DOI:
10.1039/D0MA00659A
(Paper)
Mater. Adv., 2020,
1, 2862-2871
Tris(triazolo)triazine-based emitters for solution-processed blue thermally activated delayed fluorescence organic light-emitting diodes†‡
Received
31st August 2020
, Accepted 13th October 2020
First published on 13th October 2020
Abstract
We report a new emitter 3,4,5-3TCz-TTT based on a tris(triazolo)triazine acceptor that shows thermally activated delayed fluorescence and cross-compare its performance with the recently reported analogue, 3DMAC-TTT. These compounds show blue emission and delayed fluorescence with delayed lifetimes on the order of milliseconds. Solution-processed organic light-emitting diodes achieving maximum external quantum efficiencies, EQEmax, of 5.8% for 3,4,5-3TCz-TTT and 11.0% for 3DMAC-TTT.
Introduction
Organic light-emitting diodes (OLEDs) generate light by the recombination of electrically generated holes and electrons to form excitons that subsequently radiatively decay. Due to spin-statistics, 75% of the generated excitons are triplets, while 25% are singlet excitons.1,2 Thermally activated delayed fluorescence (TADF) is considered one of the most promising mechanisms for efficient electroluminescent devices2–5 as 100% of the excitons can be converted to light. A small energy gap between the lowest singlet (S1) and triplet (T1) excited states (ΔEST) enables triplet excitons to be converted to singlet excitons by reverse intersystem crossing (RISC) at ambient temperatures, which can subsequently relax via the fluorescence channel. Typically, a small ΔEST is achieved by the spatial separation of the highest occupied molecular orbital (HOMO) and the lowest unoccupied molecular orbital (LUMO). The classic design strategies are based on the combination of donor and acceptor groups and their small electronic coupling either via (1) a twisted intramolecular charge transfer6–11 transition, (2) a through-space charge-transfer transition,7,8,12 (3) a spiro-conjugation charge transfer transition13,14 or (4) an alternating HOMO/LUMO distribution in p,n-doped polycyclic aromatic hydrocarbons, termed a multi-resonance TADF transition.2,15–18
By employing solution-based processing techniques,19–21 large-area OLEDs becomes simpler and more cost-efficient to fabricate. However, solution-processed devices often show inferior performance compared to the vacuum-deposited counterparts.22–25 For solution-processed devices to become competitive, their performance must improve to rival vacuum-sublimed devices. Typically, solution-processed devices rely on polymer emitters as the use of polymers produces high-quality, defect-free amorphous films. TADF polymers have shown promise as emitters in solution-processed devices.23,26–28 However, the synthesis of polymers will lead to a mixture of compounds with different molecular weight, each of which will have a slightly different set of photophysical properties that will result in a broadened emission spectrum.29 Moreover, the photophysical properties of TADF polymers usually suffer due to quenching via intra- and inter-molecular charge transfer between the TADF units on the polymer.30,31 Dendrimeric TADF emitters also show good solubility and generate high-quality amorphous films. OLED performance, however, tends to fall off rapidly with increasing generation size of the dendron and thus far OLEDs using dendrimer emitters have underperformed compared to their polymer and small molecule emitter counterparts.32 The use of small molecule emitters has to date produced the highest performance solution-processed OLEDs. Typically, solubilizing groups are decorated around a known emitter core to improve the film morphology of the emissive layer in solution-processed OLEDs. An example of this can be found in the work of Cho et al. where they investigated the effect that solution-processing and vacuum deposition have on the performance of OLEDs, employing three related TADF emitters: 4CzIPN, m4CzIPN and t4CzIPN (Fig. 1).33 The solution-processed device of 4CzIPN shows a substantial drop in performance compared to the vacuum-deposited OLED (maximum external quantum efficiency, EQEmax, decreasing 26.0% to 8.1%). The incorporation of methyl groups in m4CzIPN has no effect on the efficiency of the solution-processed device, leading again to inferior efficiencies than the evaporated OLED (from 19.6% to 8.2%). By contrast, the use of tert-butylcarbazole in t4CzIPN resulted in a much-enhanced solubility of the emitter, stabilizing the morphology of the solution-processed film, which translated into a solution-processed OLED with comparable efficiency to the vacuum-deposited device (17.1% for the vacuum-deposited device and 18.3% for the solution-processed device). In a similar vein, Chen et al. reported that the device efficiency could be significantly improved from 8.0% to 19.1% by replacement of carbazole with tert-butycarbazole in triarylborane-based TADF emitters.34 Acridine-based emitters have also been successfully employed in several solution-processed devices. For instance, Wada et al. reported a solution-processed OLED using triazine-based TADF emitter 3ACR-TRZ, which showed an EQEmax of 18.6%.35 The same group reported a high-performance solution-processed blue OLED (CIE: 0.15, 0.19) using MA-TA as the emitter, which achieved an EQEmax of 22.1%.36
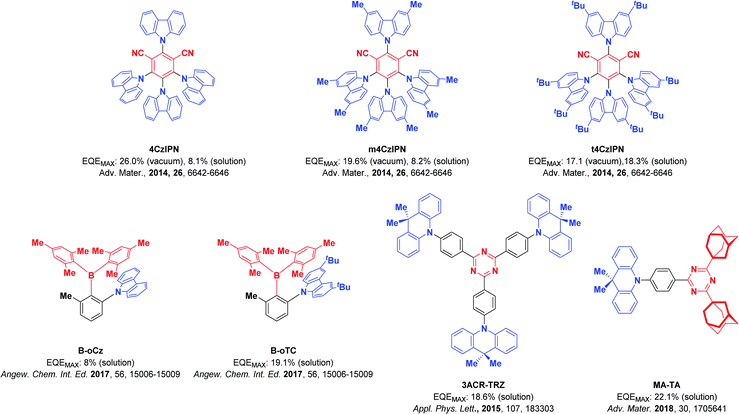 |
| Fig. 1 Molecular structures and performances of the discussed solution-processed materials. | |
1,2,4-Triazoles37 and 1,3,5-triazines2,38 have been widely applied as acceptor motifs in TADF materials due to their relatively weak electron-withdrawing character (the LUMO levels are 0.43 eV for 4H-1,2,4-triazole and −1.80 eV for 2,4,6-triphenyl-1,3,5-triazine).39 The combination of these two motifs into a single acceptor in the [1,2,4]-triazolo-[1,3,5]-triazine core (TTT) was synthesized by annulation of three triazole heterocycles onto a central triazine. This motif was first reported in 1911 by Hofmann and Erhardt,40,41 while Wystrach and co-workers investigated the structure of a triamine TTT derivative in 1953.42 Then, in 1961, Huisgen et al. were the first to synthesize the first TTT core substituted with phenyl groups by reacting cyanuric chloride with 5-phenyl tetrazole.43 In 2008, Longo et al. substituted the TTT core with peripheral flexible alkyl chains, endowing the molecule with liquid crystalline character.44 The disc-like core with the peripheral aliphatic side chains led to luminescent and charge-transporting materials known to self-assemble into columnar superstructures driven by π-stacking.
Concurrent with our work, Pathak et al. recently reported the first two examples of emitters employing the TTT motif within a TADF emitter design: TTT-PXZ and TTT-DMAC (Fig. 2).45 These emitters possess moderate photoluminescence quantum yields (ΦPL) of 39.5% and 21.4%, respectively, accompanied by short delayed fluorescence lifetimes of 4.2 μs and 4.6 μs, respectively. The emission of TTT-PXZ is expectedly considerably red-shifted given the stronger donor employed, with a λPL of 522 nm compared to 468 nm for TTT-DMAC. The improved device performance of TTT-PXZ is in part due to the smaller ΔEST 0.07 eV compared to 0.20 eV for TTT-DMAC. Unfortunately, the devices in this study were poor with EQEmax of 6.2 and 1.9% for the OLEDs with TTT-PXZ and TTT-DMAC, respectively, and associated severe efficiency roll-off.
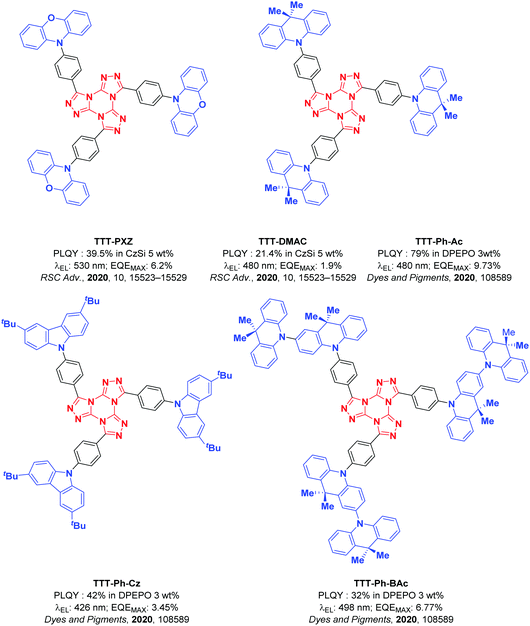 |
| Fig. 2 Molecular structure and OLED performance metrics of known TTT emitters. | |
Wang et al.46 expanded upon this work by improving the efficiency of the DMAC-based OLED (they named the TADF emitter as TTT-Ph-Ac in their work) to 9.73%. They also introduced two new TTT-based emitters: TTT-Ph-Cz and TTT-Ph-BAc (Fig. 2). TTT-Ph-Cz did not present a delayed component, reflected in the low EQEmax. TTT-Ph-BAc shows a delayed lifetime of 50.7 μs, but the ΦPL is significantly lower at 32%, which translated to a lower EQEmax for the OLED using this emitter than in the device using TTT-Ph-Ac.
Here, we introduce a new TTT-based emitter (Fig. 3), 3,4,5-3TCz-TTT, and cross-compare its performance with the recently reported compound 3DMAC-TTT (aka TTT-DMAC/TTT-Ph-Ac).
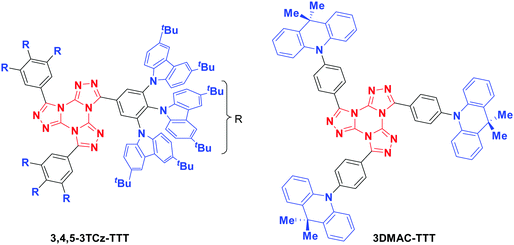 |
| Fig. 3 Molecular structures of 3,4,5-3TCz-TTT and 3DMAC-TTT. | |
Synthesis
The synthesis for the two emitters is shown in Scheme 1. Starting from the 4-fluorobenzonitrile precursors, the DMAC and tert-butylcarbazole (TCz) donor groups were attached under basic SNAr conditions. A 1,3-dipolar cycloaddition reaction with sodium azide and ammonium chloride in DMF at elevated temperatures led to the tetrazole precursors. Following a slightly modified procedure to the literature,43,47 the TTT core was assembled through a condensation between trichlorotriazine and three equivalents of the tetrazole, yielding 3DMAC-TTT and 3,4,5-3TCz-TTT in very good yields. The identity and purity of the two emitters were determined by 1H NMR, 13C NMR, Elemental Analysis (EA) and High-Resolution Mass Spectrometry (HRMS).
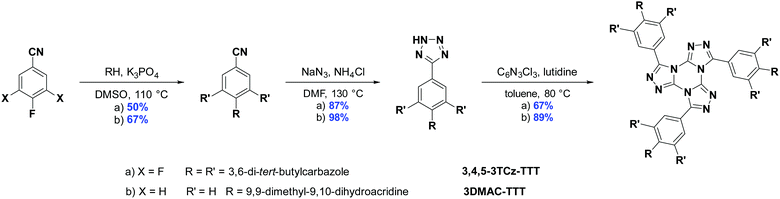 |
| Scheme 1 Synthetic route to 3,4,5-3TCz-TTT and 3DMAC-TTT. | |
Theoretical calculations
To assess the optoelectronic properties of these two compounds, we performed a combination of Density Functional Theory (DFT) calculation and Time-Dependent DFT within the Tamm–Dancoff approximation (TDA-DFT48) in the gas phase using the PBE0 functional49 and the 6-31G(d,p) basis set.50 These calculations permitted an assessment of the energies and electron density distributions of the frontier molecular orbitals and the nature and energies of the lowest-lying singlet and triplet excited states (Fig. 4). The ground-state optimization of 3,4,5-3TCz-TTT did not converge due to the large size of the molecule given a large number of tert-butyl groups and we thus instead modelled a methyl-substituted analogue (3,4,5-3MCz-TTT), which we hypothesized would present the same electronic structure. The calculated HOMO and LUMO values for 3,4,5-3MCz-TTT are −5.39 eV and −2.14 eV, respectively, while those of 3DMAC-TTT are −5.39 eV and −1.93 eV, respectively. The different LUMO levels, despite the same acceptor, reflect the different extent of the conjugation present across the TTT core that is governed by the relative sterics of the donor groups. The ΔEST for 3,4,5-3MCz-TTT is 0.16 eV while for 3DMAC-TTT it is 0.01 eV, reflecting the more orthogonal conformation of the donor with respect to the phenylene bridge (DFT calculated torsions are 57.1° for 3,4,5-3TCz-TTTversus 99.5° for 3DMAC-TTT). Given the presence of three donor groups about the central TTT core, there is significant degeneracy of the low-lying triplet states. For 3,4,5-3MCz-TTT we can also observe the presence of 5 other intermediate triplet states below the S1 level, where T1, T2, T3 and T4, T5, T6 each have almost identical energies. The large density of triplet states is a desirable trait that can facilitate RISC.51–54 For 3DMAC-TTT, there are three degenerate triplet states present. The oscillator strength (f) values for the associated CT transitions from the S1 state vary markedly at 0.1 and 0.0014 for 3,4,5-3MCz-TTT and 3DMAC-TTT, respectively.
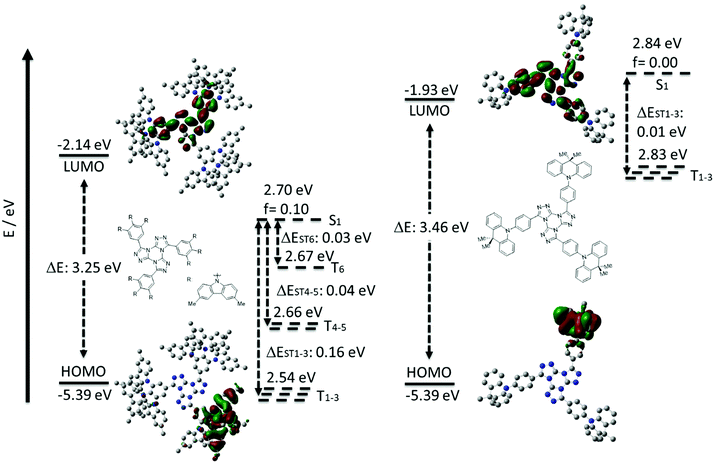 |
| Fig. 4 HOMO and LUMO electron density distributions and energy levels (in eV), excited state energy levels (in eV) 3,4,5-3MCz-TTT (right) and 3DMAC-TTT (left) (PBE0/6-31G(d,p)). | |
Optoelectronic properties
The electrochemistry of the two emitters was studied in degassed acetonitrile (Fig. 5). The CV of 3,4,5-3TCz-TTT presents three irreversible oxidation waves at 1.15 V, 1.34 V, 1.51 V vs. SCE (peaks from DPV), which are assigned to the sequential oxidation of the three TCz groups on each of the arms of the TTT core, values in line for the oxidation potentials of DTCz-containing compounds.55–593DMAC-TTT shows one irreversible anodic oxidation wave at 0.91 V vs. SCE; the value reported in the literature is 1.15 V vs. Fc/Fc+ in MeCN.45 Both emitters present a weak reduction wave at −1.76 V and −1.90 V for 3,4,5-3TCz-TTT and 3DMAC-TTT, respectively, assigned to the TTT core. The corresponding HOMO and LUMO values for 3,4,5-3TCz-TTT and 3DMAC-TTT are −5.57 eV, −2.66 eV and −5.33 eV, −2.52 eV, respectively.
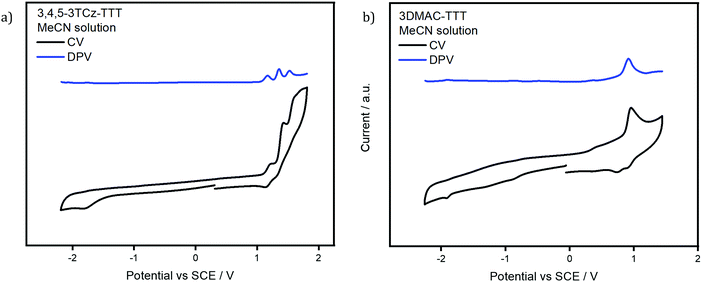 |
| Fig. 5 Cyclic voltammogramn (CV) and differential pulse voltammogram (DPV) of (a) 3,4,5-3TCz-TTT and (b) 3DMAC-TTTversus saturated calomel electrode (SCE) in MeCN and (scan rate: 0.1 V s−1). | |
The UV-vis absorption spectrum in DCM (Fig. S23, ESI‡) for 3DMAC-TTT coincides with the one measured by Pathak et al.45 Both molecules present a low intensity, low energy absorption band around 370 nm that is assigned to an ICT transition from the donor groups to the TTT acceptor. The band at 290 nm is a π–π* locally excited (LE) transition of the DMAC donor. There are four additional absorption bands for 3,4,5-3TCz-TTT which, by TD-DFT analysis, are assigned to different charge transfer states involving the carbazoles and the bridging phenyl. The absorption spectra for both compounds are not affected by changes in solvent polarity (Fig. 6). By contrast, the broad and unstructured emission red-shifts with increasing solvent polarity, indicating an emissive state that is charge-transfer in nature.
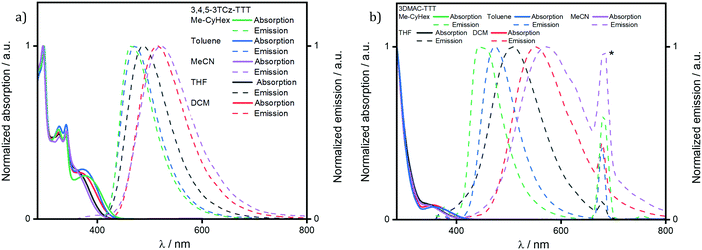 |
| Fig. 6 Solvatochromism study of (a) 3,4,5-3TCz-TTT and (b) 3DMAC-TTT. (λexc = 340 nm. * = second harmonic band of the excitation source). | |
A comparison of the excitation and steady-state emission spectra of the two emitters in DCM is shown in Fig. 6a. Due to the use of stronger DMAC donors, the emission of 3DMAC-TTT with λPL at 551 nm is red-shifted compared to that of 3,4,5-3TCz-TTT (λPL = 516 nm); the excitation spectra mirror the corresponding absorption spectra. The time-resolved PL decays in degassed DCM are shown in Fig. 7b. Both compounds show mono-exponential decay kinetics (Fig. S25a, ESI‡) with prompt lifetimes, τp, of 21.8 ns and 21.6 ns for 3,4,5-3TCz-TTT and 3DMAC-TTT, respectively, and delayed lifetimes, τd, of 89.7 μs for 3,4,5-3TCz-TTT and 3.6 μs for 3DMAC-TTT. Upon exposure to air, the delayed emission was completely suppressed, which is evidence of accessible triplet states that are quenched by oxygen (Tables 1 and 2).
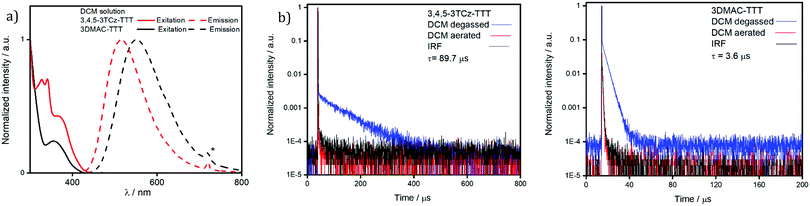 |
| Fig. 7 (a) Emission and excitation spectra of 3,4,5-3TCz-TTT and 3DMAC-TTT (DCM 10−5 M solutions, λexc = 360 nm, * = second harmonic of the excitation source). Time-resolved spectroscopy of degassed and aerated solutions of (b) 3,4,5-3TCz-TTT and (c) 3DMAC-TTT (DCM 10−5 M solutions, λexc = 378 nm). | |
Table 1 Solution-state photophysical data
Compound |
λ
PL
/nm |
Φ
PL
/% |
E
gap
/eV |
τ
p, τdd/ns; μs |
HOMOe/eV |
LUMOe/eV |
In degassed 10−5 M DCM solution.
Obtained via the relative method (see ESI), quinine sulfate in H2SO4 (aq) was used as the reference (Φr = 54.6%),60λexc = 360 nm.
Obtained from the intersection of the normalized absorption and emission spectra in DCM.
τ
p (prompt lifetime) and τd (delayed lifetime) were obtained from the transient PL decay of 3,4,5-3TCz-TTT and 3DMAC-TTT in DCM.
HOMO and LUMO were obtained from the redox potentials from the DPV, EHOMO/LUMO = −(Eox/red + 4.8) where Eox/red were taken from DPV scan corrected vs. Fc/Fc+.
|
3,4,5-3TCz-TTT
|
516 |
23 |
2.88 |
21.8; 89.7 |
−5.57 |
−2.66 |
3DMAC-TTT
|
551 |
13 |
3.01 |
21.6; 3.6 |
−5.33 |
−2.52 |
Table 2 Photophysical properties of 15 wt% 3,4,5-3TCz-TTT and 3DMAC-TTT doped films in CzSi
Compound |
λ
PL
/nm |
Φ
PL air; N2b/% |
τ
p, τdc/ns; ms |
S1d/eV |
T1e/eV |
ΔESTf/eV |
Measured at room temperature.
λ
exc = 340 nm.
τ
p (prompt lifetime) and τd (delayed lifetime) were obtained from the transient PL decay of doped film, λexc = 378 nm.
S1 was obtained from the onset of the prompt emission measured at 77 K, λexc = 343 nm.
T1 was obtained from the onset of the phosphorescence spectra measured at 77 K, λexc = 343 nm.
Obtained from the difference of S1 and T1.
|
3,4,5-3TCz-TTT
|
485 |
47; 80 |
14.5; 3.1 |
2.91 |
2.70 |
0.21 |
3DMAC-TTT
|
475 |
28; 79 |
11.9; 4.7 |
2.92 |
2.65 |
0.27 |
We next identified CzSi as an appropriate host matrix for OLEDs based on its high triplet energy of 3.02 eV.2 A doping concentration of 15 wt% was chosen as ΦPL was highest (Table S6, ESI‡). Both compounds present sky-blue emission, at 485 nm and 475 nm (Fig. 8b) and high ΦPL under N2 of 80% and 79% for 3,4,5-3TCz-TTT and 3DMAC-TTT, respectively. Both emitters present prompt fluorescence (Fig. S25b, ESI‡), with similar lifetimes reflecting tri-exponential decay kinetics, of 14.5 ns [τ1 = 26.14 ns (12.35%), τ2 = 12.97 ns (63.43%), τ3 = 22.71 (24.22%)] for 3,4,5-3TCz-TTT and bi-exponential decay kinetics 11.9 ns [τ1 = 6.95 ns (34.7%), τ2 = 14.6 (65.3%)] for 3-DMAC-TTT. Both compounds also show very long delayed PL with bi-exponential decay kinetics and lifetimes of 3.1 ms [τ1 = 1.17 ms (54.27%), τ2 = 5.30 ms (45.73%)] for 3,4,5-3TCz-TTT and 4.7 ms [τ1 = 1.16 ms (46.84%), τ2 = 7.84 ms (53.16%)] for 3-DMAC-TTT (Fig. 8a). After investigation at longer time-windows compared to the reference paper,45 we found that the two emitters present delayed fluorescence with lifetimes of the order of milliseconds, which is the result of suppression of non-radiative decay in the film, compared to solution and is reflective also in the enhanced photoluminescence quantum yield in the film. Temperature-dependent time-resolved decay curves (Fig. 9) reveal the increase in the intensity of the delayed emission with increasing temperature, which is a hallmark of compounds showing TADF. The ΔEST values of 0.21 eV for 3,4,5-3TCz-TTT and 0.27 eV for 3DMAC-TTT were determined from the onset of the prompt fluorescence and phosphorescence spectra on the CzSi films measured at 77 K (Fig. 8c and d). The latter result is slightly higher compared to that measured by Pathak et al.45 (ΔEST = 0.20 eV) in the same host matrix.
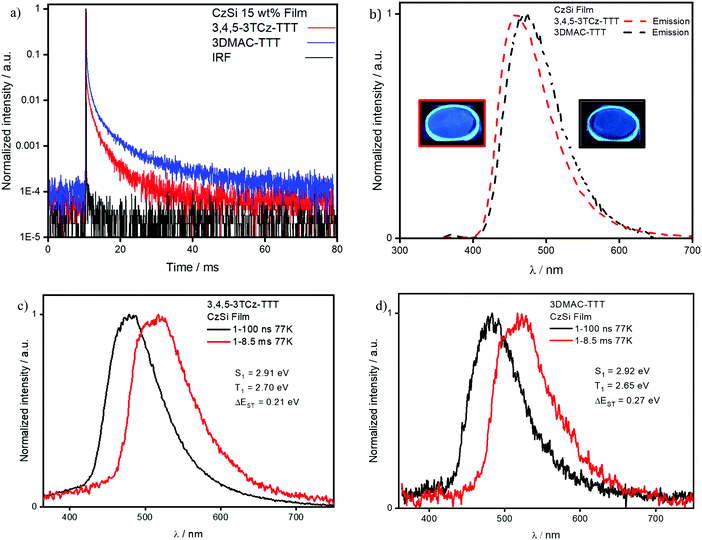 |
| Fig. 8 (a) Time-resolved PL decay of 3,4,5-3TCz-TTT and 3DMAC-TTT in spin-coated CzSi film (15 wt%); (b) emission spectra of 3,4,5-3TCz-TTT and 3DMAC-TTT in CzSi doped film (15 wt%) (λexc = 340 nm); prompt fluorescence spectra at 77 K, and phosphorescence spectra at 77 K in 15 wt% CzSi of (c) 3,4,5-3TCz-TTT and (d) 3DMAC-TTT, phosphorescence obtained at 77 K at a delay of 1 ms for 8.5 ms, λexc = 343 nm. | |
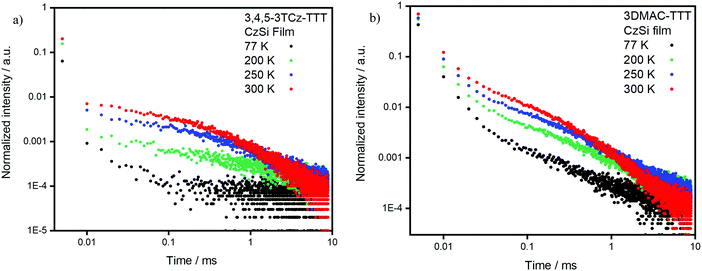 |
| Fig. 9 (a) 3,4,5-3TCz-TTT and (b) 3DMAC-TTT delayed fluorescent decay data measured at different temperatures in 15 wt% doped CzSi film (λexc = 378 nm). | |
Solution-processed organic light-emitting diodes
We fabricated solution-processed OLEDs using 3,4,5-3TCz-TTT and 3DMAC-TTT as emitters. The device structures consist of: indium tin oxide (ITO)/poly(3,4-ethylenedioxythiophene)–poly(styrenesulfonate) (PEDOT:PSS)/poly(9-vinylcarbazole) (PVK)/15 wt% Emitter:CzSi/dibenzo[b,d]furan-2,8-diylbis(diphenylphosphine oxide) (PPF)/1,3,5-tris(1-phenyl-1H-benzo[d]imidazol-2-yl)benzene (TPBi)/lithium quinolin-8-olate (Liq)/Al. PEDOT:PSS, PVK and emitter layers were each deposited by spin-coating and other layers were vacuum-deposited. Here, we inserted the PVK layer to prevent electron leakage from the emitter layer into PEDOT:PSS layer and to confine triplet exciton within the emitter layer. 3,4,5-3TCz-TTT and 3DMAC-TTT exhibited blue emission with CIE coordinates of (0.17, 0.28) and (0.16, 0.23) and EQEmax of 5.8% and 11.0%, respectively (Fig. 10). Compared to the reported work,453DMAC-TTT-based OLED exhibited improved EQEmax where the same CzSi host was used. This is probably because better exciton confinement within emitter layer was achieved by inserting PVK layer. Unfortunately, 3,4,5-3TCz-TTT exhibited lower EQE than that of 3DMAC-TTT, although the device structures are the same. As shown in Fig. S26 (ESI‡), different current density–voltage characteristics were observed, which can be attributed to the different carrier transporting and/or recombination behavior of emitters. From the PLQY and PL lifetime measurements, higher EQE for 3,4,5-3TCz-TTT OLEDs can be expected when the device structure is further optimized.
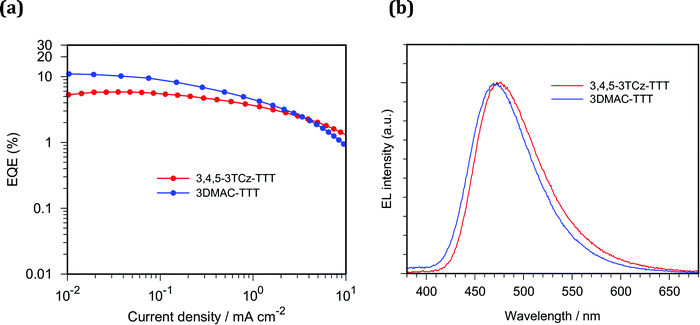 |
| Fig. 10 (a) EQE-current-density characteristics and (b) EL spectra of OLEDs at the current density of 1 mA cm−2. Performances of OLEDs containing 3,4,5-3TCz-TTT and 3DMAC-TTT as emitters are displayed in red and blue, respectively. | |
Conclusions
We report two examples of TADF molecules using the TTT as a central acceptor. The two compounds emit in the blue and show a long-lived delayed fluorescence with similar lifetimes of 3.1 ms for the TCz-based emitter and 4.7 ms for the DMAC-containing emitter in CzSi. High photoluminescence quantum yield values of 80% and 79% were observed in CzSi for 3,4,5-3TCz-TTT and 3DMAC-TTT, respectively. Both emitters were thus used in solution-processed OLEDs, showing EQEmax of 5.8% and 11% for the 3,4,5TCz-TTT and 3DMAC-TTT based-devices, respectively. The performance of the 3DMAC-TTT based is improved compared to previous reports owing to improved exciton management.
Conflicts of interest
There are no conflicts to declare.
Acknowledgements
The KIT authors are part of the Cluster 3D Matter Made To Order under Germany's Excellence Strategy (3DMM2O-EXC-2082-390761711), which has been funded by the German Research Foundation. This work was supported, in part, by the Cooperative Research Center (Sonderforschungsbereich SFB 1176) “Molecular Structuring of Soft Matter”. This work was partly supported by JSPS KAKENHI grant no. 17H01231 and 17J09631, and by the International Joint Usage/Research Centre (iJURC) at the Institute for Chemical Research (ICR), Kyoto University, Japan. The St Andrews authors thank EU Horizon 2020 Grant Agreement No. 812872 (TADFlife) for funding. EZ-C acknowledges the International Collaborative Research Program of iJURC/ICR, Kyoto University (Grant No. 2020-37).
References
- M. A. Baldo, D. F. O’Brien, Y. You, A. Shoustikov, S. Sibley, M. E. Thompson and S. R. Forrest, Nature, 1998, 395, 151–154 CrossRef CAS.
- M. Y. Wong and E. Zysman-Colman, Adv. Mater., 2017, 29, 1605444 CrossRef.
- X. Cai and S. J. Su, Adv. Funct. Mater., 2018, 28, 1–33 Search PubMed.
- Y. Tao, K. Yuan, T. Chen, P. Xu, H. Li, R. Chen, C. Zheng, L. Zhang and W. Huang, Adv. Mater., 2014, 26, 7931–7958 CrossRef CAS.
- Y. Liu, C. Li, Z. Ren, S. Yan and M. R. Bryce, Nat. Rev. Mater., 2018, 3, 18020 CrossRef CAS.
- G. Méhes, H. Nomura, Q. Zhang, T. Nakagawa and C. Adachi, Angew. Chem., Int. Ed., 2012, 51, 11311–11315 CrossRef.
- K. Kawasumi, T. Wu, T. Zhu, H. S. Chae, T. Van Voorhis, M. A. Baldo and T. M. Swager, J. Am. Chem. Soc., 2015, 137, 11908–11911 CrossRef CAS.
- E. Spuling, N. Sharma, I. D. W. Samuel, E. Zysman-Colman and S. Bräse, Chem. Commun., 2018, 54, 9278–9281 RSC.
- A. Pershin, D. Hall, V. Lemaur, J. C. Sancho-Garcia, L. Muccioli, E. Zysman-Colman, D. Beljonne and Y. Olivier, Nat. Commun., 2019, 10, 3–7 CrossRef.
- H. Kaji, H. Suzuki, T. Fukushima, K. Shizu, K. Suzuki, S. Kubo, T. Komino, H. Oiwa, F. Suzuki, A. Wakamiya, Y. Murata and C. Adachi, Nat. Commun., 2015, 6, 2–9 Search PubMed.
- D. H. Ahn, S. W. Kim, H. Lee, I. J. Ko, D. Karthik, J. Y. Lee and J. H. Kwon, Nat. Photonics, 2019, 13, 540–546 CrossRef CAS.
- X. Wang, S. Wang, J. Lv, S. Shao, L. Wang, X. Jing and F. Wang, Chem. Sci., 2019, 10, 2915–2923 RSC.
- J. Lu, Y. Zheng and J. Zhang, RSC Adv., 2015, 5, 18588–18592 RSC.
- L. Gan, Z. Xu, Z. Wang, B. Li, W. Li, X. Cai, K. Liu, Q. Liang and S. J. Su, Adv. Funct. Mater., 2019, 29, 1808088 Search PubMed.
- X. Liang, Z. L. Tu and Y. X. Zheng, Chem. – Eur. J., 2019, 25, 5623–5642 CAS.
- T. Hatakeyama, K. Shiren, K. Nakajima, S. Nomura, S. Nakatsuka, K. Kinoshita, J. Ni, Y. Ono and T. Ikuta, Adv. Mater., 2016, 28, 2777–2781 CrossRef CAS.
- X. Liang, Z. P. Yan, H. B. Han, Z. G. Wu, Y. X. Zheng, H. Meng, J. L. Zuo and W. Huang, Angew. Chem., Int. Ed., 2018, 57, 11316–11320 CrossRef CAS.
- S. Madayanad Suresh, D. Hall, D. Beljonne, Y. Olivier and E. Zysman-Colman, Adv. Funct. Mater., 2020, 1908677 CrossRef CAS.
- K. M. O. Nuyken, S. Jungermann, V. Wiederhirn and E. Bacher, Monatsh. Chem., 2006, 137, 811–824 CrossRef.
- M. Singh, H. M. Haverinen, P. Dhagat and G. E. Jabbour, Adv. Mater., 2010, 22, 673–685 CrossRef CAS.
-
D. V. A. Arjona-Esteban, Highly Efficient OLEDs: Materials Based on Thermally Activated Delayed Fluorescence, 2018, pp. 543–572 Search PubMed.
- L. Duan, L. Hou, T. W. Lee, J. Qiao, D. Zhang, G. Dong, L. Wang and Y. Qiu, J. Mater. Chem., 2010, 20, 6392–6407 RSC.
- T. Huang, W. Jiang and L. Duan, J. Mater. Chem. C, 2018, 6, 5577–5596 RSC.
- Y. Zou, S. Gong, G. Xie and C. Yang, Adv. Opt. Mater., 2018, 6, 1–25 RSC.
- J. L. Wu, Y. T. Lee, C. T. Chen and C. T. Chen, J. Chin. Chem. Soc., 2018, 65, 87–106 CrossRef CAS.
- C. Li, Z. Ren, X. Sun, H. Li and S. Yan, Macromolecules, 2019, 52, 2296–2303 CrossRef CAS.
- J. Hu, Q. Li, S. Shao, L. Wang, X. Jing and F. Wang, Adv. Opt. Mater., 2020, 1902100 CrossRef CAS.
- J. Rao, X. Liu, X. Li, L. Yang, L. Zhao, S. Wang, J. Ding and L. Wang, Angew. Chem., Int. Ed., 2020, 59, 1320–1326 CrossRef CAS.
- A. Menon, H. Dong, Z. I. Niazimbetova, L. J. Rothberg and M. E. Galvin, Chem. Mater., 2002, 14, 3668–3675 CrossRef CAS.
- Q. Wei, Z. Ge and B. Voit, Macromol. Rapid Commun., 2019, 40, 1800570 CrossRef.
- B. Zhang and Y. Cheng, Chem. Rec., 2019, 19, 1624–1643 CrossRef CAS.
- T. Jiang, Y. Liu, Z. Ren and S. Yan, Polym. Chem., 2020, 11, 1555–1571 RSC.
- Y. J. Cho, K. S. Yook and J. Y. Lee, Adv. Mater., 2014, 26, 6642–6646 CrossRef CAS.
- X. L. Chen, J. H. Jia, R. Yu, J. Z. Liao, M. X. Yang and C. Z. Lu, Angew. Chem., Int. Ed., 2017, 56, 15006–15009 CrossRef CAS.
- Y. Wada, K. Shizu, S. Kubo, K. Suzuki, H. Tanaka, C. Adachi and H. Kaji, Appl. Phys. Lett., 2015, 107, 183303 CrossRef.
- Y. Wada, S. Kubo and H. Kaji, Adv. Mater., 2018, 30, 1705641 CrossRef.
- J. Lee, K. Shizu, H. Tanaka, H. Nomura, T. Yasuda and C. Adachi, J. Mater. Chem. C, 2013, 1, 4599–4604 RSC.
- Z. Yang, Z. Mao, Z. Xie, Y. Zhang, S. Liu, J. Zhao, J. Xu, Z. Chi and M. P. Aldred, Chem. Soc. Rev., 2017, 46, 915–1016 RSC.
- Y. Im, M. Kim, Y. J. Cho, J. A. Seo, K. S. Yook and J. Y. Lee, Chem. Mater., 2017, 29, 1946–1963 CrossRef CAS.
- O. E. K. A. Hofmann, Ber. Dtsch. Chem. Ges., 1911, 44, 2713–2717 CrossRef.
- O. E. K. A. Hofmann, Ber. Dtsch. Chem. Ges., 1912, 45, 2731–2740 CrossRef.
- D. W. Kaiser, G. A. Peters and V. P. Wystrach, J. Org. Chem., 1953, 18, 1610–1615 CrossRef CAS.
- R. Huisgen, Chem. Ber., 1961, 94, 1555–1562 CrossRef CAS.
- R. Cristiano, H. Gallardo, A. J. Bortoluzzi, I. H. Bechtold, C. E. M. Campos and R. L. Longo, Chem. Commun., 2008, 5134–5136 RSC.
- S. K. Pathak, Y. Xiang, M. Huang, T. Huang, X. Cao, H. Liu, G. Xie and C. Yang, RSC Adv., 2020, 10, 15523–15529 RSC.
- S. Wang, X. Wang, K. H. Lee, S. Liu, J. Y. Lee, W. Zhu and Y. Wang, Dyes Pigm., 2020, 182, 108589 CrossRef CAS.
- A. G. Dal-Bó, G. G. L. Cisneros, R. Cercena, J. Mendes, L. M. da Silveira, E. Zapp, K. G. Domiciano, R. da Costa Duarte, F. S. Rodembusch and T. E. A. Frizon, Dyes Pigm., 2016, 135, 49–56 CrossRef.
- S. Grimme, Chem. Phys. Lett., 1996, 259, 128–137 CrossRef CAS.
- V. Adamo and C. Barone, J. Chem. Phys., 1999, 110, 6158–6170 CrossRef.
- T. H. Dunning, J. Chem. Phys., 1989, 90, 1007–1023 CrossRef CAS.
- P. L. Santos, J. S. Ward, P. Data, A. S. Batsanov, M. R. Bryce, F. B. Dias and A. P. Monkman, J. Mater. Chem. C, 2016, 4, 3815–3824 RSC.
- T. Hosokai, H. Matsuzaki, H. Nakanotani, K. Tokumaru, T. Tsutsui, A. Furube, K. Nasu, H. Nomura, M. Yahiro and C. Adachi, Sci. Adv., 2017, 3, 1603282 CrossRef.
- H. Noda, H. Nakanotani and C. Adachi, Sci. Adv., 2018, 4, 1–8 Search PubMed.
- P. K. Samanta, D. Kim, V. Coropceanu and J. L. Brédas, J. Am. Chem. Soc., 2017, 139, 4042–4051 CrossRef CAS.
- N. Sharma, E. Spuling, C. M. Mattern, W. Li, O. Fuhr, Y. Tsuchiya, C. Adachi, S. Bräse, I. D. W. Samuel and E. Zysman-Colman, Chem. Sci., 2019, 10, 6689–6696 RSC.
- D. Chen, P. Rajamalli, F. Tenopala-Carmona, C. L. Carpenter-Warren, D. B. Cordes, C. M. Keum, A. M. Z. Slawin, M. C. Gather and E. Zysman-Colman, Adv. Opt. Mater., 2020, 8, 1901283 CrossRef CAS.
- P. L. Dos Santos, D. Chen, P. Rajamalli, T. Matulaitis, D. B. Cordes, A. M. Z. Slawin, D. Jacquemin, E. Zysman-Colman and I. D. W. Samuel, ACS Appl. Mater. Interfaces, 2019, 11, 45171–45179 CrossRef CAS.
- Z. Li, W. Li, C. Keum, E. Archer, B. Zhao, A. M. Z. Slawin, W. Huang, M. C. Gather, I. D. W. Samuel and E. Zysman-Colman, J. Phys. Chem. C, 2019, 123, 24772–24785 CrossRef CAS.
- M. Y. Wong, S. Krotkus, G. Copley, W. Li, C. Murawski, D. Hall, G. J. Hedley, M. Jaricot, D. B. Cordes, A. M. Z. Slawin, Y. Olivier, D. Beljonne, L. Muccioli, M. Moral, J. C. Sancho-Garcia, M. C. Gather, I. D. W. Samuel and E. Zysman-Colman, ACS Appl. Mater. Interfaces, 2018, 10, 33360–33372 CrossRef CAS.
- G. A. Crosby and J. N. Demas, J. Phys. Chem., 1971, 75, 991–1024 CrossRef CAS.
Footnotes |
† The research data supporting this publication can be accessed at https://doi.org/10.17630/586490c1-7c86-48aa-ad7c-f90809626afb. |
‡ Electronic supplementary information (ESI) available. See DOI: 10.1039/d0ma00659a |
§ Equal contributions. |
|
This journal is © The Royal Society of Chemistry 2020 |
Click here to see how this site uses Cookies. View our privacy policy here.