Synchrotron X-ray microtomography (on a micron scale) provides three-dimensional imaging representation of bone ingrowth in calcium phosphate biomaterials, Biomaterials, 2003, 24(25), 4591–4601 CrossRef CAS PubMed
.
- S. Wenisch, J. P. Stahl, U. Horas, C. Heiss, O. Kilian and K. Trinkaus,
et al., In vivo mechanisms of hydroxyapatite ceramic degradation by osteoclasts: fine structural microscopy, J. Biomed. Mater. Res., Part A, 2003, 67(3), 713–718 CrossRef CAS PubMed
.
- R. Detsch, H. Mayr and G. Ziegler, Formation of osteoclast-like cells on HA and TCP ceramics, Acta Biomater., 2008, 4(1), 139–148 CrossRef CAS PubMed
.
- V. Karageorgiou and D. Kaplan, Porosity of 3D biomaterial scaffolds and osteogenesis, Biomaterials, 2005, 26, 5474–5491 CrossRef CAS PubMed
.
- A. Vezenkova and J. Locs, Sudoku of porous, injectable calcium phosphate cements – Path to osteoinductivity, Bioact. Mater., 2022, 17, 109–124 CrossRef CAS PubMed
.
- P. Habibovic, H. Yuan, C. M. Van Der Valk, G. Meijer, C. A. Van Blitterswijk and K. De Groot, 3D microenvironment as essential element for osteoinduction by biomaterials, Biomaterials, 2005, 26(17), 3565–3575 CrossRef CAS PubMed
.
-
I. Khairoun, P. Weiss and J.-M. Bouler, “Macroporous and highly resorbable apatitic calcium-phosphate cement”, EP1891984 A1, 2008 Search PubMed
.
- T. J. Blokhuis, M. F. Termaat, F. C. Den Boer, P. Patka, F. C. Bakker and H. J. T. M. Haarman, Properties of calcium phosphate ceramics in relation to their in vivo behavior, J. Trauma: Inj., Infect., Crit. Care, 2000, 179–186 CrossRef CAS PubMed
.
- F. Perut, E. B. Montufar, G. Ciapetti, M. Santin, J. Salvage and T. Traykova,
et al., Novel soybean/gelatine-based bioactive and injectable hydroxyapatite foam:
material properties and cell response, Acta Biomater., 2011, 7(4), 1780–1787 CrossRef CAS PubMed
.
- M. P. Ginebra, J. A. Delgado, I. Harr, A. Almirall, S. Del Valle and J. A. Planell, Factors affecting the structure and properties of an injectable self-setting calcium phosphate foam, J. Biomed. Mater. Res., Part A, 2007, 80(2), 351–361 CrossRef PubMed
.
- M. Bohner and R. J. Miron, A proposed mechanism for material-induced heterotopic ossification, Mater. Today, 2019, 22, 132–141 CrossRef CAS
.
- M. Bohner and R. J. Miron, A proposed mechanism for material-induced heterotopic ossification, Mater. Today, 2019, 22, 132–141 CrossRef CAS
.
- S. D. Valle, N. Miño, F. Muñoz, A. González, J. A. Planell and M. P. Ginebra, In vivo evaluation of an injectable Macroporous Calcium Phosphate Cement, J. Mater. Sci.: Mater. Med., 2007, 18(2), 353–361 CrossRef PubMed
.
- S. Kung, H. Devlin, E. Fu, K. Y. Ho, S. Y. Liang and Y. D. Hsieh, The osteoinductive effect of chitosan-collagen composites around pure titanium implant surfaces in rats, J. Periodontal Res., 2011, 46(1), 126–133 CrossRef CAS PubMed
.
- L. Huang, Y. Y. Cheng, P. L. Koo, K. M. Lee, L. Qin and J. C. Y. Cheng,
et al., The effect of hyaluronan on osteoblast proliferation and differentiation in rat calvarial-derived cell cultures, J. Biomed. Mater. Res., Part A, 2003, 66(4), 880–884 CrossRef CAS PubMed
.
|
This journal is © The Royal Society of Chemistry 2022 |
Click here to see how this site uses Cookies. View our privacy policy here.