A luminescent cationic MOF and its polymer composite membrane elicit selective sensing of antibiotics and pesticides in water†
Received
14th January 2023
, Accepted 24th July 2023
First published on 24th July 2023
Abstract
Medicines and pesticides are being used excessively, misused, or abused in recent times, resulting in major environmental contamination and, more specifically, water pollution. Remediation of these anthropogenic wastes in ambient water holds the key to mitigating their bioaccumulation and the subsequent negative health impacts. To address this, here, we present a novel stable cationic metal–organic framework (MOF), [Cd(L)2(ClO4)2·xG]n (L = 1,1′-(5′-(4-(1H-imidazol-1-yl) phenyl)-[1,1′:3′,1′′-terphenyl]-4,4′′-diyl) bis(1H-imidazole); G = guest molecules), abbreviated as iMOF-14C (iMOF = ionic MOF; C = cationic). Composed of Cd(II), perchlorate and an imidazole ligand L, iMOF-14C exhibits selective photoluminescence (PL) quenching towards detecting a specific spectrum of antibiotics and pesticides from the contaminated water. It is also responsive to some nitro-functionalised toxins, viz. nitrofuran antibiotics, such as nitrofurazone (NFZ) and nitrofurantoin (NFT), as well as pesticides, including nitrofen (an herbicide) and chloropyriphos (CHPS). iMOF-14C presents high selectivity towards these target analytes even in the presence of other interfering antibiotics and pesticides, and it reveals a turn-off PL response towards trace levels of NFT and NFZ, with detection limits as low as 100 ppb and 20 ppb, respectively. Furthermore, it detects CHPS and nitrofen at 50 ppb and 250 ppb, respectively, i.e., among all porous solids, iMOF-14C stands out with record-high pesticide sensing performance. Upon hybridising iMOF-14C with low-cost polymer polyvinylidene fluoride (PVDF), the composite membrane iMOF-14C@PVDF overcomes processability issues oft-encountered with MOF powders while also exhibiting efficient sensing of antibiotics in water.
Design, System, Application
Limited access to clean drinking water is recognised by the United Nations (SDG6) as one of the global grand challenges. Escalating water pollution is arguably the prime culprit. With spiraling industrialization and urbanization, clean water scarcity is only bound to exacerbate. Several emerging organic microcontaminants, such as, antibiotics and pesticides, are increasingly contributing to this alarming situation, primarily driven by their bioaccumulation caused detriments. Therefore, systematic identification of these highly toxic antibiotics and pesticides is of marked relevance. To break the trade-offs between low sensitivity, poor selectivity, high cost, and recyclability driven by high stability, new bottom-up material design blueprints are primed to be the gamechangers. To this end, luminescent metal–organic frameworks (MOFs), an advanced class of crystalline porous solids emerged as the next generation sensory materials and gained tremendous attention owing to their unique structural advantages such as ease in tunability. In this work, we report the design, synthesis and strategic utilization of a new stable luminescent cationic MOF fabricated with an electron-rich organic backbone. The combined merit of the electron-rich framework backbone and water stability of the MOF was further harnessed towards selectively recognising electron-deficient antibiotics and pesticides in water, in powder form, as well as a MOF-polymer composite.
|
Introduction
One of the pressing global challenges of current times is water pollution, specifically, the lack of clean water and sanitation.1,2 According to the United Nations Sustainable Development Goal 6 (SDG6), this is recognised as a basic necessity for all forms of life. A conspicuous increase in global population along with surging urbanization and energy demand further compounds this problem.3,4 With the rise in awareness regarding community health and natural water quality, demand for the detection and sequestration of toxic pollutants from sewage water is also gaining importance.5,6 In this regard, emerging organic contaminants and other anthropogenic contaminants account for a significant share of the pressing problem.7 In essence, a water contaminant is defined under the Safe Drinking Water Act (SDWA) as any foreign chemical, physical, biological or radiological object present in the water.8 Among the anthropogenic wastes, antibiotics and pesticides are two of the most significant pollutant classes contributing to water pollution at the global scale.9,10 Antibiotics are a class of drugs with widespread use in treating bacterial infections, animal husbandry, agriculture, and pharmaceutical industries.11–13 Due to their ability to suppress the growth of many diseases, they are frequently used as medicine in hospitals for both human and veterinary treatments.14,15 In addition to this, the need for sharp growth in agricultural production also necessitates the usage of antibiotics in the fields for food production, beekeeping, aquaculture, etc.16,17 However, continuous misuse and abuse of antibiotics can result in excessive levels of antibiotic residues in water bodies, implying a high risk to human health. Antibiotics, as a result, are now considered one of the major anthropogenic pollutants.18,19 On the other hand, pesticides, a category of emerging organic pollutants, have recently gained the “matter of concern” status.20,21 Modern agriculture heavily depends on the extensive usage of pesticides to elevate production. Pesticides are hazardous to both agricultural pests and to water bodies in which they are drained, culminating in water contamination.22–24 Prolonged exposure to such hazardous contaminants can adversely affect the nervous system of living organisms, for example, worsening the reproductive and respiratory systems of humans.25–27 Hence, systematic and routine monitoring of these antibiotics and pesticides in water has evolved as a crucial research issue, enabling us to mitigate the risks associated. To address these issues, effort towards the development and optimization of various analytical techniques have been undertaken in the last few decades. Some of them are Raman spectroscopy (RS), mass spectroscopy (MS), LC with UV detection (LC-UV), liquid chromatography with tandem mass spectrometry (LC-MS), and ion mobility spectroscopy (IMS). All these strategies are typically applied toward sensing organic contaminants.28–31 However, most of them are handicapped by limitations, such as slow kinetics, low efficiency, high cost, and complex quantification methods, which impede their applicability in more advanced applications. Hence, the development of low-cost, simple, highly sensitive and fast techniques that enable the selective detection of hazardous emerging contaminants has become a research hotspot.32,33 In this context, fluorometric detection techniques have gained a lot of traction owing to their fast kinetics, ease of handling, low cost, and high sensitivity and selectivity. Moreover, functional porous adsorbents are likely to offer higher selectivity and sensitivity towards a specific analyte as the pores allow analyte preconcentration.34–36
Metal–organic frameworks (MOFs), a relatively new family of crystalline porous materials with potential voids, are self-assembled by the periodic combination of metal ions/clusters and organic linkers.37 Since their discovery, MOFs have found a variety of potential uses.38–40 Besides, thanks to their compositional modularity, the luminescence signatures in MOFs can be controlled in a bottom-up manner. Simply put, this is achievable due to the combined contribution of photoluminescence (PL) characteristics of their π-rich (or deficient) organic ligand bridges, functional metal nodes, and adsorbed guest molecules.34 In this regard, luminescent MOFs (LMOFs) have exhibited their potential in various applications, particularly those addressing environmental concerns.41–43 A wide spectrum of LMOFs have demonstrated benchmark sensory properties towards detecting small molecules, hazardous gases, heavy metal ions, and toxic organic molecules, often presenting new paradigms of chemosensing.44–47 Nonetheless, studies on the utilization of ionic LMOFs for selectively detecting organic pollutants are too few and far between.48–52 The possible explanation for this could be the lack of stable cationic LMOFs, which are still a rarity (<10, source: Web of Science).48 To overcome this problem, we harnessed a π-electron-rich organic ligand with a highly conjugated aromatic skeleton. As a result, the MOF was highly emissive with major PL contributions from ligand-centred emission. Following the principle of HSAB (hard and soft acids and bases), the neutral and tripodal tris(imidazolate) linker was utilised.53,54 These factors facilitated metal–ligand coordination to afford a cationic framework featuring high chemical stability, which primarily arises from the complete shielding of the metal centres by the linker scaffolds. Effectively, to form a stable cationic LMOF, we selected the d10 transition metal Cd(II) to coordinate with the highly conjugated tris(imidazolyl) ligand, L, (1,1′-(5′-(4-(1H-imidazol-1-yl) phenyl)-[1,1′:3′,1′′-terphenyl]-4,4′′-diyl) bis(1H-imidazole). A novel, highly luminescent and hydrolytically stable cationic MOF, namely iMOF-14C, was the outcome and was studied herein. To explore its potential as a sensory probe, we strategically combined its a) electron-rich framework and b) water stability towards achieving the selective recognition of electron-deficient antibiotics and pesticides in water. iMOF-14C presented a highly sensitive and selective turn-off PL detection response towards a particular type of antibiotics and pesticides with ultrafast response times (Scheme 1). The limit of detection (LOD) values were found to be among the lowest reported for MOF-based sensors thus far.
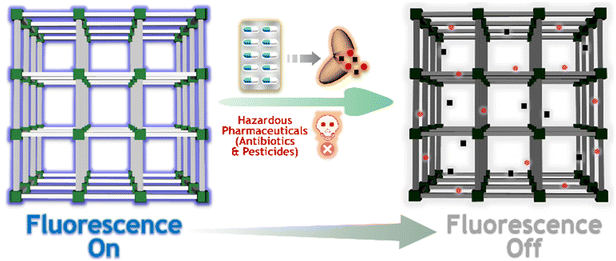 |
| Scheme 1 The schematic representation of the turn-off fluorescence response of iMOF-14C towards hazardous antibiotics and pesticides. | |
Experimental details
Caution
Benzene is a highly toxic and volatile solvent that can exert carcinogenic effects on human beings. Hence, appropriate safety measures were taken prior to its use, and it was handled with caution. Cadmium is included in the list of candidate substances of very high concern, according to EU regulation no. 1907/2006 (Registration, Evaluation, Authorisation and restriction of Chemicals, REACH list) and was handled with caution.
Materials
All the required organic reagents (to prepare the ligand L), Cd(ClO4)2·xH2O and solvents were procured commercially and used without any further purification.
The synthesis of the linker
The organic ligand 1,1′-(5′-(4-(1H-imidazol-1-yl)phenyl)-[1,1′:3′,1′′-terphenyl]-4,4′′-diyl) bis(1H-imidazole) (L) was synthesised according to a reported protocol (Scheme S1†).55
The synthesis of iMOF-14C
A slow-diffusion crystallization technique was employed to synthesise iMOF-14C (Scheme S2†). The ligand L (10.15 mg, 0.02 mmol) was dissolved in N,N′-dimethylformamide (DMF) and introduced in a cylinder-shaped glass tube to form the bottom layer. The second layer on top of the linker solution contained 1 mL benzene, which acted as a barrier, slowing down the diffusion of the metal and the ligand solutions. On the very top, Cd(ClO4)2·xH2O (6.22 mg, 0.02 mmol) was introduced in a 1 mL methanolic solution. This three-layered diffusion system was maintained in isolation and was not disturbed. Colourless hexagonal crystals were acquired within 14 days. The single crystals were acquired via filtration and washed several times with methanol. Pure iMOF-14C single crystals were obtained (yield ∼60%, based on Cd). The iMOF-14C crystals were further solvent-exchanged with MeOH for 48 hours, and all the free solvent molecules were degassed by evacuating the dried crystalline solid at 60°C. The formula of iMOF-14C was calculated by employing the PLATON SQUEEZE function to the crystal structure and found to be [Cd(L)2(ClO4)2·xG]n (G = disordered guest molecules).
The water stability test
To check the water stability, 50 mg of desolvated iMOF-14C was immersed in 20 mL of deionized (DI) water for 24 h at room temperature. Subsequently, the crystalline compound was accumulated via filtration and allowed to dry for further characterisation. In addition, inductively coupled plasma atomic emission spectroscopy (ICP-AES) was performed with the supernatant solution to check for leakage of Cd in the water.
PL measurements
Fine, activated iMOF-14C powder was employed for the fluorometric sensing studies. 1 mg iMOF-14C powder was dispersed in 2 mL of water, and subsequently, 1 mM stock solutions of various antibiotics (nitrofurazone (NFZ), nitrofurantoin (NFT)) and pesticides like nitrofen (an herbicide) and chloropyriphos (CHPS) (20–300 μL) in water were individually introduced. The gradual decrease in PL response was observed under constant stirring.
Results and discussion
Structural description of iMOF-4C
As stated in the experimental section, iMOF-14C single crystals were acquired via slow diffusion of the solutions of ligand (L) and Cd(ClO4)2·xH2O in a ternary solvent combination of DMF, benzene and MeOH at room temperature (Scheme S2†). The single-crystal X-ray diffraction experiments revealed that the two-dimensional (2D) network structure of iMOF-14C crystallized in the P1 space group (Table S3†). The asymmetric unit of iMOF-14C was found to comprise one Cd(II) with 1/2 occupancy, which was further connected to two full ligand units. One ClO4− anion remained free, occluding the pores, alongside one DMF molecule and other solvent molecules (Fig. 1a, S1 and S2†). Harnessing the five-membered imidazolates as the ligating termini was found to be advantageous to reduced steric crowding around the central Cd(II), which allowed the simultaneous coordination with six L units, resulting in the Cd(II)-L octahedral geometry (Fig. 1b, S3†). The average Cd–N bond distance was found to be 2.35 Å. Due to the hydrophobic ligand design of L, iMOF-14C exhibited a bi-porous nature, with one small and one large pore contributing to the construction of the framework (Fig. 1c, S4–S8). This characteristic is known to facilitate preferential interactions with incoming guest molecules that exhibit differences in electronic polarisation.28iMOF-14C was found to have a binodal kgd topology with (3,6) connections between the 2D metal–organic sheets, culminating in the intrinsic porosity of the compound. The free ClO4− anions further contributed to counterbalance the residual positive charge in the framework. However, we could not completely locate the free ClO4− anions crystallographically because of crystallographic disorder, but successfully confirmed their presence by Fourier-transform infrared spectroscopy (FTIR), Energy Dispersive X-Ray analysis (EDX) and elemental mapping studies. The PLATON analysis of the iMOF-14C structure revealed a guest-accessible volume (excluding anions) of 4591 Å3, accounting for ca. 58% of the porous nanospace. The structural integrity and bulk phase purity of iMOF-14C were confirmed by powder X-ray diffraction (PXRD) analysis, albeit the little variations found in the experimental PXRD pattern. This is attributed to the loss of solvent molecules, a common occurrence that results in the slippage of the 2D layers in layered network structures.55 Such subnetwork displacement often tends to affect phase purity to varying extents (Fig. S9†).55 The thermogravimetric analysis of the as-synthesised iMOF-14C revealed an initial ∼4% weight loss corresponding to the trapped solvent molecules of DMF and water (Fig. S10†). iMOF-14C was found to be stable until ∼350 °C, which was followed by a significant loss of the organic ligand (ca. 400 °C), leading to framework degradation. The FTIR spectra of iMOF-14C confirmed the coexistence of all the ligand characteristic peaks and an additional peak at around ∼1100 cm−1, indicative of the free ClO4− anions (Fig. S11†). The field-emission scanning electron microscopy (FESEM) images of iMOF-14C confirmed the hexagonal morphology of the iMOF-14C single crystals, and the EDX spectra revealed the presence of all elements at the expected ratio (Fig. S12–S14†). In addition, the PXRD patterns corroborated well with the water stability of iMOF-14C, which can directly be correlated to the hydrophobic shielding offered by Cd(II) flanked by the six independent imidazolates (Fig. S15†). Cadmium concentration was monitored by ICP-AES, revealing the presence of a negligible amount of Cd (0.16 ppm) in the supernatant. This observation also directly supports the hydrolytic stability of iMOF-14C.
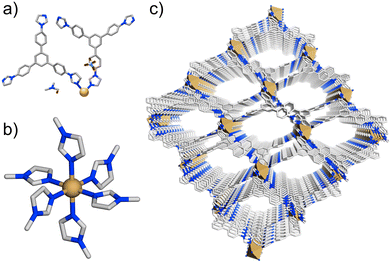 |
| Fig. 1 The structural representation of iMOF-14C illustrating (a) the asymmetric unit and (b) the coordination environment of Cd(II); (c) the view along the c axis, showing one-dimensional ultramicroporous (<7 Å) channels that sustain the MOF (colour code: grey − C, blue − N, dark yellow − Cd, red – O, green – Cl; ClO4−, the solvent molecules and hydrogen atoms are omitted for clarity in figures b and c). | |
Photoluminescence studies
The luminescence quantum yield of iMOF-14C was found to be 11.01% (absolute error ± 0.06; relative error ± 5.432 × 10−4). Because of its high luminescent nature, we envisioned that iMOF-14C can induce selective interactions with guest antibiotic and pesticide molecules, which may lead to variations in PL signatures between iMOF-14C and its guest-occluded phases. For iMOF-14C, the room-temperature PL spectra were recorded in both the aqueous phase and solid state. The observations alluded to a broad intense single emission spectrum with a peak at 375 nm upon excitation at 330 nm, which can be attributed to either the ligand-centred photoluminescence properties or the emission fingerprint arising from ligand-to-ligand charge transfer (LLCT) (Fig. S16†).20,21
Antibiotic sensing studies
The confluence of the electron-rich backbone, strong luminescence properties and hydrolytic stability of iMOF-14C prompted us to explore its potential as a chemosensor in water. To examine its sensory response towards organic micropollutants of different kinds, we conducted photoluminescence spectroscopy experiments with aqueous solutions of several such antibiotics and pesticides (Fig. S17 and S24†). As for antibiotics, we selected nine commonly used antibiotics with different chemical compositions. These included nitrofurantoin (NFT) and nitrofurazone (NFZ) from the class of nitrofurans, sulfaguanidine (SGD) from the class of sulphonamides, ampicillin (AMP) from the class of penicillins, sulfathiazole (STH) from the class of organosulfurs, sulfapyridine (SPD) from the class of sulfonamides, spectinomycin (SPCT), tetracycline (TTC) and kanamycin (KCS). 1 mg of the iMOF-14C powder was dispersed in 2 mL water, and the PL emission spectra were recorded subsequently. Thereafter, we introduced a 300 μL aqueous solution (1 mmol) of the selected antibiotics into the mixture individually, and changes in the photophysical properties of iMOF-14C were monitored. iMOF-14C exhibited 1) rapid PL quenching even under UV–vis light (Fig. 2a and b) and 2) rapid PL quenching towards NFT and NFZ with incremental addition of the antibiotics in the water medium (Fig. 3a and d and S18 and S19†). On the contrary, it showed insignificant PL quenching towards other selected antibiotics. This observation amply demonstrates the selective fluorescence turn-off response of iMOF-14C towards the nitrofuran antibiotics, such as NFZ and NFT, which are electron-deficient in nature. Further, the experimental fluorescence data for NFZ and NFT were investigated using the Stern–Volmer (SV) equation, (I0/I = 1 + KSV·[Q]), in order to quantify the quenching process, where I0 and I stand for the luminescence emission intensity of iMOF-14C before and after the addition of aqueous the antibiotics solution, [Q] stands for the concentration of the corresponding antibiotics, and KSV represents the Stern–Volmer Constant (Fig. 3b and e). iMOF-14C exhibited a very high KSV value of 4.5 × 104 M−1 towards NFT and 9.9 × 104 M−1 towards NFZ, which stand amongst the best performances reported in the field of porous sensory materials. Furthermore, the LOD values of iMOF-14C were estimated to be as low as 100 ppb for NFT and 20 ppb for NFZ (Fig. S20 and S21†). Superior selectivity is another major aspect considered for a sensory material to be upgraded to real-time applicability. To address this aspect, we examined the PL response of iMOF-14C towards other selected antibiotics (SGD, AMP, STH, SPD, SPCT, TTC, and KCS) at the same concentration as the nitrofuran antibiotics in water (Fig. S22 and S23†). Interestingly, slight quenching of PL was evident in the case of the other antibiotics. Enthused by these findings, we proceeded further to examine the efficacy of iMOF-14C towards the selective recognition of NFT and NFZ in water while the other antibiotics are present (Fig. 3c and f). To execute this experiment, 1 mg of iMOF-14C was dispersed in water, and a stock solution of a particular antibiotic was added to it subsequently. After an equilibration time span of 2 minutes, the emission intensities were collected, and further, an aqueous solution of nitrofuran antibiotics was introduced to the solution individually, and the PL spectra were recorded again. Interestingly, we found that the competing antibiotics did not exhibit any substantial alteration in luminescence intensity, but introducing nitrofuran antibiotics resulted in a significant PL quenching of iMOF-14C (Fig. 3c and f). The electron-rich nature of the tripodal organic ligand results in the highly electron-rich pore iMOF-14C surface, which predominantly interacts with the electron-deficient guest molecules. Considering all the observations, iMOF-14C functions as a selective sensor towards nitrofuran antibiotics, and it is arguably a general trend it exhibits towards electron-deficient micropollutants over their electron-rich analogues.
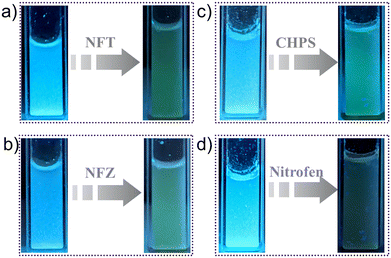 |
| Fig. 2 Photographs of the emission intensity changes of iMOF-14C in water under UV–vis light (λ = 350 nm) upon the addition of an (a) aqueous solution of NFT, (b) aqueous solution of NFZ, (c) aqueous solution of CHPS and (d) aqueous solution of nitrofen, each showing rapid quenching of PL emission intensity. | |
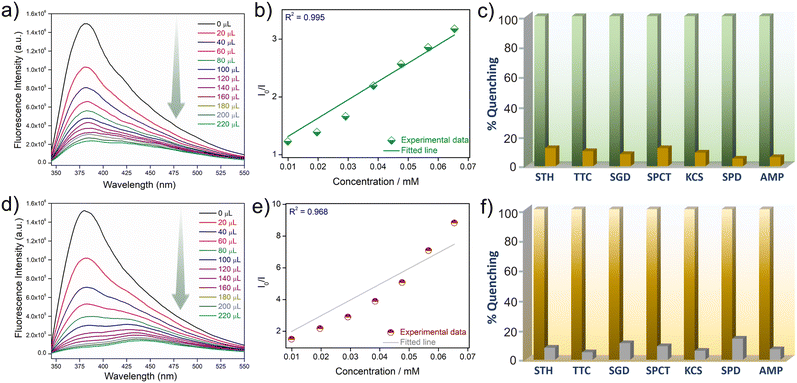 |
| Fig. 3 (a) The fluorescence turn-off response of iMOF-14C upon incremental addition of NFT; (b) the Stern–Volmer plot for iMOF-14C PL quenched by NFT; (c) the %PL quenching of iMOF-14C by the competing analytes alone (brown) and in the presence of their 1 : 1 binary mixtures with NFT (green); (d) the fluorescence turn-off response of iMOF-14C upon incremental addition of NFZ; (e) the Stern–Volmer plot for iMOF-14C PL quenched by NFZ; (f) the %PL quenching of iMOF-14C by the competing analytes alone (brown) and in the presence of their 1 : 1 binary mixtures with NFZ (green). | |
Pesticide sensing studies
As discussed earlier, apart from toxic antibiotics, aquatic contamination by hazardous pesticides has raised significant ecological concerns. This led us to explore the potential of iMOF-14C in recognising hazardous pesticides with good selectivity. For these studies, we selected four different pesticides, namely (2,4-dichloro-1(4-nitrophenoxy)-benzene (nitrofen), chloropyriphos (CHPS), cypermethrin and fenazaquin (Fig. S24†). Methods similar to antibiotic sensing were followed for the pesticide recognition experiments. iMOF-14C showed rapid quenching of fluorescence intensity under the UV–vis light setup, and a luminescence turn-off signal with the gradual addition of CHPS and nitrofen (Fig. 2c and d and 4a and d). Further, during the titration experiments, upon the addition of 300 μL (1 mmol) of the aqueous solutions of the four aforementioned pesticides, iMOF-14C demonstrated a selective PL quenching behaviour towards CHPS and nitrofen, while the changes in emission response towards the other two pesticides were relatively small (Fig. S25 and S26†). Further, we determined the KSV values towards both the pesticides to a) quantify the PL quenching phenomenon and b) establish the relationship between analyte concentration and PL quenching (Fig. 4b and d). iMOF-14C registered a very high KSV value of 1.16 × 105 M−1 for CHPS and 2.01 × 105 M−1 for nitrofen. These values are believed to be among the highest reported among porous materials thus far. In addition, the LOD values for nitrofen and CHPS were estimated by comparing the PL quenching of iMOF-14C with the analyte concentration (Fig. 4c and f). The LOD values were found to be as low as 250 ppb and 50 ppb for CHPS and nitrofen, respectively. Such a sensitive detection performance of iMOF-14C highlights its potential in the efficient detection of microcontaminants.
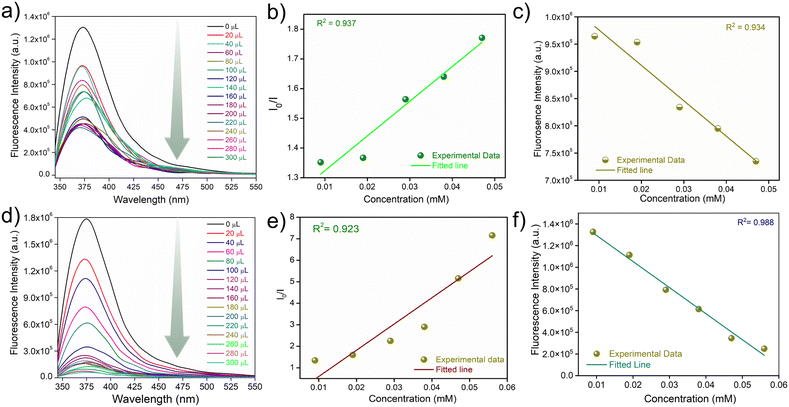 |
| Fig. 4 (a) The fluorescence turn-off response of iMOF-14C upon incremental addition of CHPS; (b) the Stern–Volmer plot for iMOF-14C PL quenched by CHPS; (c) the LOD determination plots for the PL quenching responses of iMOF-14C towards CHPS; (d) the fluorescence turn-off response of iMOF-14C upon incremental addition of nitrofen; (e) the Stern–Volmer plot for iMOF-14C quenched by nitrofen; (f) the LOD determination plots correlating with the PL-quenching responses of iMOF-14C towards nitrofen. | |
To analyse the quenching trend, we compared the emission spectra of iMOF-14C with the absorption spectra of the antibiotics and pesticides (Fig. S27 and S28†). In this study, we found significant overlaps between the emission spectra of iMOF-14C and the absorption spectra of NFZ and NFT (antibiotics), as well as CHPS and nitrofen (pesticides). These findings are clearly indicative of the energy transfer between the MOF framework and the corresponding analytes, which eventually results in the selective photoluminescence quenching of iMOF-14C. Such a significant overlap between the absorption spectra of the targeted antibiotics/pesticides and the emission profile of iMOF-14C also highlights the likely contribution of the inner filter effect to PL quenching.
Furthermore, the post-sensing phases of iMOF-14C were characterised by confocal fluorescence imaging and FESEM. The FESEM images of the post-recognition stage confirmed that the iMOF-14C crystallites retained the morphology (Fig. S29†). We probed the molecule-specific recognition by iMOF-14C further via confocal fluorescence imaging studies. For this experiment, the effects of NFT and NFZ on the iMOF-14C single crystals were monitored under a confocal microscope. The iMOF-14C single crystals showed very good luminescence turn-off behaviour upon incremental addition of NFT and NFZ (Fig. S30–S32†). Simply put, this study offers direct visual evidence of the PL quenching behaviour of the single crystals of iMOF-14C.
MOF@polymer composite studies
To overcome the practical challenges associated with the processing of powdered MOFs, our current findings prompted us to develop a standalone iMOF-14C@PVDF composite membrane, a prototypical MMM, as a sensory probe for organic micropollutants. Combining iMOF-14C with the PVDF polymer (see the ESI† for details) led to the preparation of the iMOF-14C@PVDF MMM (Fig. 5a, S33†).56 The self-standing iMOF-14C@PVDF MMM showed strong PL emission behaviour under UV light (λ = 350 nm) (Fig. 5b, S33†). Further, to check its antibiotic- and pesticide-sensing performances, we monitored its PL response towards a model antibiotic (NFT) and a pesticide (CHPS). Interestingly, the intense photoluminescence of iMOF-14C@PVDF was found to decline rapidly upon the addition of the corresponding antibiotic and pesticide solutions under UV light (λ = 350 nm) (Fig. 5b). Further, the solid-state fluorescence emission spectra of iMOF-14C@PVDF were also found to be consistent with the rapid recognition of NFT and CHPS. These observations highlight the yet untapped potential of MMMs, such as iMOF-14C@PVDF, in the real-time aqueous-phase sensing of organic micro-contaminants with enhanced selectivity.
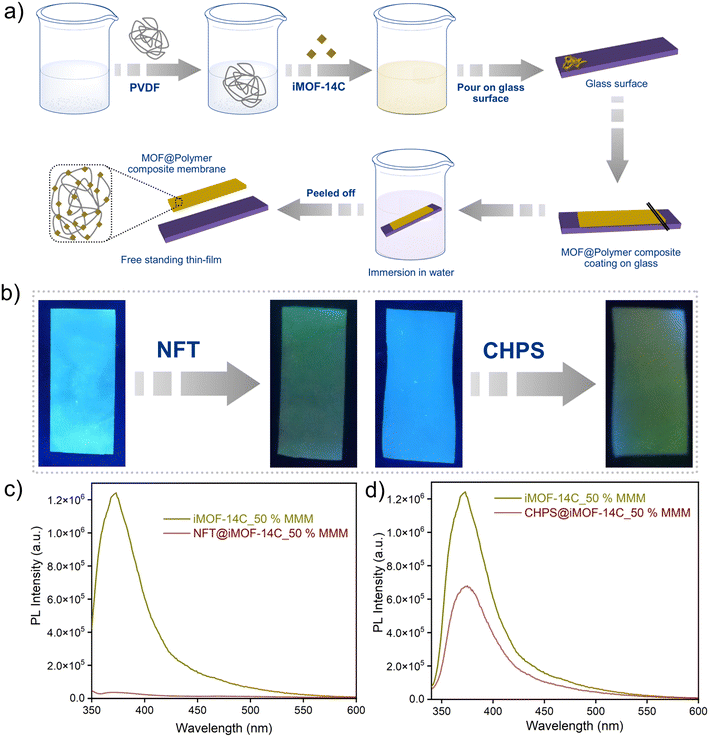 |
| Fig. 5 a) The schematic illustration of the fabrication of the iMOF-14C@PVDF film; PVDF stands for poly (vinylidene fluoride); b) the digital images of self-standing iMOF-14C@PVDF MMMs under UV light, and their change in emission properties upon the addition of NFT antibiotic and CHPS pesticide; changes in the fluorescence emission profile of iMOF-14C@PVDF upon the addition of c) NFT antibiotic and d) CHPS pesticide. | |
Conclusion
In summary, a new cationic 2D MOF, namely iMOF-14C, was found to demonstrate a rapid and selective fluorescence turn-off recognition response in the water towards hazardous microcontaminants, particularly nitro-functionalised antibiotics and pesticides. Further, iMOF-14C possessed excellent selectivity towards the nitrofuran antibiotics NFT and NFZ even in the presence of several different types of antibiotics. The quenching constants for NFT and NFZ detection set new benchmarks among porous-solid-based sensors, whereas the corresponding LOD values allude to the real-time application potential of the as-prepared sensing material. In addition, iMOF-14C also exhibited very selective and sensitive recognition of specific pesticides, namely CHPS and nitrofen. Overall, iMOF-14C was found to selectively detect electron-deficient micropollutants over their electron-rich congeners. This feature was the basis for its translation into iMOF-14C@PVDF, a prototypical MOF@polymer composite membrane. In the context of the pressing environmental issue of water pollution by emerging microcontaminants, we believe this work paves a new route towards developing advanced sensory probes designed specifically from MOFs.
Conflicts of interest
There is no conflict to declare.
Acknowledgements
S. D., W. M. and A. V. D. thank IISER-Pune for research fellowship. S. F. acknowledges DST-Inspire fellowship (DST/INSPIRE/03/2016/001694). G. K. D. thank CSIR for research fellowship. S. M. acknowledges an SFI-IRC Pathway award (21/PATH-S/9454) from the Science Foundation Ireland. S. K. G. thanks SERB (Project No. CRG/2019/000906) for research funding.
References
- I. Ali, Chem. Rev., 2012, 112, 5073–5091 CrossRef CAS PubMed.
- S. Rojas and P. Horcajada, Chem. Rev., 2020, 120, 8378–8415 CrossRef CAS PubMed.
- S. B. Grant, J.-D. Saphores, D. L. Feldman, A. J. Hamilton, T. D. Fletcher, P. L. M. Cook, M. Stewardson, B. F. Sanders, L. A. Levin, R. F. Ambrose, A. Deletic, R. Brown, S. C. Jiang, D. Rosso, W. J. Cooper and I. Marusic, Science, 2012, 337, 681–686 CrossRef CAS PubMed.
- M. Patel, R. Kumar, K. Kishor, T. Mlsna, C. U. Pittman, Jr. and D. Mohan, Chem. Rev., 2019, 119, 3510–3673 CrossRef CAS PubMed.
- J. Tang, X. Ma, J. Yang, D.-D. Feng and X.-Q. Wang, Dalton Trans., 2020, 49, 14361–14372 RSC.
- S. Dutta, S. Let, S. Sharma, D. Mahato and S. K. Ghosh, Chem. Rec., 2021, 21, 1666–1680 CrossRef CAS PubMed.
- V. Seufert, N. Ramankutty and J. A. Foley, Nature, 2012, 485, 229–232 CrossRef CAS PubMed.
- I. Ali, M. Asim and T. A. Khan, J. Environ. Manage., 2012, 113, 170–183 CrossRef CAS PubMed.
- D. J. Lapworth, N. Baran, M. E. Stuart and R. S. Ward, Environ. Pollut., 2012, 163, 287–303 CrossRef CAS PubMed.
- W. Mandal, S. Fajal, P. Samanta, S. Dutta, M. M. Shirolkar, Y. D. More and S. K. Ghosh, ACS Appl. Polym. Mater., 2022, 4, 8633–8644 CrossRef CAS.
- M. Qiao, G. G. Ying, A. C. Singer and Y. G. Zhu, Environ. Int., 2018, 110, 160–172 CrossRef CAS PubMed.
- E. M. Dias and C. Petit, J. Mater. Chem. A, 2015, 3, 22484–22506 RSC.
- W. Yan, Y. Xiao, W. Yan, R. Ding, S. Wang and F. Zhao, Chem. Eng. J., 2019, 358, 1421–1437 CrossRef CAS.
- D.-W. Sun, L. Huang, H. Pu and J. Ma, Chem. Soc. Rev., 2021, 50, 1070–1110 RSC.
- W.-B. Zhong, R.-X. Li, J. Lv, T. He, M.-M. Xu, B. Wang, L.-H. Xie and J.-R. Li, Inorg. Chem. Front., 2020, 7, 1161–1171 RSC.
- X. Wang, N. Zhuo, C. Fu, Z. Tian, H. Li, J. Zhang, W. Wu, Z. Yang and W. Yang, Chem. Eng. J., 2017, 328, 816–824 CrossRef CAS.
- N. Xu, Q. Zhang, B. Hou, Q. Cheng and G. Zhang, Inorg. Chem., 2018, 57, 13330–13340 CrossRef CAS PubMed.
- G. Xian, L. Wang, X. Wan, H. Yan, J. Cheng, Y. Chen, J. Lu, Y. Li, D. Li, J. Dou and S. Wang, Inorg. Chem., 2022, 61, 7238–7250 CrossRef CAS PubMed.
- P. Samanta, S. Let, W. Mandal, S. Dutta and S. K. Ghosh, Inorg. Chem. Front., 2020, 7, 1801–1821 RSC.
- M.-L. Xu, Y. Gao, X. X. Han and B. Zhao, J. Agric. Food Chem., 2017, 65, 6719–6726 CrossRef CAS PubMed.
- K. He, Z. Li, L. Wang, Y. Fu, H. Quan, Y. Li, X. Wang, S. Gunasekaran and X. Xu, ACS Appl. Mater. Interfaces, 2019, 11, 26250–26260 CrossRef CAS PubMed.
- D. Tilman, K. G. Cassman, P. A. Matson, R. Naylor and S. Polasky, Nature, 2002, 418, 671–677 CrossRef CAS PubMed.
- S. T. Narenderan, S. N. Meyyanathan and B. Babu, Food Res. Int., 2020, 133, 109141 CrossRef CAS PubMed.
- S. Liu, L. Yuan, X. Yue, Z. Zheng and Z. Tang, Adv. Powder Technol., 2008, 19, 419–441 CrossRef CAS.
- A. Mojiri, J. L. Zhou, B. Robinson, A. Ohashi, N. Ozaki, T. Kindaichi, H. Farraji and M. Vakili, Chemosphere, 2020, 253, 126646 CrossRef CAS PubMed.
- C. L. Tao, B. Chen, X. G. Liu, L. J. Zhou, X. L. Zhu, J. Cao, Z. G. Gu, Z. Zhao, L. Shen and B. Z. Tang, Chem. Commun., 2017, 53, 9975–9978 RSC.
- X. Zhu, B. Li, J. Yang, Y. Li, W. Zhao, J. Shi and J. Gu, ACS Appl. Mater. Interfaces, 2015, 7, 223–231 CrossRef CAS PubMed.
- M. Tabrizchi and V. J. Ilbeigi, J. Hazard. Mater., 2010, 176, 692–696 CrossRef CAS PubMed.
- J. Moros, J. A. Lorenzo, P. Lucena, L. M. Tobaria and J. J. Laserna, Anal. Chem., 2010, 82, 1389 CrossRef CAS PubMed.
- K. Håkansson, R. V. Coorey, R. A. Zubarev, V. L. Talrose and P. Håkansson, J. Mass Spectrom., 2000, 35, 337 CrossRef.
- D. Moreno-González, F. J. Lara, N. Jurgovská, L. Gámiz-Gracia and A. M. García-Campnãa, Anal. Chim. Acta, 2015, 891, 321 CrossRef PubMed.
- D. Wu, A. C. Sedgwick, T. Gunnlaugsson, E. U. Akkaya, J. Yoon and T. D. James, Chem. Soc. Rev., 2017, 46, 7105–7123 RSC.
- A. Karmakar, P. Samanta, S. Dutta and S. K. Ghosh, Chem. – Asian J., 2019, 14, 4506–4519 CrossRef CAS PubMed.
- H. Wang, W. P. Lustig and J. Li, Chem. Soc. Rev., 2018, 47, 4729–4756 RSC.
- S. Mukherjee, S. Dutta, Y. D. More, S. Fajal and S. K. Ghosh, Dalton Trans., 2021, 50, 17832–17850 RSC.
- S. Mukherjee, D. Sensharma, O. T. Qazvini, S. Dutta, L. K. Macreadie, S. K. Ghosh and R. Babarao, Coord. Chem. Rev., 2021, 437, 213852 CrossRef CAS.
- H. Li, M. Eddaoudi, M. O'Keeffe and O. M. Yaghi, Nature, 1999, 402, 276–279 CrossRef CAS.
- S. Kitagawa, R. Kitaura and S.-I. Noro, Angew. Chem., Int. Ed., 2004, 43, 2334–2375 CrossRef CAS PubMed.
- S. Mukherjee and S. K. Ghosh, Nat. Rev. Chem., 2021, 5, 600–601 CrossRef CAS PubMed.
- S. Rojas, A. Rodríguez-Diéguez and P. Horcajada, ACS Appl. Mater. Interfaces, 2022, 15, 16983–17007 CrossRef PubMed.
- M. D. Allendorf, C. A. Bauer, R. K. Bhakta and R. J. T. Houk, Chem. Soc. Rev., 2009, 38, 1330–1352 RSC.
- W. P. Lustig, S. Mukherjee, N. D. Rudd, A. V. Desai, J. Li and S. K. Ghosh, Chem. Soc. Rev., 2017, 46, 3242–3285 RSC.
- L. E. Kreno, K. Leong, O. K. Farha, M. Allendorf, R. P. V. Duyne and J. T. Hupp, Chem. Rev., 2012, 112(2), 1105–1125 CrossRef CAS PubMed.
- Y. Wang, H. Zhao, X. Li and R. Wang, J. Mater. Chem. A, 2016, 4, 12554–12560 RSC.
- I. Tibbetts and G. E. Kostakis, Molecules, 2020, 25, 1291 CrossRef CAS PubMed.
- P. R. Lakshmi, P. Nanjan, S. Kannan and S. Shanmugaraju, Coord. Chem. Rev., 2021, 435, 213793 CrossRef.
- L. Heinke, M. Tu, S. Wannapaiboon, R. A. Fischer and C. Wöll, Microporous Mesoporous Mater., 2015, 216, 200–215 CrossRef CAS.
- S. Dutta, Y. D. More, S. Fajal, W. Mandal, G. K. Dam and S. K. Ghosh, Chem. Commun., 2022, 58, 13676–13698 RSC.
- H. He, L. Hashemi, M.-L. Hu and A. Morsali, Coord. Chem. Rev., 2018, 376, 319–347 CrossRef CAS.
- S. Dutta, S. Let, M. M. Shirolkar, A. V. Desai, P. Samanta, S. Fajal, Y. D. More and S. K. Ghosh, Dalton Trans., 2021, 50, 10133–10141 RSC.
- P. Kumar, A. Pournara, K.-H. Kim, V. Bansal, S. Rapti and M. J. Manos, Coord. Chem. Rev., 2017, 86, 25–74 CAS.
- S. Sharma, S. Let, A. V. Desai, S. Dutta, G. Karuppasamy, M. M. Shirolkar, R. Babarao and S. K. Ghosh, J. Mater. Chem. A, 2021, 9, 6499–6507 RSC.
- S. Sharma, S. Dutta, G. Dam and S. K. Ghosh, Chem. – Asian J., 2021, 16, 2569–2587 CrossRef CAS PubMed.
- S. Yuan, L. Feng, K. Wang, J. Pang, M. Bosch, C. Lollar, Y. Sun, J. Qin, X. Yang, P. Zhang, Q. Wang, L. Zou, Y. Zhang, L. Zhang, Y. Fang, J. Li and H.-C. Zhou, Adv. Mater., 2018, 30, 1704303 CrossRef PubMed.
- S. Dutta, P. Samanta, B. Joarder, S. Let, D. Mahato, R. Babarao and S. K. Ghosh, ACS Appl. Mater. Interfaces, 2020, 12, 41810–41818 CrossRef CAS PubMed.
- M. S. Denny Jr. and S. M. Cohen, Angew. Chem., Int. Ed., 2015, 54, 9029–9032 CrossRef PubMed.
Footnotes |
† Electronic supplementary information (ESI) available. CCDC 2225079. For ESI and crystallographic data in CIF or other electronic format see DOI: https://doi.org/10.1039/d3me00008g |
‡ These authors contributed equally to this work. |
|
This journal is © The Royal Society of Chemistry 2023 |