DOI:
10.1039/D2QM01282K
(Review Article)
Mater. Chem. Front., 2023,
7, 1482-1495
All-alkynyl-protected coinage metal nanoclusters: from synthesis to electrocatalytic CO2 reduction applications
Received
12th December 2022
, Accepted 11th February 2023
First published on 13th February 2023
Abstract
Atomically precise metal nanoclusters have been attracting considerable research interests in the last two decades, thanks to their quantum confinement effect and molecule-like properties. Such properties are significantly affected, if not dominated, by the interfacial metal–ligand bonding motifs. Recently, ligand engineering has been applied to alkynyl molecules, as they can bind to metal atoms with σ and/or π bonds, yielding versatile interfacial bonding motifs hence drastically different properties and functionalities can be realized, as compared to the most commonly employed thiolate ligands. This review first describes the unique advantages of alkynyl-protected metal nanoclusters, with elaboration on the comparison of the interfacial coordination mode and the optical features between thiolate/alkynyl protection. Following that, the recent progress regarding the synthetic strategy is discussed, with an emphasis on the direction reduction method and the synchronous nucleation and passivation strategy. Next, alkynyl-protected metal nanoclusters for the electrochemical CO2 reduction reaction (eCO2RR) are mainly discussed, with some explicit examples to elucidate the metal core effect and surface ligand effect as well as to explain the structure–performance relationship. At last, the challenges and perspectives from synthesis to the eCO2RR of alkynyl-protected metal nanoclusters are proposed. We envision this review to stimulate more research efforts to be dedicated to developing effective synthetic strategies and advancing the catalytic mechanistic understanding of alkynyl-protected metal nanoclusters toward the eCO2RR and beyond.
1. Introduction
1.1. Why study alkynyl-protected metal nanoclusters?
Coinage metal (Au, Ag, Cu, etc.) nanoclusters with an ultrasmall size (usually below 3.0 nm) hold a unique position in the field of nanomaterials, as they possess significantly different optical properties and electronic and microscopic structural features because of the relatively large nanoparticles or nanocrystals thanks to their strong quantum confinement effect.1–3 Such an effect of the metal nanoclusters also leads to their application as antibacterial agents12,13 and versatile applications in various fields, such as photo/electrocatalysis,4,5 environmental remediation,6,7 sensing,8,9 bioimaging or biolabeling,10,11 and so on. More importantly, metal nanoclusters can be chemically synthesized with molecular purity, that is, atomic precision. Atomically precise metal nanoclusters have a well-defined size, a crystallographically resolved structure, and a definitive coordination environment at the atomic level, hence they play a critical role in the fundamental nanoscience study, because they can serve as a model to correlate the structure–property/performance relationship. In most cases, metal nanoclusters hold a spherical core@shell configuration,14–16 where a few or tens of metal atoms are arranged in a certain pattern in the core and the ligand molecules are capped onto the metal core as the protective shell.17–19
The surface ligands coordinate with a certain portion of core metal atoms to form some interfacial bonding motifs, which affect if not dominate the physicochemical properties of metal nanoclusters. Currently, thiolate molecules,20–22 phosphine,17,23 halogen,24,25N-heterocyclic carbenes,26,27 and other organic compounds28,29 have been widely employed to stabilize the metal core to form a large quantity of molecular metal nanoclusters. Recently, alkynyl ligands have been continuously gaining increasing research attention thanks to their characteristic binding patterns with surface metal atoms.30–33 For instance, compared with the most extensively employed thiolate molecules, alkynyl molecules can form σ and/or π bonding with Au or Ag atoms,34,35 yielding more diverse interfacial bonding motifs, which endow alkynyl-protected coinage metal nanoclusters with drastic physicochemical properties and functionalities.
As illustrated in Fig. 1, this review first emphasizes the uniqueness of alkynyl ligands over other ligands for preparing metal nanoclusters, with a particular focus on surface coordination motifs, optical properties, and catalytic performance. Following that, the controllable synthesis of molecular alkynyl-protected metal nanoclusters is discussed, mainly elaborating on the direct reduction (DR) method and the synchronous nucleation and passivation (SNP) strategy. Final and the major part is regarding atomically precise alkynyl-protected metal nanoclusters for the electrochemical CO2 reduction reaction (eCO2RR), involving the significance and mechanism of eCO2RR and some explicit examples for elucidating the structure–performance relationship.
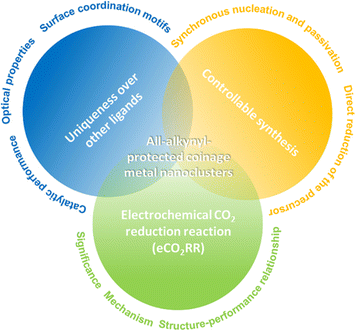 |
| Fig. 1 The main content in this review regarding all-alkynyl-protected metal nanoclusters. | |
1.2. The comparison of alkynyl ligand vs. thiolate ligand protected nanoclusters
1.2.1. The comparison of coordination modes.
The coordination modes of an alkynyl molecule on a metal surface are more variable due to the C
C bond, as the terminal C atom can attach to the metal core by forming a σ bond, while the C
C bond can coordinate the metal atom in the form of π bonding. For example, the interfacial binding motifs of alkynyl-protected Au nanoclusters can be divided into the five essential types:34 μ1-η1; μ2-η1, η1; μ3-η1, η1, η1; μ2-η1, η2 and linear PhC
C–Au–C
CPh (Fig. 2a), much more diverse and complex than the thiolate-protected Au nanoclusters, which has only roughly two types of binding motifs,36 that is, linear RS–Au–SR, and lengthened RS–Au–SR–Au–SR (Fig. 2c). For Ag nanoclusters, there are more diverse approaches for alkynyl molecules to coordinate with Ag atoms.32,37 As illustrated in Fig. 2b, in motifs C, D, and E, the –C
C–R group can bind to Ag than Au at least one or even two more metal atoms. Such a much more diverse binding behaviour from alkynyl molecules than thiolate ligands might be favourable for catalysis.
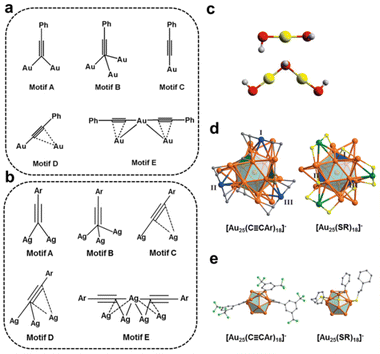 |
| Fig. 2 Fundamental coordination modes of alkynyl ligands on (a) Au atoms and (b) Ag atoms. (c) Essential bonding modes of thiolate ligands on metal atoms. Comparison of the geometric configurations of (d) V-shaped staples and (e) surface motifs on [Au25(C CAr)18]− and [Au25(SR)18]−. Copyright 2018, American Chemical Society and Wiley,34,38 and Copyright 2020, Wiley.36 | |
The structure comparison of [Au25(C
CAr)18]− and [Au25(SR)18]− is an explicit example.38 Both clusters have Au13 icosahedra capped by six Au2L3 units with similar arrangements. Even if the six V-shaped [Au2(C
CAr)3] staples in [Au25(C
CAr)18]− are similar to [Au2(SR)3] in [Au25(SR)18]−, the connection to the Au13 kernel is quite different. Three Au atoms in V-shaped staples (labelled in blue, Fig. 2d) of [Au25(C
CAr)18]− are twisted about 60°, referring to that in [Au25(SR)18]− (labelled in green, Fig. 2d), resulting in the two Au atoms in [Au2(C
CAr)3] capping the adjacent triangular pattern, while two Au atoms in [Au2(SR)3] are capped by the uniformly distributed triangles. Moreover, it also leads to [Au25(C
CAr)18]− being present as a racemate. In addition, the angle between the terminal C
C to Au atom is about 180°, while the angle of C–S–Au is less than 106° (Fig. 2e). This case exemplifies that the alkynyl ligand significantly affects the metal core configuration of the coinage metal nanoclusters.
1.2.2. The comparison of optical properties.
As the ligand interacts with a significant amount of metal core atoms of the nanocluster, it plays a critical role in modulating the electronic structure of the metal nanoclusters. For instance, the optical absorbance feature of alkynyl-protected metal nanoclusters is (drastically) different from other ligand capped nanoclusters. As shown in Fig. 3a, both alkynyl-protected and thiolate protected Au25 nanoclusters have a broad peak at ∼700 nm, and all the other peaks from the alkynyl-protected Au25 molecules are red-shifted about 50 nm compared to thiolate protected Au25 nanoclusters.38Fig. 3b compares the absorbance spectra between the alkynyl and thiolate protected Au36 nanoclusters. Au36(SR)24 displays multiple absorbance peaks, and in stark contrast, a significant red shift in the wavelength region beyond 500 nm is observed for Au36(PhC
C)24 and the intensity of the characteristic peaks in the low wavelength is strongly reduced even disappears to some extent.39
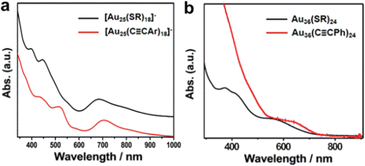 |
| Fig. 3 The comparison of optical properties of (a) [Au25(PhC CAr)18]−, [Au25(SR)18]−and (b) Au36(PhC C)24, Au36(SR)24. Copyright 2017 and 2018, Wiley.38,39 | |
In addition to the optical absorption feature, alkynyl molecules can also affect the luminescence properties of the metal nanoclusters, e.g. the photoluminescent behaviour and the corresponding quantum yield. For example, Konishi et al. reported that, despite the photoluminescence spectra of [Au13(dppe)5(C
CPh)2](PF6)3 is similar to that of [Au13(dppe)5Cl2](PF6)3, the quantum yield of the former one (0.16) is significantly higher than that of the latter one (0.11).40 The Wang group discovered that, Au22(tBuC
C)18 has a strong luminescence in the solid state with a quantum yield of 15%, and interestingly, it displays thermochromic luminescence.41 By using the chiral alkynyl group, the Zang group fabricated a new enantiomeric pair of superatomic Ag17 nanoclusters, and both molecules emit near-infrared light with a quantum yield of 8.0% under ambient conditions as well as NIR circularly polarized luminescence thanks to the chirality of the excited states.42 The emission colour of Ag51(tBuC
C)32 reported by Lu and Xie groups can change from blue to red by changing the solvent polarity from the less polar dichloromethane to the more polar methanol, exhibiting a strong solvatochromic effect.43
1.2.3. The comparison of catalytic performance.
As the electronic structure and physiochemical properties of metal nanoclusters can be affected by the surface ligand, engineering the surface ligand can fine-tune the catalytic performance of the metal nanoclusters. Specifically, once the alkynyl molecules are attached onto the metal core to form a large –C
C–M (M = metal) conjugated system, it favours the electron shuttling and hence promotes the catalytic performance for certain reactions. For instance, Wan et al. reported that, in alkyne semi-hydrogenation catalysis, the conversion rate of [Au38(L)20(Ph3P)4]2+ (L = alkynyl ligands) as catalyst reached up to 97%, while that of thiolate stabilized Au38 with the same core structure was less than 2%.44 Such a ligand effect also occurs in the hydrogen evolution reaction (HER). The Tsukuda group found that, the onset potential of hydrogen evolution for Au25(C
CArF)18 was about 70 mV positive than that of Au25(SC2H4Ph)18 under the same conditions, indicating that alkynyl ligands significantly enhanced the HER activity.45
2. Controllable synthesis
Since the seminal Brust method was reported for preparing monodisperse nanoclusters in 1994, various strategies have been developed for the controllable synthesis of metal nanoclusters with molecular purity. For alkynyl-protected metal nanoclusters, the applicable synthetic methods include a direct reduction (DR) of the precursor,39,46 synchronous nucleation and passivation (SNP),37,47,48 ligand exchange,40,49,50 one-pot method41,51,52 and so on (Fig. 4). With these methods on hand, plus the advancement of crystallographic techniques, a great deal of all-alkynyl-protected Au, Ag, and AuAg alloy nanoclusters with crystal structures have been recorded. This review will mainly discuss the DR method and SNP strategy.
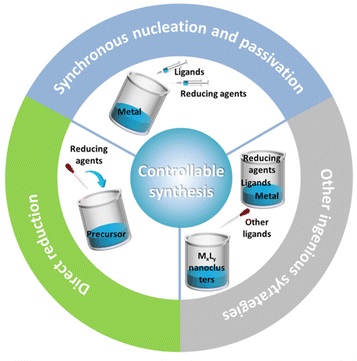 |
| Fig. 4 Controllable synthesis strategies for all-alkynyl-protected metal nanoclusters. | |
2.1. Direct reduction of the precursor
Direct reduction (DR) of the precursor is the most facile and straightforward method to fabricate metal nanoclusters, but manipulating the reaction kinetics to obtain a monodisperse product is quite challenging. Currently, the DR approach is the most widely employed strategy to acquire alkynyl-protected metal nanoclusters. Specifically, metal salts (e.g. Me2SAuCl,30,53,54 Ag2O31,55) were first reacted with the alkynyl ligand to generate the Au(I)/Ag(I)-alkynyl complexes (the precursor), then the reducing agent (e.g. NaBH443,56) was added to reduce the precursor to form metal nanoclusters. In some cases, a mixture of cluster molecules is acquired, hence the post-synthetic isolation and purification are necessary. So far, Au42,30 Au99,53 Ag47,31 Ag112,32 and Au34Ag2857 have been chemically prepared using this method.
It is worth noting that the morphology and aggregation state of the precursor can be critical for forming the final product. Our group disclosed that, by introducing ethanol in the preparation of Au–PA (PA = phenylacetylene) precursor, the amorphous precursor can self-assemble into flower-like macromolecules54 (Fig. 5, denoted as (Au–PA)f). Consequently, compared with the amorphous precursor, the well-defined (Au–PA)f precursor can lead to the formation of Au144(PA)60 with higher yield and monodispersity. Such a phenomenon is reminiscent of the high-yield synthesis of Au25(SR)18, in which the monodisperse Au–SR precursor was obtained by controlling the reaction kinetics.58
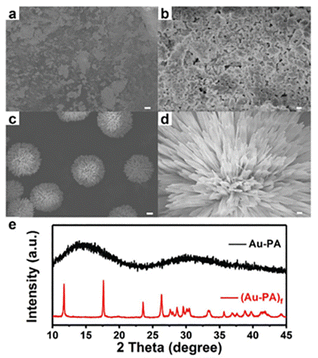 |
| Fig. 5 SEM images of Au–PA precursors at (a) 5 μm, (b) 500 nm and (Au–PA)f precursors at (c) 5 μm, (d) 500 nm. (e) XRD patterns of the amorphous Au–PA precursor generated without ethanol and the flower-like (Au–PA)f precursor generated with the presence of ethanol. Copyright 2020, Royal Society of Chemistry.54 | |
2.2. Synchronous nucleation and passivation strategy
To form a nanocluster molecule, it undergoes nuclei growth and surface passivation process which compete with each other. For the DR approach, it might be difficult to manipulate these two processes to improve the yield of the target cluster. For instance, an excess amount of reducing agent may lead to excessive reducibility to form large nanoparticles spontaneously, while a rapid reduction rate may induce the surface passivation occurring inhomogeneously hence increasing the polydispersity of the product. From that, regulating the reaction rate and manipulating the reducing process might be critical for enhancing the yield of molecular metal nanoclusters.
In 2020, our group developed a synchronous nucleation and passivation strategy to fabricate Au36(PA)24 and Au22(PA)18 nanoclusters with a high yield of 17.1% and 70.1%, respectively.47 In this method, the ligand and reducing agent were added simultaneously, and more importantly, NaBH3CN instead of NaBH4 was employed as the reductant, and the reaction was also conducted at a low temperature. By doing that, the cluster nuclei growth and surface ligand passivation can be more easily manipulated to obtain molecular metal nanoclusters. As illustrated in Fig. 6, the equivalent ratio of NaBH3CN-to-Au is critical for yielding different products. When NaBH3CN is much more excessive (eq ≫ 3), polydisperse Au nanoclusters were acquired; when NaBH3CN was decreased to a certain low amount (eq = 1/2, 1/3), Au22(PA)18 as the reaction intermediate formed, but it can transform into Au36(PA)24 overnight eventually. In this work, we also proposed the structure evolution from Au22(PA)18 to Au36(PA)24, and the calculated stoichiometric ratio of Au22(PA)18-to-Au36(PA)24 was verified by the designed experiment as well. Such synchronous nucleation and passivation strategy is of great universalities to prepare alkynyl-protected Ag, AuAg alloy nanoclusters, and in later studies, other molecules such as Ag32,37 and Au9Ag948 clusters were synthesized by this method with high yields.
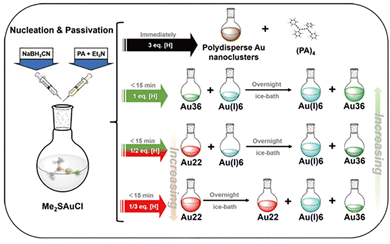 |
| Fig. 6 Synthetic routes for Au36(PA)24 with the different amounts of reducing agents in the synchronous nucleation and passivation strategy. Copyright 2020, Springer.47 | |
2.3. Other ingenious synthesis strategies
Besides the above two approaches, there are some other ingenious strategies to synthesize homoleptic alkynyl-protected metal nanoclusters. One method is the ligand exchange reaction, in which another ligand is introduced into a solution of the parent cluster with high polydispersity or molecular purity to yield new cluster molecules.59 Ligand exchange can transform the cluster size, morphology, composition, and structural arrangement and also introduce new chemical/physical properties.49,60,61 Pioneering work using this method to prepare all-alkynyl-protected metal clusters was done by Tsukuda et al. In 2011, by reacting polyvinylpyrrolidone stabilized Au nanoclusters with a series of alkynyl molecules, several magic clusters such as Au54(PA)26, Au94(PA)38, and Au110(PA)40 can be identified in the mass spectra of the final mixture product.62 In 2019, the same group reported a quasi ligand exchange reaction, in which hydride-doped [HM@Au8(PPh3)8]+ (M = Pd, Pt) reacting with Au(I)-alkynyl oligomer to yield [MAu24(C ≡ CArF)18]2− clusters.63 Interestingly, Hosier et al. revealed that the simple thiolate-to-acetylide ligand exchange is enthalpically unfavorable, while metathesis reactions between these ligands are enthalpically favorable.64 However, the direct reaction between phenylacetylene and thiolate Au clusters can not proceed, and the acetylide-for-thiolate ligand exchange is only facile when using either a gold(I)–phenylacetylide complex or lithium phenylacetylide as an incoming ligand to thiolate protected Au clusters. From this point, using ligand exchange to prepare alkynyl-protected metal nanoclusters has been restricted to some extent.
Another strategy is the one-pot synthetic approach, which is quite straightforward and of easy operability. The most significant feature of this method is that all the reagents (the metal salt, the ligand, the reductant, etc.) were added together to react in one system, similar to SNP but with less kinetic control and easier to operate. For instance, the Tsukuda group fabricated a series of homoleptic alkynyl-protected Au22(C
CR)18 cluster molecules protected with four different ligands by reacting (Me2S)AuCl, the ligand, and triethylamine together.51 Interestingly, triethylamine not only serves as a base, but also is the reducing agent, and its low reducing capability left (Me2S)AuCl not completely reduced, hence the yields of all the clusters were less than 8%. In another study, the Wang group synthesized a pair of Au23(C
CtBu)15 clusters (Au23-1 and Au23-2), which are the first isomers observed in all-alkynyl-protected metal nanoclusters.52 Specifically, Au23-1 was prepared by reduction of Me2SAuCl and HC
CtBu/Et3N with NaBH4, but Au23-2 has to be acquired with a similar synthetic procedure in the presence of tetraphenylphosphonium chloride or tetrabutylammonium chloride. The yield of Au23-1 and Au23-2 was 31% and 10%, respectively. It shows that, by optimizing the synthetic parameters, the one-pot method can obtain molecular metal nanoclusters with high yields, but more cases await to be explored.
3. Alkynyl-protected metal nanoclusters for eCO2RR
3.1. The importance of eCO2RR
Since the last century, the overuse of fossil fuels and rapid industrialization has caused serious greenhouse effects globally.65 CO2 is the major greenhouse gas, whose emission must be reduced to a certain minimum level to meet the framework of the Paris Agreement. Capturing, storing, and utilizing CO2 is one of the effective means to mitigate the great CO2 emission pressure.66 Among that, electrochemical CO2 reduction reaction (eCO2RR) has been attracting increasing research attention recently, as it can convert CO2 into valuable chemicals. More importantly, eCO2RR can be conducted under mild conditions with high efficiency, and take advantage of renewable electricity.67,68 However, the C
O bond is difficult to be broken because it has large bond dissociation energy of 750 kJ mol−1. Meanwhile, as it has multiple proton-coupled electron transfer steps with comparable potentials, plus the side reaction of hydrogen evolution, it is extremely challenging to control the product selectivity.65 The presence of electrocatalysts can lower the energy barrier of eCO2RR, but most of the electrocatalysts still suffer from high overpotential, low current density, and poor product selectivity.69 Therefore, developing electrocatalysts with high efficiency, high activity and selectivity is imperative.
3.2. The mechanism of eCO2RR
As multiple protons/electrons transfer are involved in eCO2RR, the reaction pathway and mechanism of eCO2RR are rather complicated. Based on the electron-transfer number, the reduction products can be classified into C1 molecules such as CO, HCO2H, CH3OH, and C2+ molecules such as CH3CH2OH, CH3CHO, C2H6, C2H4, etc. Regardless of the product generated, eCO2RR follows the basic process: 1. Adsorption of CO2 molecules on the catalyst surface; 2. Proton coupled electron transfer reactions of the intermediates; 3. Recombination of molecules and desorption from the surface.70 As molecular Au/Ag nanoclusters mainly produce CO products, we here focus on 2e− reaction only. An important concept regarding the reaction process is the rate-determining step (RDS), which is defined as the step with the largest energy barrier in the process. The overall reaction rate is governed by the RDS.
The general mechanism of the 2e− reaction is presented in Fig. 7. Generally, the *COOH formation is the key step for producing CO, and there are two pathways to form *COOH. In one way, CO2 grasps an electron to transform into *CO2− and then obtains a proton to produce *COOH, while in another way, CO2 directly combines with a pair of proton and electron (H+/e−) on the catalyst surface to form *COOH. CO and H2O are the products once *COOH reacts with a pair of H+/e−. From *CO, CH3OH and CH4 can also be yielded, depending on the number of electron pairs. The formation of HCO2H also has two possible pathways. On the one hand, the protonation of *CO2− generates *OCHO first and *OCHO gets a pair of H+/e− to form HCO2H. On the other hand, CO2 interacts with H which is pre-adsorbed on the catalyst surface by a pair of H+/e− to form *HCOO, and then HCO2H is produced through reacting with a pair of H+/e−.65 In addition, density functional theory (DFT) calculations help to visualize the reaction path of eCO2RR and the required energy to produce various intermediates, specific examples will be discussed in detail below.
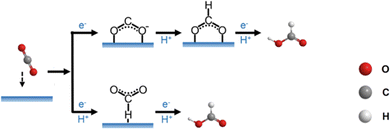 |
| Fig. 7 Schematic diagram of the 2e− reaction path in eCO2RR. Copyright 2021, Elsevier.65 | |
3.3. Alkynyl-protected metal nanoclusters for eCO2RR
Metal nanoclusters especially Au and Au-alloy nanoclusters have been widely employed as eCO2RR catalysts, thanks to the unique advantages that are not available from nanoparticle counterparts. First of all, the molecule-like transition of metal nanoclusters can facilitate the electron transfer to the electrode and reactant hence decreasing the overpotential; secondly, metal nanocluster has tunable and versatile chemical functionalities, e.g. by tuning the size, surface ligand, charge state, doping another metal, and structure engineering, the redox potential can be manipulated to enhance the catalytic performance. Finally and most importantly, metal nanoclusters have a well-defined molecular structure with atomic precision, which is quite favourable for theoretically simulating the experimental findings to unravel the reaction mechanism and disclose the catalytic reaction center, hence establishing the structure–performance relationship eventually.67
So far, Au nanoclusters capped by all kinds of ligands (e.g. thiolate,71,72 phosphine,27 hydrides,73 NHC,74 and alkynyl75,76) especially thiolate molecules have been studied for eCO2RR. Pioneering work has been conducted on thiolate-stabilized molecular Au nanoclusters by Jin groups,77–80 and multiple groups have also made significant contributions.81–84 Other ligands and mixed ligands protected Au nanoclusters also exhibited impressive performance toward eCO2RR.73 For instance, the Wang group reported that the mixed ligand capped [Au55(p-MBT)24(Ph3P)6](SbF6)3 (Au55) can reach the highest FECO of 94.1% at −0.6 V with the maximal CO production rate of 50.34 μL min−1 at −0.9 V. Moreover, the Au55 catalyst exhibited good stability for over 4 h. The authors attributed the good catalytic properties to the ultrasmall size of the Au55 cluster, the corner Au atom, and the hydrophobic nature of the ligand.85 In another study, the same group developed all-amidinate-protected [Au28(Ph-form)12](OTf)2 clusters loaded on carbon nanotubes as a catalyst, which showed the maximal FECO of 97.5% at −0.57 V, and can still maintain over 91% for 40 h at −0.69 V in eCO2RR.86
Recent high-quality review papers regarding metal nanoclusters for eCO2RR mainly focus on the thiolate ligand, whereas a systematic summary of alkynyl-protected metal clusters for eCO2RR is missing.67,87,88 Here, we mainly discuss all-alkynyl-protected Au and Ag nanoclusters for eCO2RR, with particular attention on elucidating the metal core effect and the surface ligand effect.
3.3.1. Metal core effects.
Given the uniqueness of alkynyl ligands over thiolate molecules mentioned in Section 1.2, we tend to believe alkynyl-protected metal nanoclusters might be more favourable for eCO2RR. Nevertheless, rare reports can be found on alkynyl-protected Au or Ag nanoclusters for the eCO2RR.
In 2021, our group reported the structure analysis and eCO2RR performance of the smallest all-alkynyl-protected [Ag15(C
CtBu)12]+ (abbreviated as Ag15) nanocluster.89 As shown in Fig. 8a, Ag15 possesses a body-centered-cubic (bcc) structure with an Ag@Ag8@Ag6 metal core configuration. Interestingly, the two diagonal Ag atoms in the Ag8 cube can connect to the two O atoms of the CO2 molecule at two opposite sides, making the Ag8 cube elongated and the Ag15 nanocluster assembled into one-dimensional material (Fig. 8b). Interestingly, Ag15 exhibited excellent catalytic activity toward eCO2RR with CO as the main product and H2 evolution is significantly suppressed, and the maximum FECO can reach ca. 95% at -0.6 V (Fig. 8c). In addition, the Ag15 catalyst can maintain good stability over 10 h (Fig. 8d).
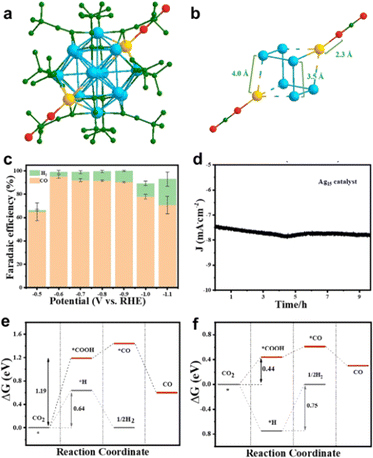 |
| Fig. 8 Diagram of CO2 adsorption on (a) [Ag15(C CtBu)12]+ and (b) Ag8 cube. (c) Faradaic efficiency of CO and (d) stability testing of [Ag15(C CtBu)12]+. Comparison of free energy of electroreduction of CO2 to CO process on (e) [Ag15(C C–CH3)12]+ and (f) [Ag15(C C–CH3)11]+. Copyright 2021, Wiley.89 | |
DFT calculations were further conducted to determine the catalytic site and compare the catalytic selectivity of eCO2RR to CO vs. H2 evolution. The methyl group was used to replace the tert-butyl group to simplify the calculations, and one ligand stripping to expose the undercoordinated metal atom serves as the active site. For both the intact [Ag15(C
C–CH3)12]+ cluster and one ligand stripped [Ag15(C
C–CH3)11]+, the formation of *COOH is the rate determining step (RDS). As shown in Fig. 8e and f, [Ag15(C
C–CH3)11]+ has much lower free energy (0.44 eV) to form *COOH rather than [Ag15(C
C–CH3)12]+ (1.19 eV). Correspondingly, [Ag15(C
C–CH3)11]+ has higher energy barrier for H2 evolution than [Ag15(C
C–CH3)12]+. Moreover, the energy barrier for *H formation is much larger than forming *COOH for [Ag15(C
C–CH3)11]+, suggesting H2 generation can be largely restricted. On the intact [Ag15(C
C–CH3)12]+ cluster, the staple Ag atom acts as the active site for eCO2RR, while in the one-ligand removed [Ag15(C
C–CH3)11]+, the loss of one ligand causes 4 shell-Ag atoms to expose two equivalent triangular faces, in which one undercoordinated Ag atom prefers to CO2 adsorption and reduction. This study manifests that the alkynyl-protected Ag nanocluster holds great potential for the eCO2RR in terms of activity and stability and can advance the fundamental understanding of the eCO2RR catalyzed by alkynyl-protected metal nanoclusters.
Doping another metal into the metal core to form bimetallic nanoclusters can alter the physiochemical properties of the clusters and lead to enhanced catalytic performance due to the synergistic catalytic effect.90–92 The well-defined Ag@Ag8@Ag6 metal core structure of the Ag15 nanocluster allows us to conduct such metal exchange to tune and optimize the eCO2RR performance. In a following study, through the metal exchange, our group first prepared the [Au7Ag8(tBuC
C)12]+ (Au7Ag8 in short) cluster, of which the optical feature and crystal structure agree well with the previous report from Zheng group.93 Meanwhile, the [Ag9Cu6(tBuC
C)12]+ (Ag9Cu6 in short) cluster was also synthesized via a one-pot strategy, and it displayed significantly different optical properties and chemical stability from Au7Ag8.94 Furthermore, the [Au2Ag8Cu5(tBuC
C)12]+ cluster (Au2Ag8Cu5 in short) was also prepared by a metal exchange approach from Ag9Cu6.95
It is worth noting that, Au7Ag8, Ag9Cu6, and Au2Ag8Cu5 are all M15 clusters with a similar metal core configuration. As demonstrated in Fig. 9a, they all possess an M@M8@M6 metal core, with one metal atom in the center, an M8 cube in the middle layer, and an M6 octahedron in the outmost layer in the core. Interestingly, the three clusters exhibited drastically different catalytic performances toward eCO2RR. Au7Ag8 presented high selectivity for CO formation (Fig. 9b), with the highest FECO of 98.1% at −0.49 V. For both Ag9Cu6 and Au2Ag8Cu5, CO and formate are the main products, despite the FECO showed a similar volcanic shape with Au7Ag8 and the highest FECO can reach 94.1% and 95.0% at −0.49 V for Ag9Cu6 and Au2Ag8Cu5, respectively, the FECO decreased rapidly when the potential goes more negatively (Fig. 9b). Fig. 9c depicts FEformate of Ag9Cu6, and Au2Ag8Cu5. When the potential goes negative, FEformate of Ag9Cu6 kept increasing while FEformate of Au2Ag8Cu5 first increased gradually and then decreased. The maximal FEformate value for Ag9Cu6 and Au2Ag8Cu5 is 47.0% at −1.19 V and 28.3% at −0.99 V, respectively. In addition, the highest FECO+formate for Ag9Cu6 and Au2Ag8Cu5 is ∼100.0% and ∼97.4%, suggesting both clusters were able to convert CO2 into valuable chemicals. Meanwhile, both Au7Ag8 and Ag9Cu6 can suppress H2 evolution, while at a higher negative potential range, HER gradually becomes dominant for Au2Ag8Cu5 (Fig. 9d). Correspondingly, both Au7Ag8 and Ag9Cu6 exhibited robust stability while Au2Ag8Cu5 showed slightly inferior long-term durability than the above two clusters, probably due to its metal core (Au1@Au1Ag4Cu3@Ag4Cu2) is slightly asymmetric.
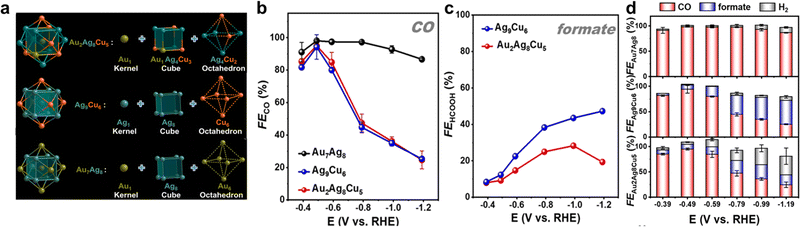 |
| Fig. 9 (a) Structural anatomy of three nanoclusters. (b) FECO of three nanoclusters and (c) FEformate of [Ag9Cu6(C CtBu)12]+, [Au2Ag8Cu5(C CtBu)12]+. (d) Product selectivity of three nanoclusters. Copyright 2022, Royal Society of Chemistry.95 | |
By using the simplified single-crystal structures to build models, DFT calculations were next conducted to unravel the reaction mechanism. The eCO2RR and HER compete at the same metal site, specifically, Au for Au7Ag8, Cu for Ag9Cu6, and for Au2Ag8Cu5, the staple Cu acts as the active site for HCOO* binding, while *COOH, *CO, and *H tend to bind with the sub-surface Au atom. It is predicted that one ligand stripping to expose the metal atom is the active center. For the intact Au7Ag8 cluster, the energy barrier to form *H (0.88 eV) is lower than that of forming *COOH (1.08 eV, Fig. 10a) hence not favours eCO2RR. However, once one ligand is stripped, trans-COOH* prefers to bind to the undercoordinated Ag atom and ΔG significantly decreases to 0.47 eV, much lower than ΔG for desorption of *H (1.07 eV) hence eCO2RR is quite favourable (Fig. 10b). For the Ag9Cu6 cluster, eCO2RR and HER compete at the Cu site regardless of the structural integrity. As shown in Fig. 10c, the RDS for CO generation is forming *COOH (ΔG = 0.43 eV), and the RDS for formate generation is *HCOO-to-HCOOH (ΔG = 0.46 eV), while after stripping a ligand, the value is changed into 0.40 eV and 0.54 eV (Fig. 10d), respectively. However, for the intact Ag9Cu6 cluster, the energy barrier for H2 formation is only 0.14 eV, hence HER prevails. The reaction mechanism of Au2Ag8Cu5 is rather complicated, and it turns out that the removal of one alkynyl ligand bonded to two Ag atoms near the shell Au atom is more thermodynamically supported. When the Au2Ag8Cu5 cluster is intact, ΔG of the RDS in *COOH generation is 0.62 eV (Fig. 10e), rather larger than the corresponding RDS energy barrier when removing one ligand (Fig. 10f), suggesting that the latter one favours CO formation. Furthermore, for forming formate, the active site shifted from the Cu site to the Au site, and the RDS changed from *HCOO-to-HCOOH (0.67 eV) to the generation of *HCOO (0.44 eV). Such shift of the active site may be responsible for the decrease of formate selectivity of Au2Ag8Cu5 compared to Ag9Cu6.
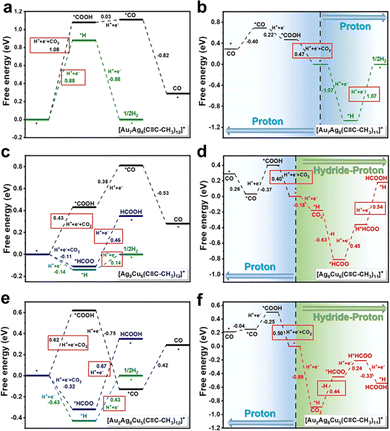 |
| Fig. 10 Free energy of eCO2RR and HER on (a) and (b) intact and incomplete Au7Ag8, (c) and (d) Ag9Cu6 and (e) and (f) Au2Ag8Cu5. Copyright 2022, Royal Society of Chemistry.95 | |
The series of the M15 clusters (Ag15, Au7Ag8, Ag9Cu6, Au2Ag8Cu5) with the identical ligand shell offers a good example to examine the metal core effect of alkynyl-protected metal nanoclusters. For both Ag15 and Au7Ag8, CO is formed exclusively with high selectivity. However, with the presence of Cu, formate can be produced by Ag9Cu6 and Au2Ag8Cu5, and the two-atom-difference led to drastically different eCO2RR behaviours. Ag9Cu6 has higher FEformate than Au2Ag8Cu5 at the applied potential, and at high negative potentials, the HER becomes dominant for Au2Ag8Cu5. For Ag15 and Au7Ag8, one ligand stripping exposed the Ag and Au atom as the active site to form CO, respectively, while for Ag9Cu6 and Au2Ag8Cu5, one ligand stripping exposed the Cu atom as the active site in the eCO2RR, but the active site was shifted to the Au atom for producing formate for Au2Ag8Cu5. That is, the exposed (111)-like Ag2Cu2 surface and the Au1Cu1Ag2 surface are responsible for the markedly different eCO2RR properties especially the selectivity of formate.
3.3.2. Ligand effects.
The surface of the metal nanoclusters is capped by organic molecules, and these ligands can affect the physicochemical properties and electronic structure of the metal nanoclusters hence play a critical role in fine-tuning the electrocatalytic performance of the metal nanoclusters. For instance, an early study by the Jin group discovered that, compared to the phenylselenol (–SePh) protected Au25 cluster, the thiolate Au25 (–PET) cluster exhibited higher FECO and mass activity of CO while the former one exhibited higher FEH2.96 DFT calculations revealed that the breakage of ligand carbon tail to expose S/Se atoms as the active site. Due to the higher electron density, the S site is more favourable for forming the key intermediate of *COOH.
Recently, Wang et al. reported a ligand–shell engineering of Au28 clusters toward eCO2RR, in which Au28(C2B10H11S)12(tht)4Cl4 (Au28–S) and [Au28(C4B10H11)12(tht)8]3+ (Au28–C) with the identical Au28 metal core showed different eCO2RR performance.97 Specifically, Au28–S is co-protected by carboranethiolate, tetrahydrothiophene (tht), and chloride, while Au28–C is co-protected by carboranealkynyl and tht ligands (Fig. 11a and b). Interestingly, despite the same metal kernel, the different protecting layer leads to the change in the overall electronic structure, e.g. Au28–C has 13 valence electrons while Au28–S has 12. In addition, compared to Au28–C, the emission peak is blue-shifted at about 167 nm with the intensity increased about 5 times for Au28–S. Furthermore, Au28–S exhibited markedly superior eCO2RR properties than Au28–C. As illustrated in Fig. 11c, Au28–S showed higher FECO values in all tested potentials, and it achieves the maximum FECO of 98.5% at −0.9 V, about 1.4 times than that of Au28–C. However, both clusters exhibited satisfactory stability with a negligible change of FECO (Fig. 11d). In situ FTIR analysis shows that the intensity of the peaks at 1362 and 1636 cm−1 attributed to the *COOH species from Au28–S is stronger than that from Au28–C. Moreover, the authors conducted DFT calculations to unravel the active site and elucidate the reaction mechanism. For Au28–C, the Au atoms in the linear C2B10H11–C
C–Au–C
C–C2B10H11 motifs are accessible for CO2 molecules, while removing Cl atoms to expose Au atoms are probably the active center for Au28–S. Both clusters adopt the CO2(g)–*CO2–*COOH–*CO–CO pathway, while the formation of *COOH is the rate-determining step. Au28–C has a ΔG of 0.85 eV, much smaller than that of Au28–C with 1.11 eV, while high ΔG of H* adsorption indicated that HER is unfavorable on both Au28–S and Au28–C (Fig. 11e and f), yet Au28–S is even more unlikely for HER to occur.
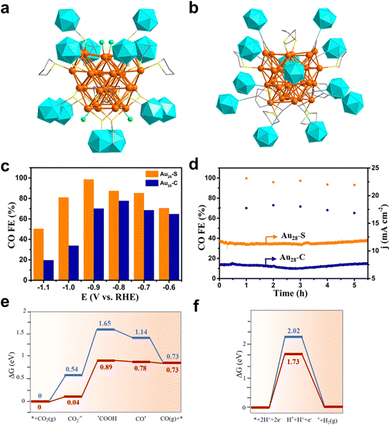 |
| Fig. 11 The total structures of (a) Au28(C2B10H11S)12(tht)4Cl4 and (b) [Au28(C4B10H11)12(tht)8]3+. (c) Faradaic efficiency of CO and (d) stability testing of two nanoclusters. Free energy of (e) eCO2RR and (f) HER on Au28(C2B10H11S)12(tht)4Cl4 (red) and [Au28(C4B10H11)12(tht)8]3+ (blue). Copyright 2022, Wiley.97 | |
In another recent study reported by our group, the surface ligand effect of Ag32 nanoclusters toward eCO2RR has been probed.37 In this study, two metal clusters, a homoleptic alkynyl-protected Ag32L24 (L = 3,5-bis(trifluoromethylbenzene) acetylide, Ag32 in short) plus thiolate and phosphine co-protected [Ag32(DPPE)5(SR)24]2− were prepared. Interestingly, thanks to the σ and/or π binding between Ag and the C
C bond, Ag32 presented more versatile ligand–metal binding motifs than that of [Ag32(DPPE)5(SR)24]2−. Such structure difference leads to drastically different eCO2RR performance. As demonstrated in Fig. 12a and b, in all the tested potentials, FECO of Ag32 is higher than that of [Ag32(DPPE)5(SR)24]2−. The highest FECO is 96.44% at −0.8 V for Ag32, while in stark contrast, only a maximum of 56.67% can be achieved at −1.0 V for [Ag32(DPPE)5(SR)24]2−. Impressively, both samples exhibited excellent rather comparable stability for prolonged operation, as no significant current decrease was observed for 15 h (Fig. 12c). DFT calculations disclosed that, one ligand stripping to form Ag32L23 as the active center for Ag32, however, for [Ag32(DPPE)5(SR)24]−, as the Ag–P bond strength is stronger than that of the Ag–S bond, one –SR ligand stripping to form [Ag32(DPPE)5(SR)23]− as the active center. As depicted in Fig. 12d, the free energy profile shows that [Ag32(C
C–CH3)23]+ has a smaller energy for forming *COOH (0.4 eV) rather than [Ag32(P2C2H6)5(SCH3)23]− (0.5 eV); meanwhile, [Ag32(C
C–CH3)23]+ has a larger thermodynamic barrier for H2 formation from adsorbed H* (0.51 eV) compared to [Ag32(P2C2H6)5(SCH3)23]− (0.05 eV), indicating a higher CO selectivity. This study indicates that, without the presence of an easy stripping ligand (e.g. halogen), with a similar metal kernel, the alkynyl-protected metal nanocluster might have better eCO2RR performance than thiolate counterparts.
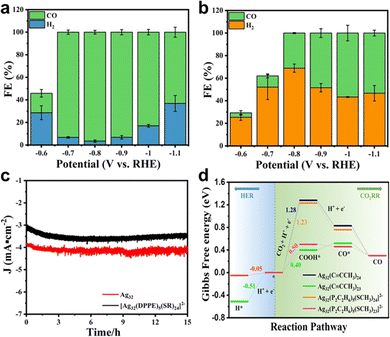 |
| Fig. 12 Faradaic efficiency of CO for (a) Ag32(C CArF)24 and (b) [Ag32(DPPE)5(SR)24]2− at different potentials. (c) Stability testing of two nanoclusters. (d) Comparison of ΔG of eCO2RR on Ag32(C CCH3)24, Ag32(C CCH3)23, [Ag32(P2C2H6)5(SCH3)24]2− and [Ag32(P2C2H6)5(SCH3)23]2− with the HER. Copyright 2022, Springer.37 | |
3.3.3. Other factors.
There are other factors such as the metal–ligand motif arrangement can also affect the eCO2RR performance. Recently, Zhu and Pei groups reported the evolution from superatomic Au24Ag20 monomers into molecular-like Au43Ag38 dimeric nanoclusters, whereas the monomeric and dimeric nanoclusters exhibited markedly different eCO2RR performance.75 Specifically, Au24Ag20(C12H13)24Cl2 (abbreviated as Au24Ag20-1) was first obtained and it can transform into Au43Ag38(C12H13)36Cl12 (abbreviated as Au43Ag38-1), while Au24Ag20(C9H7)24Cl2 (denoted as Au24Ag20-2) was acquired and it was further converted into Au43Ag38(C9H7)36Cl9 (denoted as Au43Ag38-2). The two Au24Ag20 monomer has the identical Au12@Ag20 metal kernel, which undergoes a self-assembly pathway to form a Au12@Ag19–Ag–Au12@Ag19 kernel, and forms two dimeric Au43Ag38 nanoclusters eventually (Fig. 13a). As shown in Fig. 13b, the monomers had higher current density than the dimers, while the CO partial current density followed the descending order of Au24Ag20-1 > Au24Ag20-2 > Au43Ag38-1 > Au43Ag38-2 (Fig. 13c). Notably, the monomers exhibited much higher FECO than the dimers in the potential range from −0.4 to −0.8 V (Fig. 13d and e). The authors ascribed the different catalytic performances to the atomic-packing structures (individual-core vs. dual-core) and surface motif arrangements (parallel vs. crossed).
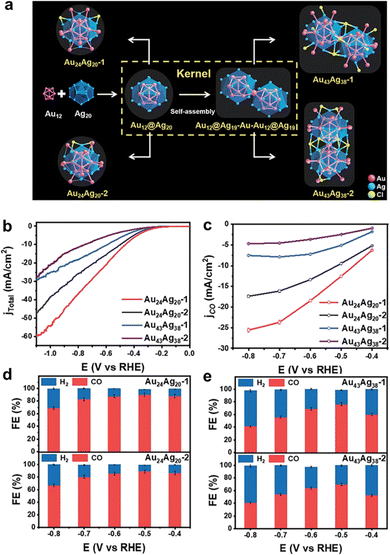 |
| Fig. 13 (a) Structural anatomy of four nanoclusters. (b) LSV curves and (c) the corresponding CO partial current density of the Au24Ag20 and Au43Ag38 nanoclusters. Faradaic efficiency for CO2RR products obtained on (d) Au24Ag20 and (e) Au43Ag38 nanoclusters. Copyright 2022, Royal Society of Chemistry.75 | |
4. Challenges and perspectives
In this review, we highlight the uniqueness of employing alkynyl molecules to prepare Au and Ag nanoclusters with atomic precision. Compared to thiolate molecules, the versatile binding motifs between alkynyl molecules and Au/Ag atoms facilitate the alkynyl-protected nanoclusters to possess drastically different optical properties, geometric configurations, and electronic structures. More intriguingly, significantly different catalytic performances can be achieved. The metal core effect and the surface ligand effect of the all-alkynyl-protected Au and Ag nanoclusters toward the eCO2RR are discussed, demonstrating their great potential to modulate the performance through metal exchange or ligand shell engineering. Some conclusions and future perspectives can be drawn from the above discussions:
(1) Developing efficient synthetic methods with high yield to obtain more atomically precise alkynyl-protected metal nanoclusters is still highly desirable. Currently, in the case of Au and Ag, only Au22,51 Au23,52 Au36,47 Au50,30 Au67,98 Au99,53 Au11056 and Ag15,89 Ag32,37 Ag51,43 and Ag7499 clusters with crystal structures have been documented. The case for Cu,100 AuCu,101 AgCu,94 AuPd,63 AuPt45 and AgPt102 is quite rare, and some (e.g. AgPd) are even missing. The family members of homoleptic alkynyl-protected metal nanoclusters need to be enriched. The direct reduction method is quite straightforward, but to control the monodispersity of the precursor is critical and deserves more research efforts. A synchronous nucleation and passivation strategy has proved its applicability for preparing single metal and some bimetallic nanoclusters with molecular purity, but more cases and examples need to be explored to confirm its generality. For ligand exchange and the one-pot method, more precise reaction condition control (e.g. the choice of introducing a ligand, the temperature, the concentration of the reactant, and the post-reaction purification) is essential to achieve precise synthesis;
(2) To obtain more valuable products from the eCO2RR, synthesizing a Cu nanocluster or a Cu-alloy nanocluster is indispensable hence holds great potential. With Au and Ag nanoclusters as catalysts, CO is formed with high selectivity, and in the presence of Cu, HCO2H can be acquired. So far, no C2+ products have been documented by coinage metal nanoclusters with atomic precision, regardless of the ligand type. In the presence of a hydride ligand for Cu nanoclusters, the negatively charged hydride plays a critical role in determining the selectivity, where HCO2H is formed via the lattice-hydride mechanism.103 The above case of all-alkynyl-protected Cu alloy clusters (Ag9Cu6, Au2Ag8Cu5) shows that the HCO2H formation occurs through a rather different proton-hydride mechanism or hydride-proton mechanism. Obtaining all-alkynyl-protected Cu nanoclusters for the eCO2RR application would be of particular interest to advance the fundamental understanding of the eCO2RR mechanism but still remains challenging, and Cu based bimetallic or trimetallic nanoclusters are also hoped to promote the activity and selectivity as well as to improve the mechanistic understanding.
(3) Advanced in situ techniques are urgently needed to examine the eCO2RR process for gaining more mechanistic insights. For instance, DFT calculations predict one ligand stripping to expose undercoordinated metal atoms as the active site but losing several ligands might not necessarily favour the eCO2RR reaction for the Ag15 cluster, and in situ techniques such as surface enhanced Raman scattering, infrared spectroscopy, high energy X-ray diffraction (XRD), extended X-ray absorption fine structure (EXAFS),104 X-ray absorption near-edge structure (XANES),104 small-angle neutron scattering (SANS)105etc. can provide some key structural information to confirm that. In addition, the in situ monitoring of the structural evolution of the catalyst during the eCO2RR process may detect the metal–carbon cleavage, the adsorption configuration of CO2 molecules on metal nanoclusters, the interaction between the cluster and the electrolyte, the real active site, the evolution of the key intermediates, and the formation process of the product more accurately.
In summary, with the advancement of synthetic methods, more molecular homoleptic alkynyl-protected metal nanoclusters with precise structures are anticipated to be discovered. The unique surface binding motifs and different physicochemical properties of the all-alkynyl-protected coinage metal nanoclusters can bring profound and valuable mechanistic insights of the eCO2RR. We envision that increasing research efforts should be dedicated to this fundamentally interesting yet important field.
Conflicts of interest
There are no conflicts to declare.
Acknowledgements
Z. T. acknowledges the financial support from Natural Science Foundation of Guangdong Province (No. 2022A1515011840).
Notes and references
- L. Z. He and T. T. Dong, Progress in Controlling the Synthesis of Atomically Precise Silver Nanoclusters, CrystEngComm, 2021, 23, 7369–7379 RSC
.
- R. C. Jin, C. J. Zeng, M. Zhou and Y. X. Chen, Atomically Precise Colloidal Metal Nanoclusters and Nanoparticles: Fundamentals and Opportunities, Chem. Rev., 2016, 116, 10346–10413 CrossRef CAS PubMed
.
- I. Chakraborty and T. Pradeep, Atomically Precise Clusters of Noble Metals: Emerging Link between Atoms and Nanoparticles, Chem. Rev., 2017, 117, 8208–8271 CrossRef CAS PubMed
.
- T. Kawawaki, Y. Negishi and H. Kawasaki, Photo/Electrocatalysis and Photosensitization Using Metal Nanoclusters for Green Energy and Medical Applications, Nanoscale Adv., 2020, 2, 17–36 RSC
.
- K. Kwak and D. Lee, Electrochemistry of Atomically Precise Metal Nanoclusters, Acc. Chem. Res., 2019, 52, 12–22 CrossRef CAS PubMed
.
- Y. M. Wang, J. Cai, Q. Y. Wang, Y. Li, Z. Han, S. Li, C. H. Gong, S. Wang, S. Q. Zang and T. C. W. Mak, Electropolymerization of Metal Clusters Establishing a Versatile Platform for Enhanced Catalysis Performance, Angew. Chem., Int. Ed., 2022, 61, e202114538 CAS
.
- A. K. Deb, B. Biswas, R. Naidu and M. M. Rahman, Mechanistic Insights of Hexavalent Chromium Remediation by Halloysite-Supported Copper Nanoclusters, J. Hazard. Mater., 2022, 421, 126812 CrossRef CAS PubMed
.
- V. Subramanian, S. Jena, D. Ghosh, M. Jash, A. Baksi, D. Ray and T. Pradeep, Dual Probe Sensors Using Atomically Precise Noble Metal Clusters, ACS Omega, 2017, 2, 7576–7583 CrossRef CAS PubMed
.
- B. J. Shi, L. Shang, W. Zhang, L. P. Jia, R. N. Ma, Q. W. Xue and H. S. Wang, Electrochemical Stripping Chemiluminescent Sensor Based on Copper Nanoclusters for Detection of Carcinoembryonic Antigen, Sens. Actuators, B, 2021, 344, 130291 CrossRef CAS
.
- C. Zhang, X. B. Gao, W. R. Chen, M. He, Y. Yu, G. B. Gao and T. L. Sun, Advances of Gold Nanoclusters for Bioimaging, iScience, 2022, 25, 105022 CrossRef CAS PubMed
.
- T. T. Jia, G. Yang, S. J. Mo, Z. Y. Wang, B. J. Li, W. Ma, Y. X. Guo, X. Chen, X. Zhao, J. Q. Liu and S. Q. Zang, Atomically Precise Gold-Levonorgestrel Nanocluster as a Radiosensitizer for Enhanced Cancer Therapy, ACS Nano, 2019, 13, 8320–8328 CrossRef CAS PubMed
.
- H. G. Zhu, N. W. Liu, Z. P. Wang, Q. Xue, Q. Wang, X. M. Wang, Y. Liu, Z. M. Yin and X. Yuan, Marrying Luminescent Au Nanoclusters to TiO2 for Visible-Light-Driven Antibacterial Application, Nanoscale, 2021, 13, 18996–19003 RSC
.
- N. W. Liu, Y. C. Wang, Z. P. Wang, Q. X. He, Y. Liu, X. Y. Dou, Z. M. Yin, Y. Li, H. G. Zhu and X. Yuan, Conjugating AIE-Featured AuAg Nanoclusters with Highly Luminescent Carbon Dots for Improved Visible-Light-Driven Antibacterial Activity, Nanoscale, 2022, 14, 8183–8191 RSC
.
- W. J. Du, S. Jin, L. Xiong, M. Chen, J. Zhang, X. J. Zou, Y. Pei, S. X. Wang and M. Z. Zhu, Ag50(Dppm)6(SR)30 and Its Homologue AuxAg50-x(Dppm)6(SR)30 Alloy Nanocluster: Seeded Growth, Structure Determination, and Differences in Properties, J. Am. Chem. Soc., 2017, 139, 1618–1624 CrossRef CAS PubMed
.
- M. S. Bootharaju, H. Chang, G. C. Deng, S. Malola, W. Baek, H. Hakkinen, N. F. Zheng and T. Hyeon, Cd12Ag32(SePh)36: Non-Noble Metal Doped Silver Nanoclusters, J. Am. Chem. Soc., 2019, 141, 8422–8425 CrossRef CAS PubMed
.
- H. Hirai, S. Takano, T. Nakamura and T. Tsukuda, Understanding Doping Effects on Electronic Structures of Gold Superatoms: A Case Study of Diphosphine-Protected M@Au12 (M = Au, Pt, Ir), Inorg. Chem., 2020, 59, 17889–17895 CrossRef CAS PubMed
.
- S. Takano, H. Hirai, S. Muramatsu and T. Tsukuda, Hydride-Doped Gold Superatom (Au9H)2+: Synthesis, Structure, and Transformation, J. Am. Chem. Soc., 2018, 140, 8380–8383 CrossRef CAS PubMed
.
- S. L. Zhuang, L. W. Liao, M. B. Li, C. H. Yao, Y. Zhao, H. W. Dong, J. Li, H. T. Deng, L. L. Li and Z. K. Wu, The fcc Structure Isomerization in Gold Nanoclusters, Nanoscale, 2017, 9, 14809–14813 RSC
.
- Q. Zhou, S. Kaappa, S. Malola, H. Lu, D. Guan, Y. J. Li, H. C. Wang, Z. X. Xie, Z. B. Ma, H. Häkkinen, N. F. Zheng, X. M. Yang and L. S. Zheng, Real-Space Imaging with Pattern Recognition of a Ligand-Protected Ag374 Nanocluster at Sub-Molecular Resolution, Nat. Commun., 2018, 9, 2948 CrossRef PubMed
.
- Y. X. Du, H. T. Sheng, D. Astruc and M. Z. Zhu, Atomically Precise Noble Metal Nanoclusters as Efficient Catalysts: A Bridge between Structure and Properties, Chem. Rev., 2020, 120, 526–622 CrossRef CAS PubMed
.
- Y. T. Cao, T. K. Chen, Q. F. Yao and J. P. Xie, Diversification of Metallic Molecules through Derivatization Chemistry of Au25 Nanoclusters, Acc. Chem. Res., 2021, 54, 4142–4153 CrossRef CAS PubMed
.
- L. S. Luo, Z. Y. Liu, X. S. Du and R. C. Jin, Near-Infrared Dual Emission from the Au42(SR)32 Nanocluster and Tailoring of Intersystem Crossing, J. Am. Chem. Soc., 2022, 144, 19243–19247 CrossRef CAS PubMed
.
- M. S. Bootharaju, S. Lee, G. Deng, S. Malola, W. Baek, H. Hakkinen, N. Zheng and T. Hyeon, Ag44(EBT)26(TPP)4 Nanoclusters with Tailored Molecular and Electronic Structure, Angew. Chem., Int. Ed., 2021, 60, 9038–9044 CrossRef CAS PubMed
.
- X. Q. Liang, Y. Z. Li, Z. Wang, S. S. Zhang, Y. C. Liu, Z. Z. Cao, L. Feng, Z. Y. Gao, Q. W. Xue, C. H. Tung and D. Sun, Revealing the Chirality Origin and Homochirality Crystallization of Ag14 Nanocluster at the Molecular Level, Nat. Commun., 2021, 12, 4966 CrossRef CAS PubMed
.
- A. K. Das, S. Mukherjee, A. S. Nair, S. Bhandary, D. Chopra, D. Sanyal, B. Pathak and S. Mandal, Defects Engineering on Ceria and C–C Coupling Reactions Using [Au11(PPh3)7I3] Nanocluster: A Combined Experimental and Theoretical Study, ACS Nano, 2020, 14, 16681–16688 CrossRef CAS PubMed
.
- H. Shen, S. J. Xiang, Z. Xu, C. Liu, X. H. Li, C. F. Sun, S. C. Lin, B. K. Teo and N. F. Zheng, Superatomic Au13 Clusters Ligated by Different N-Heterocyclic Carbenes and Their Ligand-Dependent Catalysis, Photoluminescence, and Proton Sensitivity, Nano Res., 2020, 13, 1908–1911 CrossRef CAS
.
- M. R. Narouz, K. M. Osten, P. J. Unsworth, R. W. Y. Man, K. Salorinne, S. Takano, R. Tomihara, S. Kaappa, S. Malola, C. T. Dinh, J. D. Padmos, K. Ayoo, P. J. Garrett, M. Nambo, J. H. Horton, E. H. Sargent, H. Hakkinen, T. Tsukuda and C. M. Crudden, N-Heterocyclic Carbene-Functionalized Magic-Number Gold Nanoclusters, Nat. Chem., 2019, 11, 419–425 CrossRef CAS PubMed
.
- S. F. Yuan, C. Q. Xu, W. D. Liu, J. X. Zhang, J. Li and Q. M. Wang, Rod-Shaped Silver Supercluster Unveiling Strong Electron Coupling between Substituent Icosahedral Units, J. Am. Chem. Soc., 2021, 143, 12261–12267 CrossRef CAS PubMed
.
- S. F. Yuan, W. D. Liu, C. Y. Liu, Z. J. Guan and Q. M. Wang, Nitrogen Donor Protection for Atomically Precise Metal Nanoclusters, Chem. – Eur. J., 2022, 28, e202104445 CAS
.
- Z. J. Guan, F. Hu, J. J. Li, Z. R. Liu and Q. M. Wang, Homoleptic Alkynyl-Protected Gold Nanoclusters with Unusual Compositions and Structures, Nanoscale, 2020, 12, 13346–13350 RSC
.
- W. D. Liu, J. Q. Wang, S. F. Yuan, X. Chen and Q. M. Wang, Chiral Superatomic Nanoclusters Ag47 Induced by the Ligation of Amino Acids, Angew. Chem., Int. Ed., 2021, 60, 11430–11435 CrossRef CAS PubMed
.
- F. Hu, J. J. Li, Z. J. Guan, S. F. Yuan and Q. M. Wang, Formation of an Alkynyl-Protected Ag112 Silver Nanocluster as Promoted by Chloride Released In Situ from CH2Cl2, Angew. Chem., Int. Ed., 2020, 59, 5312–5315 CrossRef CAS PubMed
.
- X. S. Ma, Y. Tang, G. Y. Ma, L. B. Qin and Z. H. Tang, Controllable Synthesis and Formation Mechanism Study of Homoleptic Alkynyl-Protected Au Nanoclusters: Recent Advances, Grand Challenges, and Great Opportunities, Nanoscale, 2021, 13, 602–614 RSC
.
- Z. Lei, X. K. Wan, S. F. Yuan, Z. J. Guan and Q. M. Wang, Alkynyl Approach toward the Protection of Metal Nanoclusters, Acc. Chem. Res., 2018, 51, 2465–2474 CrossRef CAS PubMed
.
- Z. Lei, X. K. Wan, S. F. Yuan, J. Q. Wang and Q. M. Wang, Alkynyl-Protected Gold and Gold-Silver Nanoclusters, Dalton Trans., 2017, 46, 3427–3434 RSC
.
- E. Wang, W. W. Xu, B. Zhu and Y. Gao, Understanding the Chemical Insights of Staple Motifs of Thiolate-Protected Gold Nanoclusters, Small, 2021, 17, e2001836 CrossRef PubMed
.
- L. Y. Chen, F. Sun, Q. L. Shen, L. B. Qin, Y. G. Liu, L. Qiao, Q. Tang, L. K. Wang and Z. H. Tang, Homoleptic Alkynyl-Protected Ag32 Nanocluster with Atomic Precision: Probing the Ligand effect toward CO2 Electroreduction and 4-Nitrophenol Reduction, Nano Res., 2022, 15, 8908–8913 CrossRef CAS
.
- J. J. Li, Z. J. Guan, Z. Lei, F. Hu and Q. M. Wang, Same Magic Number but Different Arrangement: Alkynyl-Protected Au25 with D3 symmetry, Angew. Chem., Int. Ed., 2019, 58, 1083–1087 CrossRef CAS PubMed
.
- X. K. Wan, Z. J. Guan and Q. M. Wang, Homoleptic Alkynyl-Protected Gold Nanoclusters: Au44(PhC
C)28 and Au36(PhC
C)24, Angew. Chem., Int. Ed., 2017, 56, 11494–11497 CrossRef CAS PubMed
.
- M. Sugiuchi, Y. Shichibu, T. Nakanishi, Y. Hasegawa and K. Konishi, Cluster-π Electronic Interaction in a Superatomic Au13 Cluster Bearing σ-Bonded Acetylide Ligands, Chem. Commun., 2015, 51, 13519–13522 RSC
.
- X. S. Han, X. Luan, H. F. Su, J. J. Li, S. F. Yuan, Z. Lei, Y. Pei and Q. M. Wang, Structure Determination of Alkynyl-Protected Gold Nanocluster Au22(tBuC
C)18 and Its Thermochromic Luminescence, Angew. Chem., Int. Ed., 2020, 59, 2309–2312 CrossRef CAS PubMed
.
- M. M. Zhang, X. Y. Dong, Z. Y. Wang, X. M. Luo, J. H. Huang, S. Q. Zang and T. C. Mak, Alkynyl-Stabilized Superatomic Silver Clusters Showing Circularly Polarized Luminescence, J. Am. Chem. Soc., 2021, 143, 6048–6053 CrossRef CAS PubMed
.
- G. X. Duan, L. Tian, J. B. Wen, L. Y. Li, Y. P. Xie and X. Lu, An Atomically Precise All-Tert-Butylethynide-Protected Ag51 Superatom Nanocluster with Color Tunability, Nanoscale, 2018, 10, 18915–18919 RSC
.
- X. K. Wan, J. Q. Wang, Z. A. Nan and Q. M. Wang, Ligand Effects in Catalysis by Atomically Precise Gold Nanoclusters, Sci. Adv., 2017, 3, e1701823 CrossRef PubMed
.
- X. Li, S. Takano and T. Tsukuda, Ligand Effects on the Hydrogen Evolution Reaction Catalyzed by Au13 and Pt@Au12: Alkynyl vs Thiolate, J. Phys. Chem. C, 2021, 125, 23226–23230 CrossRef CAS
.
- Z. Lei, J. J. Li, X. K. Wan, W. H. Zhang and Q. M. Wang, Isolation and Total Structure Determination of an All-Alkynyl-Protected Gold Nanocluster Au144, Angew. Chem., Int. Ed., 2018, 57, 8639–8643 CrossRef CAS PubMed
.
- X. S. Ma, G. Y. Ma, L. B. Qin, G. X. Chen, S. W. Chen and Z. H. Tang, A Synchronous Nucleation and Passivation Strategy for Controllable Synthesis of Au36(PA)24: Unveiling the Formation Process and the Role of Au22(PA)18 Intermediate, Sci. China: Chem., 2020, 63, 1777–1784 CrossRef CAS
.
- G. Y. Ma, Y. Tang, L. Y. Chen, L. B. Qin, Q. L. Shen, L. K. Wang and Z. H. Tang, A Homoleptic Alkynyl-Protected Au(I)9–Ag(I)9 Cluster: Structure Analysis, Optical Properties, and Catalytic Implications, Eur. J. Inorg. Chem., 2022, e202200176 CAS
.
- K. Konishi, M. Iwasaki, M. Sugiuchi and Y. Shichibu, Ligand-Based Toolboxes for Tuning of the Optical Properties of Subnanometer Gold Clusters, J. Phys. Chem. Lett., 2016, 7, 4267–4274 CrossRef CAS PubMed
.
- N. Kobayashi, Y. Kamei, Y. Shichibu and K. Konishi, Protonation-Induced Chromism of Pyridylethynyl-Appended [core + exo]-Type Au8 Clusters. Resonance-Coupled Electronic Perturbation through π-Conjugated Group, J. Am. Chem. Soc., 2013, 135, 16078–16081 CrossRef CAS PubMed
.
- S. Ito, S. Takano and T. Tsukuda, Alkynyl-Protected Au22(C
CR)18 Clusters Featuring New Interfacial Motifs and R-Dependent Photoluminescence, J. Phys. Chem. Lett., 2019, 10, 6892–6896 CrossRef CAS PubMed
.
- Z. J. Guan, F. Hu, J. J. Li, Z. R. Wen, Y. M. Lin and Q. M. Wang, Isomerization in Alkynyl-Protected Gold Nanoclusters, J. Am. Chem. Soc., 2020, 142, 2995–3001 CrossRef CAS PubMed
.
- J. J. Li, Z. K. Liu, Z. J. Guan, X. S. Han, W. Q. Shi and Q. M. Wang, A 59-Electron Non-Magic-Number Gold Nanocluster Au99(C
CR)40 Showing Unexpectedly High Stability, J. Am. Chem. Soc., 2022, 144, 690–694 CrossRef CAS PubMed
.
- X. S. Ma, Z. H. Tang, L. B. Qin, J. Peng, L. G. Li and S. W. Chen, Unravelling the Formation Mechanism of Alkynyl Protected Gold Clusters: A Case Study of Phenylacetylene Stabilized Au144 Molecules, Nanoscale, 2020, 12, 2980–2986 RSC
.
- S. F. Yuan, P. Li, Q. Tang, X. K. Wan, Z. A. Nan, D. E. Jiang and Q. M. Wang, Alkynyl-Protected Silver Nanoclusters Featuring an Anticuboctahedral Kernel, Nanoscale, 2017, 9, 11405–11409 RSC
.
- J. Q. Wang, S. Shi, R. L. He, S. F. Yuan, G. Y. Yang, G. J. Liang and Q. M. Wang, Total Structure Determination of the Largest Alkynyl-Protected fcc Gold Nanocluster Au110 and the Study on Its Ultrafast Excited-State Dynamics, J. Am. Chem. Soc., 2020, 142, 18086–18092 CrossRef CAS PubMed
.
- Y. Wang, X. K. Wan, L. T. Ren, H. F. Su, G. Li, S. Malola, S. C. Lin, Z. C. Tang, H. Hakkinen, B. K. Teo, Q. M. Wang and N. F. Zheng, Atomically Precise Alkynyl-Protected Metal Nanoclusters as a Model Catalyst: Observation of Promoting Effect of Surface Ligands on Catalysis by Metal Nanoparticles, J. Am. Chem. Soc., 2016, 138, 3278–3281 CrossRef CAS PubMed
.
- M. Z. Zhu, E. Lanni, N. Garg, M. K. Bier and R. C. Jin, Kinetically Controlled, High-Yield Synthesis of Au25 Clusters, J. Am. Chem. Soc., 2008, 130, 1138–1139 CrossRef CAS PubMed
.
- X. Kang and M. Z. Zhu, Transformation of Atomically Precise Nanoclusters by Ligand-Exchange, Chem. Mater., 2019, 31, 9939–9969 CrossRef CAS
.
- W. Suzuki, R. Takahata, Y. Chiga, S. Kikkawa, S. Yamazoe, Y. Mizuhata, N. Tokitoh and T. Teranishi, Control over Ligand-Exchange Positions of Thiolate-Protected Gold Nanoclusters Using Steric Repulsion of Protecting Ligands, J. Am. Chem. Soc., 2022, 144, 12310–12320 CrossRef CAS PubMed
.
- Y. Zeng, S. Havenridge, M. Gharib, A. Baksi, K. L. D. M. Weerawardene, A. R. Ziefuss, C. Strelow, C. Rehbock, A. Mews, S. Barcikowski, M. M. Kappes, W. J. Parak, C. M. Aikens and I. Chakraborty, Impact of Ligands on Structural and Optical Properties of Ag29 Nanoclusters, J. Am. Chem. Soc., 2021, 143, 9405–9414 CrossRef CAS PubMed
.
- P. Maity, H. Tsunoyama, M. Yamauchi, S. Xie and T. Tsukuda, Organogold Clusters Protected by Phenylacetylene, J. Am. Chem. Soc., 2011, 133, 20123–20125 CrossRef CAS PubMed
.
- S. Takano, S. Ito and T. Tsukuda, Efficient and Selective Conversion of Phosphine-Protected (MAu8)2+ (M = Pd, Pt) Superatoms to Thiolate-Protected (MAu12)6+ or Alkynyl-Protected (MAu12)4+ Superatoms via Hydride Doping, J. Am. Chem. Soc., 2019, 141, 15994–16002 CrossRef CAS PubMed
.
- C. A. Hosier, I. D. Anderson and C. J. Ackerson, Acetylide-for-Thiolate and Thiolate-for-Acetylide Exchange on Gold Nanoclusters, Nanoscale, 2020, 12, 6239–6242 RSC
.
- Y. B. Ma, J. Wang, J. L. Yu, J. W. Zhou, X. C. Zhou, H. X. Li, Z. He, H. W. Long, Y. H. Wang, P. Y. Lu, J. W. Yin, H. Y. Sun, Z. C. Zhang and Z. X. Fan, Surface Modification of Metal Materials for High-Performance Electrocatalytic Carbon Dioxide Reduction, Matter, 2021, 4, 888–926 CrossRef CAS
.
- Z. Xin, J. Liu, X. Wang, K. Shen, Z. Yuan, Y. Chen and Y. Q. Lan, Implanting Polypyrrole in Metal-Porphyrin MOFs: Enhanced Electrocatalytic Performance for CO2RR, ACS Appl. Mater. Interfaces, 2021, 13, 54959–54966 CrossRef CAS PubMed
.
- L. B. Qin, G. Y. Ma, L. K. Wang and Z. H. Tang, Atomically Precise Metal Nanoclusters for (Photo)Electroreduction of CO2: Recent Advances, Challenges and Opportunities, J. Energy Chem., 2021, 57, 359–370 CrossRef CAS
.
- Y. B. Ma, J. L. Yu, M. Z. Sun, B. Chen, X. C. Zhou, C. L. Ye, Z. Q. Guan, W. H. Guo, G. Wang, S. Y. Lu, D. S. Xia, Y. H. Wang, Z. He, L. Zheng, Q. B. Yun, L. Q. Wang, J. W. Zhou, P. Y. Lu, J. W. Yin, Y. F. Zhao, Z. B. Luo, L. Zhai, L. W. Liao, Z. L. Zhu, R. Q. Ye, Y. Chen, Y. Lu, S. B. Xi, B. L. Huang, C. S. Lee and Z. X. Fan, Confined Growth of Silver-Copper Janus Nanostructures with {100} Facets for Highly Selective Tandem Electrocatalytic Carbon Dioxide Reduction, Adv. Mater., 2022, 34, e2110607 CrossRef PubMed
.
- B. Xiong, J. Liu, Y. J. Yang, Y. C. Yang and Z. X. Hua, Effect Mechanism of NO on Electrocatalytic Reduction of CO2 to CO over Pd@Cu Bimetal Catalysts, Fuel, 2022, 323, 124339 CrossRef CAS
.
- M. M. Ayyub and C. N. R. Rao, Designing Electrode Materials for the Electrochemical Reduction of Carbon Dioxide, Mater. Horiz., 2021, 8, 2420–2443 RSC
.
- X. Z. Lin, W. G. Ma, K. J. Sun, B. Sun, X. M. Fu, X. Q. Ren, C. Liu and J. H. Huang, [AuAg26(SR)18S]− Nanocluster: Open Shell Structure and High faradaic Efficiency in Electrochemical Reduction of CO2 to CO, J. Phys. Chem. Lett., 2021, 12, 552–557 CrossRef CAS PubMed
.
- W. Choi, H. Seong, V. Efremov, Y. Lee, S. Im, D. H. Lim, J. S. Yoo and D. Lee, Controlled Syngas Production by Electrocatalytic CO2 Reduction on Formulated Au25(SR)18 and PtAu24(SR)18 Nanoclusters, J. Chem. Phys., 2021, 155, 014305 CrossRef CAS PubMed
.
- Z. H. Gao, K. Wei, T. Wu, J. Dong, D. E. Jiang, S. Sun and L. S. Wang, A Heteroleptic Gold Hydride Nanocluster for Efficient and Selective Electrocatalytic Reduction of CO2 to CO, J. Am. Chem. Soc., 2022, 144, 5258–5262 CrossRef CAS PubMed
.
- V. K. Kulkarni, B. N. Khiarak, S. Takano, S. Malola, E. L. Albright, T. I. Levchenko, M. D. Aloisio, C. T. Dinh, T. Tsukuda, H. Hakkinen and C. M. Crudden, N-Heterocyclic Carbene-Stabilized Hydrido Au24 Nanoclusters: Synthesis, Structure, and Electrocatalytic Reduction of CO2, J. Am. Chem. Soc., 2022, 144, 9000–9006 CrossRef CAS PubMed
.
- J. Y. Xu, L. Xiong, X. Cai, S. S. Tang, A. C. Tang, X. Liu, Y. Pei and Y. Zhu, Evolution from Superatomic Au24Ag20 Monomers into Molecular-Like Au43Ag38 Dimeric Nanoclusters, Chem. Sci., 2022, 13, 2778–2782 RSC
.
- S. S. Tang, J. Y. Xu, X. Liu and Y. Zhu, Ag Doped Au44 Nanoclusters for Electrocatalytic Conversion of CO2 to CO, Chem. – Eur. J., 2022, 28, e202201262 CAS
.
- D. R. Kauffman, D. Alfonso, C. Matranga, H. F. Qian and R. C. Jin, Experimental and Computational Investigation of Au25 Clusters and CO2: A Unique Interaction and Enhanced Electrocatalytic Activity, J. Am. Chem. Soc., 2012, 134, 10237–10243 CrossRef CAS PubMed
.
- S. Li, D. Alfonso, A. V. Nagarajan, S. D. House, J. C. Yang, D. R. Kauffman, G. Mpourmpakis and R. C. Jin, Monopalladium Substitution in Gold Nanoclusters Enhances CO2 Electroreduction Activity and Selectivity, ACS Catal., 2020, 10, 12011–12016 CrossRef CAS
.
- S. Zhao, N. Austin, M. Li, Y. B. Song, S. D. House, S. Bernhard, J. C. Yang, G. Mpourmpakis and R. C. Jin, Influence of Atomic-Level Morphology on Catalysis: The Case of Sphere and Rod-Like Gold Nanoclusters for CO2 Electroreduction, ACS Catal., 2018, 8, 4996–5001 CrossRef CAS
.
- D. R. Kauffman, D. Alfonso, C. Matranga, P. Ohodnicki, X. Y. Deng, R. C. Siva, C. J. Zeng and R. C. Jin, Probing Active Site Chemistry with Differently Charged Au25q Nanoclusters (q = −1, 0, +1), Chem. Sci., 2014, 5, 3151–3157 RSC
.
- H. Seong, V. Efremov, G. Park, H. Kim, J. S. Yoo and D. Lee, Atomically Precise Gold Nanoclusters as Model Catalysts for Identifying Active Sites for Electroreduction of CO2, Angew. Chem., Int. Ed., 2021, 60, 14563–14570 CrossRef CAS PubMed
.
- S. L. Zhuang, D. Chen, L. W. Liao, Y. Zhao, N. Xia, W. H. Zhang, C. M. Wang, J. Yang and Z. K. Wu, Hard-Sphere Random Close-Packed Au47Cd2(TBBT)31 Nanoclusters with a faradaic Efficiency of Up to 96% for Electrocatalytic CO2 Reduction to CO, Angew. Chem., Int. Ed., 2020, 59, 3073–3077 CrossRef CAS PubMed
.
- Y. N. Sun, X. Liu, K. Xiao, Y. Zhu and M. Y. Chen, Active-Site Tailoring
of Gold Cluster Catalysts for Electrochemical CO2 Reduction, ACS Catal., 2021, 11, 11551–11560 CrossRef CAS
.
- N. Austin, S. Zhao, J. R. McKone, R. C. Jin and G. Mpourmpakis, Elucidating the Active Sites for CO2 Electroreduction on Ligand-Protected Au25 Nanoclusters, Catal. Sci. Technol., 2018, 8, 3795–3805 RSC
.
- X. K. Wan, J. Q. Wang and Q. M. Wang, Ligand-Protected Au55 with a Novel Structure and Remarkable CO2 Electroreduction Performance, Angew. Chem., Int. Ed., 2021, 60, 20748–20753 CrossRef CAS PubMed
.
- S. F. Yuan, R. L. He, X. S. Han, J. Q. Wang, Z. J. Guan and Q. M. Wang, Robust Gold Nanocluster Protected with Amidinates for Electrocatalytic CO2 Reduction, Angew. Chem., Int. Ed., 2021, 60, 14345–14349 CrossRef CAS PubMed
.
- D. Yang, J. W. Wang, Q. J. Wang, Z. T. Yuan, Y. H. Dai, C. M. Zhou, X. Y. Wan, Q. C. Zhang and Y. H. Yang, Electrocatalytic CO2 Reduction over Atomically Precise Metal Nanoclusters Protected by Organic Ligands, ACS Nano, 2022, 16, 15681–15704 CrossRef CAS PubMed
.
- S. Zhao, R. X. Jin and R. C. Jin, Opportunities and Challenges in CO2 Reduction by Gold- and Silver-Based Electrocatalysts: From Bulk Metals to Nanoparticles and Atomically Precise Nanoclusters, ACS Energy Lett., 2018, 3, 452–462 CrossRef CAS
.
- L. B. Qin, F. Sun, X. S. Ma, G. Y. Ma, Y. Tang, L. K. Wang, Q. Tang, R. C. Jin and Z. H. Tang, Homoleptic Alkynyl-Protected Ag15 Nanocluster with Atomic Precision: Structural Analysis and Electrocatalytic Performance toward CO2 Reduction, Angew. Chem., Int. Ed., 2021, 60, 26136–26141 CrossRef CAS PubMed
.
- A. Ghosh, O. F. Mohammed and O. M. Bakr, Atomic-Level Doping of Metal Clusters, Acc. Chem. Res., 2018, 51, 3094–3103 CrossRef CAS PubMed
.
- S. X. Wang, Q. Li, X. Kang and M. Z. Zhu, Customizing the Structure, Composition, and Properties of Alloy Nanoclusters by Metal Exchange, Acc. Chem. Res., 2018, 51, 2784–2792 CrossRef CAS PubMed
.
- Z. B. Gan, N. Xia and Z. K. Wu, Discovery, Mechanism, and Application of Antigalvanic Reaction, Acc. Chem. Res., 2018, 51, 2774–2783 CrossRef CAS PubMed
.
- Y. Wang, H. F. Su, L. T. Ren, S. Malola, S. C. Lin, B. K. Teo, H. Hakkinen and N. F. Zheng, Site Preference in Multimetallic Nanoclusters:Incorporation of Alkali Metal Ions or Copper Atoms into the Alkynyl-Protected BodyCentered Cubic Cluster [Au7Ag8(C
CtBu)12], Angew. Chem., Int. Ed., 2016, 55, 15152–15156 CrossRef CAS PubMed
.
- X. S. Ma, L. Xiong, L. B. Qin, Y. Tang, G. Y. Ma, Y. Pei and Z. H. Tang, A Homoleptic Alkynyl-Protected [Ag9Cu6(tBuC
C)12]+ Superatom with Free Electrons: Synthesis, Structure Analysis, and Different Properties Compared with the Au7Ag8 Cluster in the M15+ Series, Chem. Sci., 2021, 12, 12819–12826 RSC
.
- X. S. Ma, F. Sun, L. B. Qin, Y. G. Liu, X. W. Kang, L. K. Wang, D. E. Jiang, Q. Tang and Z. H. Tang, Electrochemical CO2 Reduction Catalyzed by Atomically Precise Alkynyl-Protected Au7Ag8, Ag9Cu6, and Au2Ag8Cu5 Nanoclusters: Probing the Effect of Multi-Metal Core on Selectivity, Chem. Sci., 2022, 13, 10149–10158 RSC
.
- S. Li, A. V. Nagarajan, Y. Li, D. R. Kauffman, G. Mpourmpakis and R. C. Jin, The Role of Ligands in Atomically Precise Nanocluster-Catalyzed CO2 Electrochemical Reduction, Nanoscale, 2021, 13, 2333–2337 RSC
.
- J. Wang, F. Xu, Z. Y. Wang, S. Q. Zang and T. C. W. Mak, Ligand-Shell Engineering of a Au28 Nanocluster Boosts Electrocatalytic CO2 Reduction, Angew. Chem., Int. Ed., 2022, 61, e202207492 CAS
.
- J. J. Li, Z. J. Guan, S. F. Yuan, F. Hu and Q. M. Wang, Enriching Structural Diversity of Alkynyl-Protected Gold Nanoclusters with Chlorides, Angew. Chem., Int. Ed., 2021, 60, 6699–6703 CrossRef CAS PubMed
.
- M. Qu, H. Li, L. H. Xie, S. T. Yan, J. R. Li, J. H. Wang, C. Y. Wei, Y. W. Wu and X. M. Zhang, Bidentate Phosphine-Assisted Synthesis of an All-Alkynyl-Protected Ag74 Nanocluster, J. Am. Chem. Soc., 2017, 139, 12346–12349 CrossRef CAS PubMed
.
- B. L. Han, Z. Liu, L. Feng, Z. Wang, R. K. Gupta, C. M. Aikens, C. H. Tung and D. Sun, Polymorphism in Atomically Precise Cu23 Nanocluster Incorporating Tetrahedral [Cu4]0 Kernel, J. Am. Chem. Soc., 2020, 142, 5834–5841 CrossRef CAS PubMed
.
- X. K. Wan, X. L. Cheng, Q. Tang, Y. Z. Han, G. Hu, D. E. Jiang and Q. M. Wang, Atomically Precise Bimetallic Au19Cu30 Nanocluster with an Icosidodecahedral Cu30 Shell and an Alkynyl-Cu Interface, J. Am. Chem. Soc., 2017, 139, 9451–9454 CrossRef CAS PubMed
.
- H. Shen and T. Mizuta, An Alkynyl-Stabilized Pt5Ag22 Cluster Featuring a Two-Dimensional Alkynyl-Platinum “Crucifix Motif”, Chem. – Eur. J., 2017, 23, 17885–17888 CrossRef CAS PubMed
.
- Q. Tang, Y. J. Lee, D. Y. Li, W. J. Choi, C. W. Liu, D. Lee and D. E. Jiang, Lattice-Hydride Mechanism in Electrocatalytic CO2 Reduction by Structurally Precise Copper-Hydride Nanoclusters, J. Am. Chem. Soc., 2017, 139, 9728–9736 CrossRef CAS PubMed
.
- D. M. Chevrier, L. Raich, C. Rovira, A. Das, Z. T. Luo, Q. F. Yao, A. Chatt, J. P. Xie, R. C. Jin, J. Akola and P. Zhang, Molecular-Scale Ligand Effects in Small Gold-Thiolate Nanoclusters, J. Am. Chem. Soc., 2018, 140, 15430–15436 CrossRef CAS PubMed
.
- X. D. Liu, H. Y. Yang, Y. X. Chen, Y. Yang, L. Porcar, A. Radulescu, S. Guldin, R. C. Jin, F. Stellacci and Z. Luo, Quantifying the Solution Structure of Metal Nanoclusters Using Small-Angle Neutron Scattering, Angew. Chem., Int. Ed., 2022, 61, e202209751 CAS
.
|
This journal is © the Partner Organisations 2023 |
Click here to see how this site uses Cookies. View our privacy policy here.