DOI:
10.1039/D4NJ01395F
(Paper)
New J. Chem., 2024,
48, 9429-9441
Ethylenediamine modified ZnAlCu-LDO with high adsorption for phosphate†
Received
25th March 2024
, Accepted 3rd May 2024
First published on 14th May 2024
Abstract
Layered double hydroxides (LDHs) have emerged as an efficient and easily prepared class of anion adsorbents, showcasing remarkable potential in the adsorption and recovery of phosphate from wastewater, notably due to their unique “memory effect” post-calcination. This study focuses on the enhancement of phosphate adsorption capabilities of ZnAl layered double hydroxides (ZnAl-LDHs), which are particularly known for their high phosphate affinity, through the incorporation of copper and subsequent modification with ethylenediamine. The resultant ZnAlCu layered trimetallic oxide (ZnAlCu-1%-LDO), post-calcination, was modified with ethylenediamine to produce a novel inorganic metal/organic amine composite adsorbent, designated as ZACen-0.5. Characterization of ZACen-0.5 demonstrated an improvement in surface hydrophilicity, alongside a notable alteration in the adsorbent's skeletal structure, which manifested as degraded granular layers, an increased pore volume, and an enhanced specific surface area. The specific surface area of ZACen-0.5 was found to be 104.61 m2 g−1, marking a 51% increase compared to ZnAlCu-1%-LDO (69.27 m2 g−1). The adsorption capacity of ZACen-0.5 for phosphate was significantly elevated to 271.00 mg P g−1, maintaining an efficiency of 88.2% over four regeneration cycles. The study elucidated the adsorption mechanism of ZACen-0.5, attributing it to monolayer and chemical adsorption processes, which involve electrostatic attraction, ligand exchange, and surface precipitation. In practical wastewater treatment, ZACen-0.5 achieved a phosphate removal efficiency of over 98% from wastewater with an initial phosphate concentration of 3.43 mg P L−1, reducing the phosphate level to 0.0531 mg P L−1, thereby surpassing the stringent first-level standard of GB 8978–1996 (0.5 mg P L−1). The findings of this research underscore the effectiveness of ethylenediamine modification of ZnAlCu-1%-LDO in significantly enhancing the phosphate adsorption efficiency of LDH-based materials, presenting a promising avenue for the development of advanced wastewater treatment technologies.
1. Introduction
Nowadays, phosphate finds extensive application across various domains of life, inevitably leading to the generation of wastewater with elevated phosphate concentrations. Furthermore, a large number of phosphates as cathode materials for lithium-ion batteries can be obtained by molten salt method.1 However, a large number of waste lithium-ion batteries also cause serious environmental pollution problems. Failure to adequately treat phosphate-containing wastewater can result in eutrophication of water bodies.2 To prevent the water quality from getting worse, reducing the concentration of phosphate in wastewater and developing effective means of phosphate recovery are of great significance. Therefore, various phosphate removal techniques have been devised, including adsorption, chemical precipitation, electrolysis, ion exchange, membrane separation, and biological treatment.3–8 However, many phosphate removal methods exhibit inherent limitations, including elevated costs and susceptibility to secondary pollution. In contrast, the adsorption technique enjoys widespread adoption due to its simplicity, cost-effectiveness, high removal efficiency, and absence of secondary pollutants.9,10
The adsorption method relies on the principle of phosphate removal, wherein phosphate ions undergo physical adsorption, ligand exchange, electrostatic attraction, and surface precipitation onto the surface of a solid adsorbent. Subsequently, the enriched phosphate is separated from the sewage through solid–liquid separation processes.11 The key to phosphate removal by adsorption is the creation of adsorbents with high efficiency, high adsorption capacity, and reusability. Ideally, an adsorbent should exhibit superior adsorption selectivity, rapid adsorption kinetics, stability, absence of secondary pollution, high recyclability efficiency, and economical raw material costs.12 In wastewater treatment, commonly used phosphate removal adsorbents include natural minerals (dolomite, zeolite, montmorillonite, etc.), carbon materials (activated carbon, biochar, etc.), and synthetic materials (metal–organic frameworks, metal oxides and metal hydroxides, etc.).13–18 While natural and carbonaceous materials offer cost-effectiveness, they often require combination with metals to enhance phosphate removal efficacy. Metal–organic framework materials, a widely employed phosphate removal adsorbent, boast large specific surface areas, yet suffer from expensive synthetic processes and potential release of toxic organic ligands during adsorption, posing risks of secondary pollution to the environment.19–21 Metal hydroxides, abundant and easily obtainable in nature, present a viable alternative. Their substantial positive charge facilitates strong adsorption of phosphate anions, rendering them frequently utilized as phosphate removal adsorbents.22
A novel kind of adsorption material is layered double hydroxides (LDHs), which are widely used in various neighborhoods,23–26 especially used to remove anionic pollutants due to their rich species, controllable interlayer spacing, positive charge of laminates, and negative charge between the layers.27–29 Upon calcination within the temperature range of 200–500 °C, the LDHs undergo a transformation where hydroxyl groups are gradually eliminated as water molecules, leading to an increase in specific surface area and pore volume. At these temperatures, the material becomes an amorphous metastable mixed solid oxide.30,31 This process results in the formation of relatively stable layered double metal oxides (LDOs) exhibiting enhanced alkalinity. There is a “memory effect” with the LDOs in this state, that is LDOs can be reformed to partially restore the layered structure of LDHs in the presence of anions in an aqueous solution.32 Using this property, it is beneficial to adsorb more phosphate.
It has been observed that the adsorption capacity of LDHs with different metal compositions to phosphate differs significantly. Among these, ZnAl layered double hydroxides (ZnAl-LDHs) generally exhibit superior phosphate adsorption capabilities compared to other hydrotalcite variants.33 The common LDHs flakes have a diameter of 0.5–2 μm and a thickness of 15–20 nm.34,35 To further improve the adsorption capacity of ZnAl-LDHs, previous studies predominantly focused on the partial substitution of M3+ for Al3+, while the use of M2+ to partially replace Zn2+ for phosphate removal is rare. Jahn–Teller effect is an electronic effect describing the geometric distortion of molecules and ions, which is related to some electronic configurations. It shows that any nonlinear molecule with a spatially degenerate electron ground state will undergo geometric distortion, thereby eliminating degeneracy and reducing energy. This distortion is usually observed in the octahedral complex, and the d-orbital theoretical electron number of Cu2+ is 9, which can cause the Jahn–Teller effect. Therefore, our research group has carried out relevant modification work, producing ZnAlCu layered trimetallic oxide (ZnAlCu-1%-LDO) to enhance the phosphorus removal efficiency of ZnAl hydrotalcite by utilizing the “Jahn–Teller effect” of Cu2+ and the “memory effect” of LDHs.36 Upon replacing a portion of Zn2+ with Cu2+ in ZnAl hydrotalcite-like layers, a fraction of the Zn–O octahedra transforms into elongated Cu–O octahedra, resulting in reduced layer regularity and crystallinity. Consequently, hydroxyl ligands on the layers become more susceptible to phosphate exchange. This deformation of the layers not only enlarges the interlayer space, facilitating easier penetration of phosphate ions, but also enhances surface irregularity, thereby augmenting specific surface area and significantly increasing phosphate adsorption sites.
Surface modification and enhancement of specific surface area are effective means to improve the adsorption capacity of ZnAlCu-1%-LDO. Recent investigations elucidate that the introduction of ethylenediamine onto the surfaces of transition metal substrates engenders novel surface states. Specifically, the incorporation of amino functionalities significantly enhances hydrophilicity, as documented in literature.37–39 In addition, the strong bonding between ethylenediamine and metal can destroy the unstable metal–organic ligand bonds in metal–organic frameworks (MOFs), increasing the specific surface area and making the surface rough. Vrtovec et al. experiment indicated that after ethylenediamine modification, the Cu-based metal organic framework (copper 1,3,5-benzenetricarboxylate, HKUST-1) crystal skeleton was partially degraded to form a microporous/mesoporous system, which improved the specific surface area and diffusion adsorption of HKUST-1 adsorbate.40 Other transition metal MOFs (Cu-BTTri, Ni-MOF-74, Co-MOF-74, Zn-MOF-74) also exhibit affinity towards ethylenediamine,41,42 substantially boosting their adsorption potential for diminutive gas molecules (such as CO, SO2, CO2etc.) Ethylenediamine can not only provide amino functional groups for the adsorbent, but also enhances its hydrophilicity. Even the metal–ligand link with modest binding force can be destroyed by the strong bonding impact of ethylenediamine and the metal on the surface of the adsorbent, thereby increasing the specific surface area and inducing surface roughening. Consequently, employing ethylenediamine-modified hydrotalcite as an adsorbent adeptly fulfills both criteria of amino modification and specific surface area augmentation, underscoring its utility in advanced adsorption applications.
In this study, we synthesized an inorganic-metal–organic amine composite adsorbent, denoted as ZACen-0.5, through the modification of ZnAlCu layered trimetallic oxide (ZnAlCu-1%-LDO), itself derived from the calcination of copper-doped ZnAl-LDHs, utilizing ethylenediamine as a modifying agent. The surface transition metals (Zn and Cu) within ZnAlCu-1%-LDO react with ethylenediamine, engendering novel surface conditions that significantly enhance the hydrophilicity of ZACen-0.5, thereby promoting its homogeneous dispersion in aqueous environments. Concurrently, the protonation of amino groups under acidic environments augments the surface positive charge on ZACen-0.5, improving its electrostatic interactions with phosphate ions. Most importantly, ethylenediamine modification leads to the partial decomposition of the hydrotalcite framework into a granular layer structure, resulting in an expanded specific surface area and pore volume. This transformation escalates the availability of adsorption sites and facilitates the diffusion adsorption of phosphate ions. Comparative analysis reveals that ZACen-0.5 exhibits superior adsorption capacity relative to its precursor, ZnAlCu-1%-LDO, underscoring the effectiveness of ethylenediamine modification in enhancing adsorbent performance.
2. Experimental section
2.1. Materials and characterization
The relevant materials and instruments used in this experiment are shown in the ESI† Text S2.
2.2. Synthesis of ZACen-0.5 adsorbents
CuZnAl-1%-LDO was modified by ethylenediamine using a solvothermal method. The detailed synthesis process of CuZnAl-1%-LDO is described in the ESI† Text S2, with Fig. S1 (ESI†) illustrating the preparation process. Firstly, 200 mg of ZnAlCu-1%-LDO was introduced into 20 mL of ethylenediamine. Following ultrasonic dispersion to ensure homogeneity, the mixture was subjected to heating at 120 °C for 30 minutes within an oil bath, with continuous agitation provided by magnetic stirring. Subsequent to the solvothermal treatment, the mixture was allowed to cool to ambient temperature and then centrifuged at 6000 rpm to facilitate the collection of the precipitate. The precipitate was washed with ethanol to remove residual ethylenediamine adhering to the adsorbent's surface. The final step involved drying the washed precipitate at 60 °C in a forced-air drying oven, yielding the ethylenediamine-modified ZnAlCu-1%-LDO, designated as ZACen-0.5.
2.3. Adsorption phosphate removal experiment
2.3.1. Adsorption phosphate removal.
A mixed solution of 20 mg adsorbent and phosphate solution (200 mg P L−1, 50 mL, pH = 3.5) was prepared. This mixture was subjected to oscillation at 25 °C for a duration of 24 hours, subsequently undergoing vacuum assisted filtration (The filtration methods in this paper are all vacuum assisted filtration.) through a 0.22 μm filter membrane and circulating water pump. The filtrate was diluted and determined by ammonium molybdate spectrophotometry. The absorbance was measured at 710 nm by an ultraviolet-visible spectrophotometer, and the phosphate standard curve was used to determine the amount of phosphate in the adsorbed solution. Detailed theoretical calculations supporting this analysis are provided in Text S1 of the ESI.†
2.3.2. Study on adsorption isotherm.
A 50 mL of phosphate solution, with an initial concentration ranging from 5 to 1000 mg P L−1 and adjusted to a pH of 3.5, add to a conical flask placed in a frozen thermostatic oscillator, and subjected to oscillatory adsorption at 25 °C for 24 hours. (The oscillatory adsorption in this paper is all carried out by using a frozen thermostatic oscillator.) Subsequent filtration was performed utilizing a 0.22 μm filter membrane. The adsorption capacity was calculated following the determination of phosphate concentration in the filtrate.
2.3.3. Study on adsorption kinetics.
The adsorption kinetics experiments were carried out at a solution temperature of 25 °C, a solution pH of 3.5 and phosphate concentrations of 50, 100 and 200 mg P L−1, respectively. The dosage of the adsorbent was 25 mg. During the adsorption period, 0.5 mL of solution were periodically extracted at predetermined intervals. These samples were analyzed to determine the phosphate concentration, which facilitated the calculation of the adsorption capacity.
2.3.4. Impact of starting solution pH on phosphate adsorption performance of adsorbent.
The impact of initial solution pH on the phosphate adsorption performance of the adsorbent was performed under a phosphate concentration of 200 mg P L−1, solution temperature of 25 °C, and a starting pH range of 3.5 to 9. The dosage of the adsorbent was 0.4 g L−1. The mixture was subjected to oscillation at a speed of 1600 rpm for a duration of 24 hours, followed by filtration through a 0.22 μm filter membrane. The adsorption capacity was determined after measuring the phosphate concentration in the filtrate.
2.3.5. Impact of adsorption temperature on phosphate adsorption performance of adsorbent.
The impact of adsorption temperature on the phosphate adsorption performance of the adsorbent was studied under a phosphate concentration of 200 mg P L−1, solution pH of 3.5 and temperature range of 20 to 40 °C. The dosage of the adsorbent was 0.4 g L−1. The dispersion underwent shaking at 1600 rpm for 24 hours, after which it was filtered through a 0.22 μm filter membrane. Subsequent to the filtration process, the adsorption capacity was calculated based on the measured phosphate concentration in the filtrate.
2.3.6. Impact of coexisting anions on phosphate adsorption performance of adsorbents.
The impact of coexisting anions (NO3−, CO32−, SO42−, Cl−) on the phosphate adsorption performance of adsorbents was carried out at phosphate concentration of 200 mg P L−1 and solution pH of 3.5. The dosage of the adsorbent was 0.4 g L−1. The dispersion was agitated at 25 °C for 24 hours and subsequently filtered through a 0.22 μm filter membrane. The adsorption capacity was determined after measuring the phosphate concentration in the filtrate.
2.3.7. Impact of adsorbent dosage on phosphate adsorption performance.
The adsorbents (ranging from 5 to 50 mg) were introduced into a phosphate solution (50 mL, 200 mg P L−1, pH = 3.5) to create a dispersion. Subsequently, the adsorption capacity was determined after measuring the phosphate concentration in the filtrate.
2.3.8. Regeneration experiment.
The regeneration experiment was studied under phosphate concentration of 200 mg P L−1, solution pH of 3.5 and temperature of 25 °C. The dosage of the adsorbent was 0.4 g L−1. The dispersion underwent oscillation at 1600 rpm for 6 hours, followed by filtration through a 0.22 μm filter membrane to separate the adsorbent from the filtrate. The phosphate-enriched adsorbent was then subjected to elution with 0.05 mol L−1 NaOH solution, neutralized with deionized water, dried at 60 °C, and subsequently calcined in a muffle furnace for 2 hours at 350 °C.
2.3.9. Performance of the adsorbent in removing phosphate from actual wastewater.
The real wastewater sample was collected from the vicinity of the campus area. Initially, the suspended matter in the wastewater was removed by filtration through a 0.22 μm filter membrane. The resulting treated wastewater exhibited a pH of 6.3 and a phosphate content of 3.43 mg P L−1. Subsequently, 100 mL of the treated wastewater was combined with 0.1 g of the adsorbent to form a dispersion. This dispersion underwent agitation for 24 hours at 25 °C, after which it was filtered through a 0.22 μm filter membrane. The adsorption capacity was determined by measuring the phosphate concentration in the filtrate.
3. Results and discussion
3.1. Characterization
3.1.1. SEM, TEM and EDS analysis.
The characterization of ZACen-0.5 by SEM, TEM and EDS is shown in Fig. 1. Generally, LDHs will be exfoliated after ultrasonic treatment, mechanical oscillation or stirring in aqueous solutions such as formamide, NaOH and urea. However, the calcined ZnAlCu-1%-LDO and modified ZACen-0.5 did not exfoliate the layers, and the morphology of the ZACen-0.5 changed significantly to granular, as shown in Fig. S2 (ESI†). At the same magnification, the CuZnAl-1%-LDO of Fig. S2a (ESI†) exhibits a plate-like morphology with a diameter of 2–4 μm and the surface becomes wrinkled after high temperature calcination. It can be seen from Fig. S2b (ESI†) that the ZACen-0.5 laminates obtained after ethylenediamine modification become granular. In Fig. 1b, the localized magnification of Fig. 1a reveals a granular structure replacing the previously observed thin plate structure, indicative of the degradation of the layered hydrotalcite structure induced by ethylenediamine. The particle size distribution of ZACen-0.5 ranges from approximately 20 to 70 nm, it shows the presence of 24 nm and 65 nm particles in Fig. 1b. The results coinciding with an increase in specific surface area and pore volume, thus enhancing its capacity for adsorbing other substances. Fig. 1c provides a clearer depiction of numerous particles congregating at the periphery of the adsorbent. Fig. 1d displays the SEM image of ZACen-0.5 alongside the corresponding EDS map, demonstrating a uniform distribution of elements, including O, Zn, Al, Cu, and N. The presence of N, inferred from the EDS analysis, indirectly confirms the successful modification with ethylenediamine.
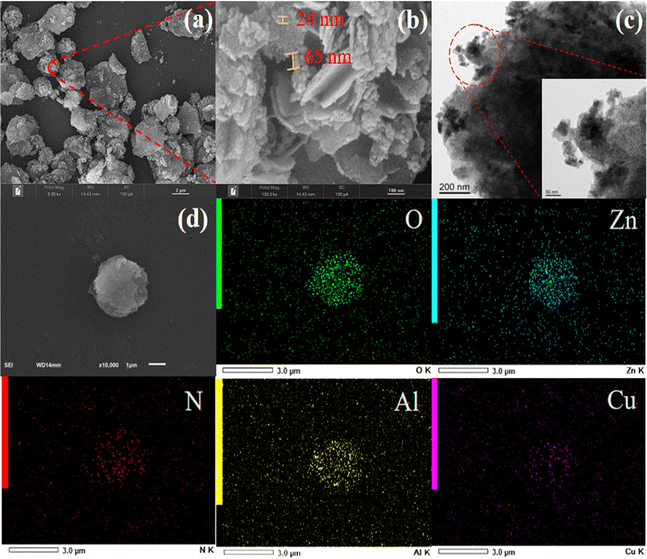 |
| Fig. 1 (a) SEM image of ZACen-0.5; (b) local amplification image of (a); (c) TEM images of ZACen-0.5; (d) SEM and EDS of ZACen-0.5. | |
3.1.2. XRD analysis.
The X-ray diffraction (XRD) comparison between ZnAlCu-1%-LDO and ZACen-0.5 is illustrated in Fig. 2. The ZnAlCu-1%-LDO obtained by calcination is a metastable mixed metal oxide, and the crystal form is mainly ZnO (PDF#79-0206). The diffraction peaks of CuO and Al2O3 do not appear, indicating that they exist in an amorphous state. In addition, it is observed that neither the position nor the appearance of diffraction peaks in the adsorbent treated with ethylenediamine has been altered, suggesting that the ethylenediamine modification has not induced phase changes in the adsorbent. However, there is an increase in the intensity of diffraction peaks, which could be attributed to the alkaline nature of the ethylenediamine solution, promoting the growth of ZnO crystals within the adsorbent in an alkaline environment.
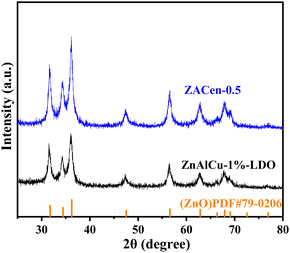 |
| Fig. 2 XRD patterns of ZnAlCu-1%-LDO and ZACen-0.5. | |
3.1.3. XPS analysis.
X-ray photoelectron spectroscopy (XPS) was employed to ascertain the elemental composition of the adsorbent. Fig. 3a displays the XPS full spectrum of both ZnAlCu-1%-LDO and ZACen-0.5. ZnAlCu-1%-LDO primarily comprises elements such as Zn, Al, O, C, and Cu. Following modification with ethylenediamine, ZACen-0.5 exhibits an additional peak corresponding to N 1s at 399.7 eV, confirming the successful modification with ethylenediamine. In order to better understand the surface chemical state of the adsorbent subsequent to ethylenediamine modification, a N 1s peak fitting study of ZACen-0.5 was performed. Fig. 3b shows the spectrum of ZACen-0.5 displays four distinct peaks in the N 1s region. The binding energies of 399.7 eV and 398.4 eV correspond to the peaks of –NH2 and –C–NH2 on ethylenediamine, respectively.43,44 The peak of –N–Cu is associated with the binding energy of 399.4 eV,45 while the peak of –N–Zn may be associated with the binding energy of 400.3 eV, which proves that ethylenediamine is coordinated with the transition metals Cu and Zn.
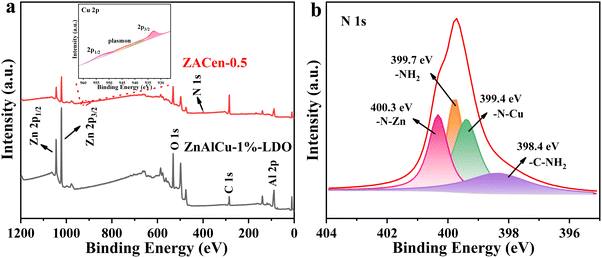 |
| Fig. 3 (a) XPS spectra of ZnAlCu-1%-LDO and ZACen-0.5; (b) N 1s spectrum of ZACen-0.5. | |
3.1.4. Zeta potential analysis.
The Zeta potential value of ZACen-0.5 surface was measured by Zeta potentiometer. As depicted in Fig. S3 (ESI†), the surface potential of ZACen-0.5 shifts as the solution pH shifts, and its surface zero charge point (PZC) is 9.90. Compared with ZnAlCu-1%-LDO, ZACen-0.5 has a higher positive charge when pH < 5.7. When the solution pH < pHPZC, the adsorbent has a positive surface potential. At pH values lower than the PZC, the adsorbent surface demonstrates positive potential. As the solution pH decreases, the concentration of H+ ions in the solution increases, resulting in a greater abundance of positive charges on the adsorbent surface. When the solution pH > pHPZC, the surface potential of the adsorbent is negative. In the range of solution pH < 9.90, the surface potential of ZACen-0.5 is positive, indicating its capability to adsorb negatively charged phosphate anions through electrostatic attraction across a wide range of solution pH values.
3.1.5. BET analysis.
The isotherms of nitrogen adsorption and desorption for ZnAlCu-1%-LDO and ZACen-0.5 are shown in Fig. S4 (ESI†). According to the classification of International Pure and Applied Chemistry (IUPAC), both ZnAlCu-1%-LDO and ZACen-0.5 feature the H3 hysteresis loop, indicative of type III isotherms.46 This kind of hysteresis line is normally derived from the slit pores created by the lamellar structure of the aggregates. Table S1 (ESI†) compares the specific surface areas of ZACen-0.5 and ZnAlCu-1%-LDO. Notably, the specific surface area of ZACen-0.5, derived from ethylenediamine-modified ZnAlCu-1%-LDO, has increased from 69.27 m2 g−1 to 104.61 m2 g−1. This enhancement can be attributed to the partial degradation of the adsorbent's layer structure, resulting in granulation. The stacking between particles resulted in larger pore volume (0.1392 cm3 g−1 to 0.2102 cm3 g−1) and smaller pore size (28.71 nm to 25.91 nm). These changes indicate that ZACen-0.5 has more mesoporous structure, which is helpful for the diffusion adsorption of phosphate.
3.1.6. FT-IR analysis.
The infrared spectra of ethylenediamine, ZnAlCu-1%-LDO and ZACen-0.5 are displayed in Fig. 4. For the infrared spectrum of ethylenediamine, the absorption peaks at 3364 cm−1, 3289 cm−1 and 3187 cm−1 are caused by –NH2 stretching vibration, while the absorption peaks at 2938 cm−1 and 2851 cm−1 correspond to the stretching vibration of –CH2. Additionally, the absorption peaks at 1591 cm−1 and 1165 cm−1 represent the shear vibration and torsional vibration of –NH2, respectively. The absorption peaks at 1290 cm−1, 1350 cm−1 and 1460 cm−1 are caused by –CH2 torsional vibration, swing and shear vibration, while the absorption peaks at 1090 cm−1 and 1045 cm−1 are caused by C–C and C–N stretching vibration, respectively. Furthermore, the absorption peaks at 893 cm−1 and 816 cm−1 correspond to –CH2 and –NH2 shaking vibrations.47
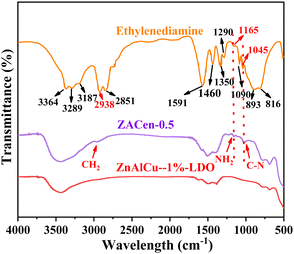 |
| Fig. 4 Infrared spectra of ethylenediamine, ZnAlCu-1%-LDO and ZACen-0.5. | |
Compared with ZnAlCu-1%-LDO, the infrared spectrum of ZACen-0.5 exhibits additional absorption peaks at 2961 cm−1, 1165 cm−1 and 1045 cm−1. Among these, the absorption peak at 2961 cm−1 is attributed to the stretching vibration of –CH2 groups, which shifts to higher frequencies compared to the stretching vibration frequency of –CH2 in ethylenediamine. The stretching vibration of C–N is represented by the absorption peaks at 1045 cm−1 and 1165 cm−1, respectively, which are associated with ethylenediamine, indicating that ethylenediamine has been successfully modified on the ZACen-0.5 adsorbent. Furthermore, upon modification with ethylenediamine, the absorption bands in the regions of 3600–3100 cm−1, 1700–1300 cm−1, and 900–600 cm−1 exhibit broadening, possibly due to the overlap of infrared absorption peaks from the ethylenediamine group with the original infrared absorption peaks of the adsorbent.
3.1.7. TG analysis.
Thermal stability of the ZACen-0.5 adsorbent was examined by using TG-DTG analysis. As shown in Fig. S5 (ESI†), there are four endothermic peaks approximately 65.8 °C, 154.2 °C, 298.5 °C and 387.6 °C on the DTG curve between 30 and 800 °C. Because ZACen-0.5 adsorbent was calcined at 350 °C prior to ethylenediamine modification, its thermal stability was relatively stable below 350 °C. Therefore, the first three endothermic peaks can be attributed to the thermal decomposition of ethylenediamine and the thermal removal of water molecules in the air adsorbed on the adsorbent surface during storage, with a corresponding weight loss rate of approximately 8.96%. Subsequently, within the temperature range of 350–800 °C, a weight loss rate of about 2.0% is observed, possibly attributed to the gradual removal of impurities during the sintering process, leading to the transformation of zinc aluminum copper oxide into a spinel structure with higher density.48
3.1.8. Hydrophilicity analysis.
If the adsorbent has good hydrophilicity, it can be fully dispersed in aqueous solution, which is more conducive to adsorption other substances. Therefore, the hydrophilicity of ZACen-0.5 surface was studied by contact angle meter, and the results as depicted in Fig. S6 (ESI†). Comparing Fig. S6a and b (ESI†), one can find that the water contact angle of the ZACen-0.5 decrease from 52.87° to 18.84° following ethylenediamine modification. This reduction in contact angle can be attributed to the surface modification by ethylenediamine, which introduces amino groups onto the surface of the adsorbent, enhancing its hydrophilicity.
3.2. Research on adsorption
3.2.1. The impact of reaction time on phosphate adsorption performance of ZACen-0.5.
As can be seen from Fig. 5, without the ethylenediamine modification, the adsorbent had a 199.28 mg P g−1 phosphate adsorption capacity. After reacting with ethylenediamine for 0.5 hour, the phosphate adsorption capacity of ZACen-0.5 increased by 11% to 221.2 mg P g−1. This enhancement can be attributed to several factors elucidated through SEM, BET, and Zeta potential analyses. Specifically, ethylenediamine modification partially degrades the layered structure of the adsorbent, leading to increased porosity and thereby augmenting the number of adsorption sites due to the expanded specific surface area. Moreover, the modification of amino groups renders the surface of the adsorbent more hydrophilic, enhancing its dispersibility in water. The adsorption capacity decreases when the reaction time is prolonged. This phenomenon can be attributed to the progressive alkalinity of the ethylenediamine solution, which etches small pores on the adsorbent into larger ones, consequently reducing the available adsorption sites.
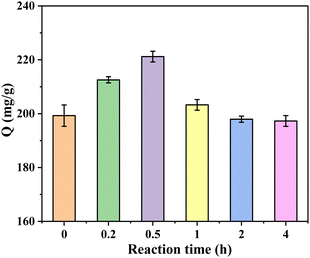 |
| Fig. 5 The impact of different reaction time on the phosphate adsorption by ZACen-0.5. | |
3.2.2. The impact of initial solution pH on the phosphate adsorption performance of ZACen-0.5.
The initial solution pH has a significant impact on the adsorption of phosphate by ZACen-0.5. The adsorption performance of ZACen-0.5 is more stable at pH = 3–6, as depicted in Fig. 6a, then decreases sharply when pH > 6. The following three factors contribute to the variation in adsorption capacity with respect to solution pH:
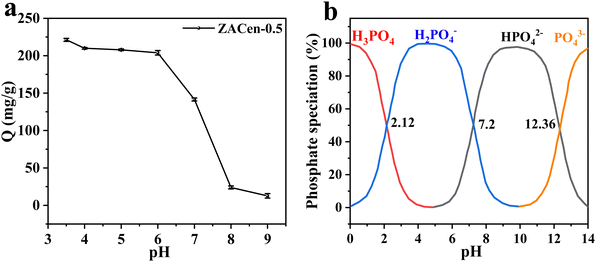 |
| Fig. 6 (a) The impact of initial pH value on phosphate adsorption performance of ZACen-0.5; (b) the existence forms of phosphate under different pH values. | |
(1) Phosphate exists in different forms depending on the pH of the solution. According to Fig. 6b, the primary form of phosphate was H2PO4− in a pH range of 3.5 to 6,49 and H2PO4− was easier to adsorb than HPO42− due to lower adsorption free energy.50 When pH > 6, H2PO4− gradually transformed into HPO42−, resulting in decreased adsorption capacity.
(2) The Zeta potential of the adsorbent is different with the different pH of the solution. According to Zeta potential analysis, the pHPZC of ZACen-0.5 is 9.90. When pH < 9.90, ZACen-0.5 has a positively charged surface that can attract negatively charged phosphate ions via electrostatic interaction. However, as the solution pH increases, the positive charge on the surface diminishes, leading to reduced phosphate ion adsorption capacity.
(3) The pH of the solution affected the concentration of OH−. The concentration of OH− increased as the solution pH increased. If the pH is greater than 6, the adsorption capacity of ZACen-0.5 to phosphate sharply reduced. This may be attributed to OH− and phosphate competitive adsorption on the surface of ZACen-0.5.
3.2.3. Thermodynamics of adsorption research.
The change of adsorption capacity of ZACen-0.5 at different adsorption temperatures is shown in Fig. S7 (ESI†). Fig. S7a (ESI†) demonstrates that the adsorption capability of phosphate ions diminishes as temperature rises. Because the reaction was exothermic, the increase of temperature made the adsorption equilibrium move towards the desorption direction, which prevented the adsorption of phosphate.
The thermodynamic properties of ZACen-0.5 were determined by three thermodynamic data: ΔS, ΔH and ΔG. Through the van't Hoff plot of ln(qe/Ce) to 1/T, Fig. S7b (ESI†) can be obtained. According to the slope and intercept of the fitted line, it is possible to calculate ΔH and ΔS and to derive ΔG.51
As determined by the thermodynamic parameter calculations in Table S2 (ESI†), ΔG < 0, and with a rise in temperature, the |ΔG| value drops, indicating that the adsorption process is spontaneous and the temperature rise is not conducive to the reaction. ΔH < 0 signifies an exothermic reaction during the adsorption process, and adsorption is not facilitated by raising the temperature.52 ΔS < 0, showing that solid–liquid interface confusion increases, which is not conducive to adsorption.53
3.2.4. Study on adsorption kinetics.
The relationship between the adsorption time and the capacity of ZACen-0.5 to phosphate, as can be seen from Fig. 7. The adsorption capacity quickly increased during the initial stages of adsorption. The adsorption rate slowed down with the increase in time and gradually tended to balance. There are likely many adsorption sites present on the surface of the adsorbent, which could account for the initial high adsorption rate, and because the initial solution contains the most phosphate, the adsorption driving power is considerable, which can be quickly adsorbed with phosphate. The adsorption rate lowered with the increase in adsorption time, which was attributed to the adsorption of a large amount of phosphate on the surface of the adsorbent increasing as time went on. ZACen-0.5 showed an increase in surface electronegativity, and the effective adsorption sites decreased. Meanwhile, the adsorption rate was also impacted by the drop in phosphate concentration in the solution.
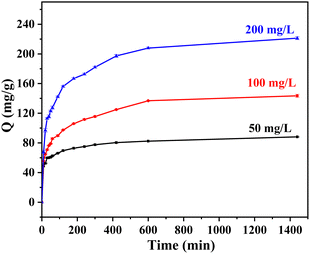 |
| Fig. 7 Impact of adsorption time on phosphate adsorption performance of ZACen-0.5. | |
Fig. 8 displays the fitting curve for the adsorption kinetics. The adsorption process was fitted by pseudo-first-order and pseudo-second-order kinetic equations, respectively. The fitting parameters are depicted in Table S3 (ESI†). Compared with the pseudo-first-order kinetic fitting curve, the R2 value of the pseudo-second-order kinetic fitting curve is closer to 1, and the theoretical adsorption capacity is closer to the actual adsorption capacity. Therefore, the phosphate adsorption by ZACen-0.5 is better described by the pseudo-second-order kinetic model, indicating the main role in the adsorption process is chemical adsorption.
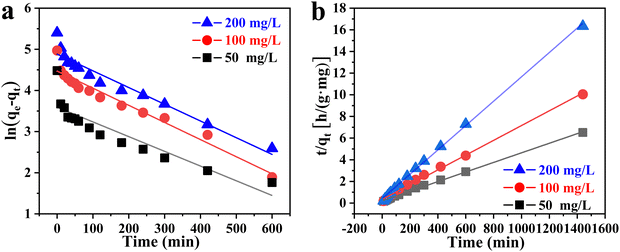 |
| Fig. 8 Kinetic model: (a) pseudo-first-order kinetic equation curve; (b) pseudo-second-order kinetic equation curve. | |
3.2.5. Study on adsorption isotherm.
Important information about the adsorption mechanism can be obtained from the adsorption isotherm. It can be seen from Fig. 9 that the removal rate increases as the phosphate concentration decreases, and when the phosphate concentration is 5 mg P L−1, the removal rate can reach 97.59%, and the residual phosphate concentration is only 0.1205 mg P L−1. When the initial concentration of phosphate increased, the probability of collision between ZACen-0.5 and phosphate increased, and the adsorption sites were more utilized, leading to an increase of the adsorption capacity of ZACen-0.5.
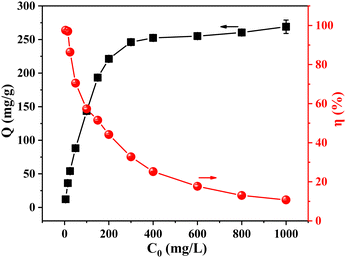 |
| Fig. 9 Effect of initial phosphorus concentration on adsorption effect. | |
Fig. S8 and Table S4 (ESI†) are the fitting findings for the Langmuir model and the Freundlich model, respectively. Compared to the R2 value of the Freundlich model (0.9538), the R2 value of the Langmuir model was 0.9985, indicating that the adsorption process of the adsorbent to phosphate was more in line with the Langmuir model, and monolayer adsorption was the primary type of adsorption that occurred. The saturated adsorption capacity, which was determined to be 271.0 mg P g−1, was comparable to the adsorption capacity at C0 = 1000 mg P L−1 (269.03 mg P g−1). In addition, ZACen-0.5 showed superior adsorption capacity compared to reported adsorbents (Table S5, ESI†). The 1/n value obtained by Freundlich model fitting is 0.3345, which is between 0.1 and 0.5, indicating that ZACen-0.5 is easy to adsorb phosphate.54
3.2.6. The impact of coexisting anions on phosphate adsorption performance of ZACen-0.5.
The phosphate adsorption procedure can be influenced by the presence of other anions in actual water samples. Therefore, in this study, coexisting anions SO42−, CO32−, Cl−, and NO3− were chosen for studying their impact on the adsorption of phosphate by ZACen-0.5. As shown in Fig. S9 (ESI†), in the absence of additional anions, ZACen-0.5 had an adsorption capacity of 199.28 mg P g−1. The change in adsorption capacity was −0.9%, −2.7%, −5.4%, and −4.5% when SO42−, CO32−, Cl− and NO3− were introduced into the mixture, respectively. These results suggest that the phosphate adsorption by ZACen-0.5 remained unaffected by the presence of SO42− and CO32−. This phenomenon can be attributed to the failure of the memory effect of ZACen-0.5 following ethylenediamine modification, thereby preventing ions with greater negative charges from advantageously entering the interlayer of the adsorbent to reorganize the hydrotalcite structure. The memory effect failure can be observed from the SEM image of the middle plate structure collapse in Fig. 1. Furthermore, the impact of Cl− and NO3− on the adsorption of phosphate by ZACen-0.5 was minor, indicating that ethylenediamine modification may promote the adsorption of other monovalent anions to some extent. However, the decrease in the adsorption capacity of ZACen-0.5 for phosphate did not exceed 6%, suggesting the presence of strong anti-interference properties in ZACen-0.5.
3.2.7. Regeneration study.
Fig. 10 shows the regeneration study results of ZACen-0.5. As the number of regeneration cycles increased, the adsorption efficiency of ZACen-0.5 on phosphate gradually decreased. Notably, after ZACen-0.5 had been utilized for 2, 3, 4, and 5 cycles, the regeneration rates were remarkably high at 96%, 93%, 91% and 88%, respectively. These findings underscore the excellent regeneration capability of ZACen-0.5.
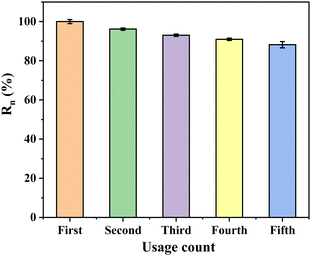 |
| Fig. 10 Regeneration rate of ZACen-0.5. | |
3.2.8. Performance of ZACen-0.5 in removing phosphate from actual wastewater.
To assess the practical application potential of ZACen-0.5, it was applied to wastewater that containing phosphate. With a wastewater pH of 6.3 and a phosphate concentration of 3.43 mg P L−1, ZACen-0.5 demonstrated exceptional performance. As depicted in Fig. S10 (ESI†), ZACen-0.5 achieved a remarkable phosphate removal rate of 98.45%, resulting in a residual phosphate concentration of only 0.0531 mg P L−1 in the treated wastewater. Notably, this concentration is significantly lower than the primary standard for phosphate emission in China (0.5 mg P L−1). These results underscore the excellent capability of ZACen-0.5 to effectively eliminate phosphate from real wastewater, highlighting its promising practical application prospects.
3.3. Adsorption mechanism
The alteration in the crystal structure of ZACen-0.5 before and after phosphate adsorption is shown in Fig. 11a. After adsorption of a low concentration (5 mg P L−1) of phosphate, ZACen-0.5 exhibited no restoration of the hydrotalcite structure, and its characteristic diffraction peak intensity decreased. Notably, there was an absence of characteristic diffraction peaks associated with LDHs, suggesting the loss of memory effect in ZACen-0.5 following ethylenediamine modification. Moreover, the distinctive diffraction peak related to ZnO associated with ZACen-0.5 diminished after adsorbing 200 mg P L−1 phosphate, while the appearance of the diffraction peak corresponding to Zn3(PO4)2·4H2O indicated the formation of a precipitate between the adsorbed phosphate ions and Zn2+ present on the surface of ZACen-0.5.
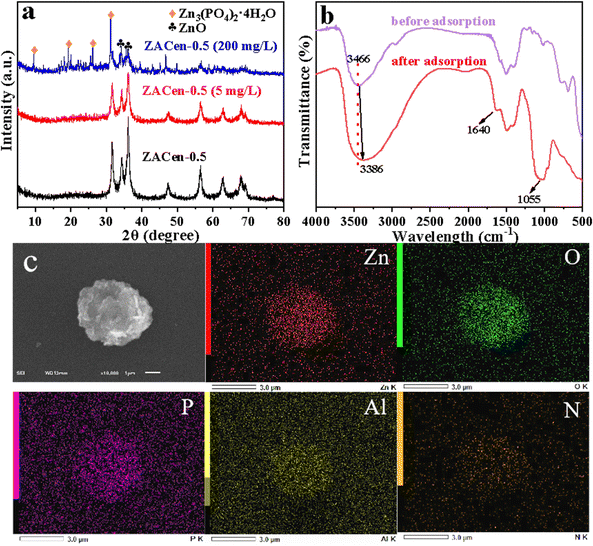 |
| Fig. 11 (a) XRD pattern of ZACen-0.5 before and after phosphate adsorption; (b) infrared spectra of ZACen-0.5 before and after phosphate adsorption; (c) SEM images and corresponding EDS images of ZACen-0.5 after phosphate adsorption. | |
The infrared spectrum of ZACen-0.5 is shown in Fig. 11b. After the adsorption of phosphate, ZACen-0.5 shows an obvious broad band at 1055 cm−1, corresponding to the PO43− stretched vibration band.55 The stretching vibration of –OH in crystal water and the bending vibration of –OH, respectively, are represented by the absorption peaks at 3466 cm−1 and 1640 cm−1.56 Notably, after phosphate adsorption, the intensity of both peaks enhances, indicating the formation of Zn3(PO4)2·4H2O precipitate. After the adsorption of phosphate, the absorption peak at 3466 cm−1 shifted to 3386 cm−1, indicating a ligand exchange between phosphate and the surface hydroxyl of ZACen-0.5.57Fig. 11c shows the SEM diagram and the corresponding EDS diagram after the adsorption of phosphate by ZACen-0.5. Obviously, compared with the SEM and EDS of the Fig. 1d, the results showed that the elemental composition of ZACen-0.5 adsorbent did not change before and after adsorption. In addition, there was a large amount of P element on the surface of ZACen-0.5 after adsorption, indicating that phosphate was adsorbed by ZACen-0.5.
The principle of surface precipitation as can be seen from Fig. S11 (ESI†). The adsorbed phosphate ions and the metal ions (such as Zn2+) that have leached from the hydrotalcite layer form a surface precipitate Zn3(PO4)2·4H2O:
| ZnO + 2H+ → Zn2+ + H2O | (1) |
| 2HPO42− + 3Zn2+ + 4H2O → Zn3(PO4)2·4H2O↓ + 2H+ | (2) |
| 2HPO4− + 3Zn2+ + 4H2O → Zn3(PO4)2·4H2O↓ + 4H+ | (3) |
The mechanism of phosphate adsorption by ZACen-0.5 was further explored by XPS. Fig. S12 (ESI†) is the XPS full spectrum of ZACen-0.5 before and after adsorption of phosphate. After adsorption, the characteristic peak of P 2p at 133.8 eV,54 indicating that ZACen-0.5 successfully adsorbed phosphate.
In addition, the adsorption mechanism was studied using Zeta potential analysis. Fig. 12a demonstrates that the point of zero charge (PZC) of ZACen-0.5 is 9.90. As the pH of the solution decreases, the surface positive charge of ZACen-0.5 intensifies, leading to enhanced electrostatic interaction with phosphate anions in the water. The zero charge point of ZACen-0.50 decreases to about 5.42 after phosphate adsorption. This alteration can be attributed to the protonation and deprotonation of hydroxyl groups on the surface of mixed metal oxides, which influence the point of zero charge.58 It's noteworthy that the chemical reaction of the surface charge of the adsorbent remains unaffected by the electrostatic interaction between the adsorbate and the adsorbent surface, thereby keeping the zero charge point unchanged.59 This observation strongly suggests the occurrence of chemical adsorption rather than pure electrostatic interaction between phosphate and ZACen-0.5.60
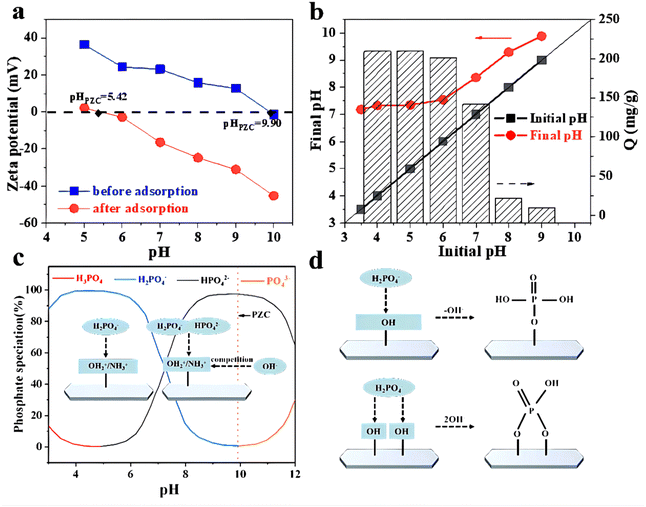 |
| Fig. 12 (a) Zeta potential changes before and after phosphate adsorption by ZACen-0.5; (b) the impact of starting pH on adsorption performance and pH changes before to and following adsorption; (c) diagram of the electrostatic interaction mechanism; (d) ligand exchange mechanism diagram. | |
From Fig. 12b, it can be seen that when the solution pH is between 3.5 and 9, ΔpH (defined as the final pH minus the starting pH) increases as the initial pH decreases, while the adsorption capacity of phosphate significantly rises. According to the Zeta potential test, the positive charge on the surface of the adsorbent increases as the pH decreases, leading to enhanced protonation of hydroxyl and amino groups. Consequently, the negatively charged phosphate experiences strong electrostatic attraction, thereby augmenting the adsorption capacity. Under weak acidic conditions, the main form of phosphate is H2PO4−. The electrostatic interaction makes H2PO4− move to the surface of the adsorbent, where it engages in monodentate ligand exchange with surface hydroxyl groups.61 This process ensures efficient utilization of adsorption sites, ultimately resulting in a substantial increase in the adsorption capacity. Theelectrostatic interaction and ligand exchange mechanism are shown in Fig. 12c and d. On the contrary, under weak alkaline conditions, the abundance of free hydroxyl groups in the initial solution competes with phosphate ions for adsorption. Consequently, the positive charge on the ZACen-0.5 surface diminishes, weakening the electrostatic attraction. Moreover, the proportion of HPO42− in the solution rises, leading to reduced utilization of adsorption sites due to bidentate ligand exchange with surface hydroxyl groups. Additionally, with increasing pH, hydroxyl ligands become more stable on the adsorbent surface, rendering them less prone to exchange. These factors collectively contribute to a significant reduction in adsorption capacity.
In summary, the adsorption mechanism of ZACen-0.5 mainly includes surface precipitation, electrostatic attraction, and ligand exchange. The processes of electrostatic interaction and ligand exchange are as follows:
Electrostatic interaction:
| –OH + H+ + H2PO4− → −OH2+---H2PO4− | (4) |
| –OH + H+ + HPO42− → −OH2+---HPO42− | (5) |
| –OH + H+ + OH− → −OH2+---OH− | (6) |
| –NH2 + H+ + H2PO4− → −NH3+---H2PO− | (7) |
| –NH2 + H+ + HPO42− → −NH3+---HPO42− | (8) |
Ligand exchange:
| –OH + H2PO4− → −H2PO4 + OH− | (9) |
| –2OH + HPO42− → −HPO4 + 2OH− | (10) |
The adsorption mechanism of ZACen-0.5 mainly includes surface precipitation, electrostatic attraction, and ligand exchange, as summarized as Fig. S13 (ESI†). Although ZACen-0.5 no longer has the memory effect of hydrotalcite, the ethylenediamine modification makes the surface of ZACen-0.5 more hydrophilic as evidenced by a decrease in the water contact angle from 52.8° to 18.84°. Moreover, there is an increase in positive charge, with the Zeta potential rising from 31.93 to 36.38 mV at pH = 5. Concurrently, there is a notable enhancement in specific surface area by 51.02% (from 69.27 m2 g−1 to 104.61 m2 g−1) and pore volume by 51.01% (from 0.1392 cm3 g−1 to 0.2102 cm3 g−1). These modifications compensate for the reduced adsorption capacity resulting from the loss of the memory effect and, in fact, lead to further enhancement of the adsorption capacity.
4. Conclusions
In this study, we introduced ethylenediamine as a novel modifier for ZnAlCu-1%-LDO, resulting in the synthesis of the inorganic metal–organic amine composite adsorbent, ZACen-0.5, which exhibited enhanced adsorption performance for phosphate in wastewater. Our experimental findings highlight several key insights: Firstly, ethylenediamine modification induced a transformative change in ZACen-0.5, creating a new surface state characterized by increased hydrophilicity and improved dispersibility in water. Moreover, the modification process led to the granulation of the adsorbent's structure, along with the enlargement of pores and specific surface area. These alterations not only augmented the adsorption sites but also facilitated the diffusion adsorption of phosphate. Compared to CuZnAl-1%-LDO, ZACen-0.5 exhibited a notably higher adsorption capacity. Secondly, the adsorption mechanism of ZACen-0.5 for phosphate was predominantly governed by monolayer adsorption and chemical adsorption. The kinetics of the adsorption process followed the pseudo-second-order kinetic equation, while the Langmuir isothermal adsorption model accurately described the adsorption behavior. The saturated adsorption capacity reached 271.00 mg P g−1, and the adsorption performance remained promising, retaining 88.2% efficiency even after four cycles of regeneration. Furthermore, when applied to real wastewater containing a phosphate concentration of 3.43 mg P L−1, ZACen-0.5 exhibited exceptional phosphate removal efficiency, surpassing 98%. Post-adsorption analysis revealed a residual phosphate content of 0.0531 mg P L−1, meeting the stringent GB 8978–1996 first-level standard of 0.5 mg P L−1. Overall, the ethylenediamine modification strategy offers a promising avenue for enhancing the phosphate adsorption capabilities of hydrotalcite-like compounds, opening up new possibilities for water treatment applications.
Author contributions
Na Qin: investigation, methodology, data curation, writing – original draft. Weiwei Lin: methodology, investigation, resources. Jianhua Chen: conceptualization, methodology, resources, funding acquisition, supervision, project administration, validation, writing – review & editing. Dingling Gao, Yuxiang Liu and Yayuan Zhang: investigation, project administration, methodology. Qian Yang: conceptualization, methodology, resources, funding acquisition, supervision, project administration, validation.
Conflicts of interest
The authors declare no conflicts of interest.
Acknowledgements
This work was supported by the National Natural Science Foundation of China (No. 21676133) and the Natural Science Foundation of Fujian Province (No. 2021J01990; No. 2021J05192). The author also expresses their sincere appreciation to the anonymous referee for his comments on this manuscript.
References
- X. Liu, M. Wang, L. Deng, Y.-J. Cheng, J. Gao and Y. Xia, Ind. Eng. Chem. Res., 2022, 61, 3831–3839 CrossRef CAS.
- Y. Li, X. Nan, D. Li, L. Wang, R. Xu and Q. Li, Iop Conf. Ser.: Earth Environ. Sci., 2021, 647, 012163 CrossRef.
- D. Xu, C. Zhong, K. Yin, S. Peng, T. Zhu and G. Cheng, Bioresour. Technol., 2018, 249, 783–790 CrossRef CAS PubMed.
- B. Gong, Y. Wang, J. Wang, W. Huang, J. Zhou and Q. He, Bioresour. Technol., 2018, 256, 562–565 CrossRef CAS PubMed.
- L. Li, Z. Zhu, J. Shi, Z. Li and X. Zuo, J. Cleaner Prod., 2023, 384, 135591 CrossRef CAS.
- X. Lu, H. Duan, A. Oehmen, G. Carvalho, Z. Yuan and L. Ye, Water Res., 2021, 203, 117563 CrossRef CAS PubMed.
- M. Ownby, D.-A. Desrosiers and C. Vaneeckhaute, npj Clean Water, 2021, 4, 6 CrossRef CAS.
- M. S. Shalaby, H. Abdallah, A. Cenian, G. Sołowski, M. Sawczak, A. M. Shaban and R. Ramadan, Sep. Purif. Technol., 2020, 247, 116994 CrossRef CAS.
- H. Liang, H. Zhang, Q. Wang, C. Xu, Z. Geng, D. She and X. Du, Sci. Total Environ., 2021, 792, 148452 CrossRef CAS PubMed.
- X. Jing, Y. Li, Y. Shen, Q. Li and Q. Fang, Sci. Total Environ., 2023, 859, 160334 CrossRef CAS PubMed.
- B. Liu, J. Nan, R. Jiang, F. Wu, L. Song, Z. Ge, X. Ye, X. Zhang and W. Wang, Chem. Eng. J., 2023, 451, 138509 CrossRef CAS.
- J. Li, L. Cao, B. Li, H. Huang, W. Yu, C. Sun, K. Long and B. Young, J. Cleaner Prod., 2023, 382, 135395 CrossRef CAS.
- R. Liu, L. Chi, X. Wang, Y. Wang, Y. Sui, T. Xie and H. Arandiyan, Chem. Eng. J., 2019, 357, 159–168 CrossRef CAS.
- H. Yin, X. Yan and X. Gu, Water Res., 2017, 115, 329–338 CrossRef CAS PubMed.
- D. Mitrogiannis, M. Psychoyou, I. Baziotis, V. J. Inglezakis, N. Koukouzas, N. Tsoukalas, D. Palles, E. Kamitsos, G. Oikonomou and G. Markou, Chem. Eng. J., 2017, 320, 510–522 CrossRef CAS.
- Y. Wang, X. Xie, X. Chen, C. Huang and S. Yang, J. Hazard. Mater., 2020, 396, 122626 CrossRef CAS PubMed.
- S. Saadat, E. Raei and N. Talebbeydokhti, J. Environ. Manage., 2018, 225, 75–83 CrossRef CAS PubMed.
- H. Qiu, M. Ye, Q. Zeng, W. Li, J. Fortner, L. Liu and L. Yang, Chem. Eng. J., 2019, 360, 621–630 CrossRef CAS.
- Y. Zou, R. Zhang, L. Wang, K. Xue and J. Chen, Appl. Clay Sci., 2020, 192, 105638 CrossRef CAS.
- M. H. Hassan, R. Stanton, J. Secora, D. J. Trivedi and S. Andreescu, ACS Appl. Mater. Interfaces, 2020, 12, 52788–52796 CrossRef CAS PubMed.
- C. Sun, H. Cao, C. Huang, P. Wang, J. Yin, H. Liu, H. Tian, H. Xu, J. Zhu and Z. Liu, Bioresour. Technol., 2022, 362, 127851 CrossRef CAS PubMed.
- L. Zhang, H. Dan, O. T. Bukasa, L. Song, Y. Liu, L. Wang and J. Li, ACS Omega, 2020, 5, 25326–25333 CrossRef CAS PubMed.
- Y. Wang, Q. Pan, Y. Qiao, X. Wang, D. Deng, F. Zheng, B. Chen and J. Qiu, Adv. Mater., 2023, 35, 2210871 CrossRef CAS PubMed.
- Q. Pan, F. Zheng, D. Deng, B. Chen and Y. Wang, ACS Appl. Mater. Interfaces, 2021, 13, 56692–56703 CrossRef CAS PubMed.
- Y. Wang, F. Zheng, Q. Pan, D. Deng, L. Liu and B. Chen, J. Alloys Compd., 2021, 884, 161162 CrossRef CAS.
- W. Tan, T. Gao and Y. Wang, Langmuir, 2020, 36, 3836–3842 CrossRef CAS PubMed.
- Z. Xu, Y. Zhong, Y. Wang, X. Song and W. Huang, Environ. Sci. Pollut. Res., 2022, 29, 74591–74601 CrossRef CAS PubMed.
- Q. Pan, F. Zheng, D. Deng, B. Chen and Y. Wang, ACS Appl. Mater. Interfaces, 2021, 13, 56692–56703 CrossRef CAS PubMed.
- K. Rybka, J. Matusik and M. Marzec, J. Cleaner Prod., 2022, 332, 130084 CrossRef CAS.
- W. Yang, Y. Kim, P. K. T. Liu, M. Sahimi and T. T. Tsotsis, Chem. Eng. Sci., 2002, 57, 2945–2953 CrossRef CAS.
- H.-J. Kim, T.-H. Kim, J.-M. Oh, F. Salles, G. Chevallier, C. Thouron, P. Trens, J. Soulie, S. Cazalbou and C. Drouet, Mater. Sci. Eng. B, 2022, 280, 115704 CrossRef CAS.
- K. Nava-Andrade, G. G. Carbajal-Arízaga, S. Obregón and V. Rodríguez-González, J. Environ. Manage., 2021, 288, 112399 CrossRef CAS PubMed.
- K. Yang, L. Yan, Y. Yang, S. Yu, R. Shan, H. Yu, B. Zhu and B. Du, Sep. Purif. Technol., 2014, 124, 36–42 CrossRef CAS.
- Y. Tokudome, M. Fukui, S. Iguchi, Y. Hasegawa, K. Teramura, T. Tanaka, M. Takemoto, R. Katsura and M. Takahashi, J. Mater. Chem. A, 2018, 6, 9684–9690 RSC.
- S. Jaśkaniec, C. Hobbs, A. Seral-Ascaso, J. Coelho, M. P. Browne, D. Tyndall, T. Sasaki and V. Nicolosi, Sci. Rep., 2018, 8, 4179 CrossRef PubMed.
- D. L. Gao, W. W. Lin, Q. J. Lin, F. F. Dai, Y. X. Xue, J. H. Chen, Y. X. Liu, Y. Huang and Q. Yang, J. Environ. Chem. Eng., 2023, 11, 109472 CrossRef CAS.
- R. S. Zambare, K. B. Dhopte, A. V. Patwardhan and P. R. Nemade, Desalination, 2017, 403, 24–35 CrossRef CAS.
- A. Hernández-Gordillo, S. Oros-Ruiz and R. Gómez, J. Colloid Interface Sci., 2015, 451, 40–45 CrossRef PubMed.
- A. Hernández-Gordillo, E. Maya-Flores and V. Rodríguez-Gonzalez, Mater. Lett., 2015, 148, 9–13 CrossRef.
- N. Vrtovec, M. Mazaj, G. Buscarino, A. Terracina, S. Agnello, I. Arčon, J. Kovač and N. Zabukovec Logar, Cryst. Growth Des., 2020, 20, 5455–5465 CrossRef CAS.
- K. Tan, S. Zuluaga, E. Fuentes, E. C. Mattson, J.-F. Veyan, H. Wang, J. Li, T. Thonhauser and Y. J. Chabal, Nat. Commun., 2016, 7, 13871 CrossRef CAS PubMed.
- A. Demessence, D. M. D’Alessandro, M. L. Foo and J. R. Long, J. Am. Chem. Soc., 2009, 131, 8784–8786 CrossRef CAS PubMed.
- Z. Huang, Z. Huang, L. Feng, X. Luo, P. Wu, L. Cui and X. Mao, Carbohydr. Polym., 2018, 202, 470–478 CrossRef CAS PubMed.
- M. Huang, S. B. Mishra and S. Liu, J. Alloys Compd., 2017, 718, 270–278 CrossRef CAS.
- G. Xue and J. Ding, Appl. Surf. Sci., 1990, 40, 327–332 CrossRef CAS.
- P. Chen, S. Zeng, Y. Zhao, S. Kang, T. Zhang and S. Song, J. Mater. Sci. Technol., 2020, 41, 88–97 CrossRef CAS.
- Md. A. Qaiyum, R. Kumari, J. Mohanta, P. P. Samal, S. Dutta, B. Dey and S. Dey, J. Cluster Sci., 2023, 34, 963–975 CrossRef.
- D. Cosano, J. Hidalgo-Carrillo, D. Esquivel, F. J. Romero-Salguero, C. Jiménez-Sanchidrián and J. R. Ruiz, J. Porous Mater., 2020, 27, 441–450 CrossRef CAS.
- P. J. A. Withers, J. J. Elser, J. Hilton, H. Ohtake, W. J. Schipper and K. C. van Dijk, Green Chem., 2015, 17, 2087–2099 RSC.
- Q. Zheng, L. Yang, D. Song, S. Zhang, H. Wu, S. Li and X. Wang, Chemosphere, 2020, 259, 127469 CrossRef CAS PubMed.
- Z. Wang, X. Zhang, X. Wu, J.-G. Yu, X.-Y. Jiang, Z.-L. Wu and X. Hao, J. Sol–Gel Sci. Technol., 2017, 82, 440–449 CrossRef CAS.
- J. Wang, M. Cao, C. Jiang, Y. Zheng, C. Zhang and J. Wei, Mater. Lett., 2018, 229, 160–163 CrossRef CAS.
- F. Xie, F. Wu, G. Liu, Y. Mu, C. Feng, H. Wang and J. P. Giesy, Environ. Sci. Technol., 2014, 48, 582–590 CrossRef CAS PubMed.
- J. He, Y. Xu, W. Wang, B. Hu, Z. Wang, X. Yang, Y. Wang and L. Yang, Chem. Eng. J., 2020, 379, 122431 CrossRef CAS.
- L. Zhang, Q. Zhou, J. Liu, N. Chang, L. Wan and J. Chen, Chem. Eng. J., 2012, 185–186, 160–167 CrossRef CAS.
- Y. Yu and J. Paul Chen, J. Colloid
Interface Sci., 2015, 445, 303–311 CrossRef CAS PubMed.
- H. Liu, S. Deng, Z. Li, G. Yu and J. Huang, J. Hazard. Mater., 2010, 179, 424–430 CrossRef CAS PubMed.
- G. Zhang, H. Liu, R. Liu and J. Qu, J. Colloid Interface Sci., 2009, 335, 168–174 CrossRef CAS PubMed.
- Y. Su, H. Cui, Q. Li, S. Gao and J. K. Shang, Water Res., 2013, 47, 5018–5026 CrossRef CAS PubMed.
- Z. Ren, L. Shao and G. Zhang, Water, Air, Soil Pollut., 2012, 223, 4221–4231 CrossRef CAS.
- R. Li, J. J. Wang, B. Zhou, M. K. Awasthi, A. Ali, Z. Zhang, L. A. Gaston, A. H. Lahori and A. Mahar, Sci. Total Environ., 2016, 559, 121–129 CrossRef CAS PubMed.
|
This journal is © The Royal Society of Chemistry and the Centre National de la Recherche Scientifique 2024 |