Hydrogen peroxide emissions from surface cleaning in a single-family residence†
Received
26th October 2022
, Accepted 16th March 2023
First published on 31st March 2023
Abstract
High levels of reactive chemicals may be emitted to the indoor air during household surface cleaning, leading to poorer air quality and potential health hazards. Hydrogen peroxide (H2O2)-based cleaners have gained popularity in recent years, especially in times of COVID-19. Still, little is known regarding the effects of H2O2 cleaning on indoor air composition. In this work we monitored time-resolved H2O2 concentrations during a cleaning campaign in an occupied single-family residence using a cavity ring-down spectroscopy (CRDS) H2O2 analyzer. During the cleaning experiments, we investigated how unconstrained (i.e., “real-life”) surface cleaning with a hydrogen peroxide solution influenced the indoor air quality of the house, and performed controlled experiments to investigate factors that could influence H2O2 levels including surface area and surface material, ventilation, and dwell time of the cleaning solution. Mean peak H2O2 concentrations observed following all surface cleaning events were 135 ppbv. The factors with the greatest effect on H2O2 levels were distance of the cleaned surface from the detector inlet, type of surface cleaned, and solution dwell time.
Environmental significance
Surface cleaning and disinfection can alter the composition of indoor air, sometimes leading to health risks. Emissions following the use of chlorine bleach have recently been characterized, but little is known about the effects of hydrogen peroxide (H2O2)-based cleaners on indoor air quality (IAQ). We measured H2O2(g) concentrations in a house during cleaning events using H2O2 solutions and observed elevated local H2O2(g) concentrations. We demonstrated that cleaning method (e.g., mopping vs. spraying) affects emissions, as do factors including the type of surface cleaned and the area of the surface cleaned. The results of this work can be used to inform evidence-based exposure mitigation strategies.
|
1. Introduction
Washing indoor surfaces has evident aesthetic and health benefits. However, it may also pose health risks.1–3 Several recent publications have reported changes in air composition following cleaning surfaces. Chlorinated species emitted and formed during the use of sodium hypochlorite bleach (“bleach”)4,5 and volatile organic compounds (VOCs) emitted during the use of plant-based (thymol and terpene) cleaners3,6 can cause irritation and harm to skin and to the respiratory system.7,8 Hydrogen peroxide (H2O2)-based cleaners are an alternative to bleach and have gained popularity in times of COVID-19 for being powerful oxidants and effective at deactivating SARS-CoV-2 on surfaces.9,10 Hydrogen peroxide has long- and short-term exposure limits of 1 and 2 ppmv, respectively,11 with potential exposure effects including irritation and inflammation of mucous membranes.12 Although H2O2 appears to be a safer alternative to bleach for routine surface cleaning, gas-phase concentrations of H2O2 during cleaning activities are largely unknown. In addition to direct exposure risks, H2O2 can undergo gas-phase reactions such as photolysis to form reactive hydroxyl radicals (OH). This chemistry is expected to be slow at ambient H2O2 concentrations (estimated at ∼1 ppbv) due to attenuation of short wavelength photons by windows.13,14 However, high H2O2 concentrations during and following cleaning events could increase photolysis rates sufficiently to affect indoor OH concentrations and alter indoor oxidative capacity.15
Only one study has measured H2O2 emissions during surface cleaning.14 That study, performed in an environmental chamber designed to mimic a room in a residence, provided important insight into the effects of using H2O2-based cleaners on indoor air composition. However, important questions, including the applicability of chamber results to occupied residences, remain. For example, most residences have larger air volumes than the chamber (29.1 m3); one might expect lower H2O2 levels due to dilution. Conversely, larger surface areas will often be cleaned in residences (a 0.75 m2 area was cleaned in the chamber), likely leading to higher emissions. Changes in ventilation (e.g., caused by opening windows) could also lead to large differences. Finally, recent studies have highlighted the spatial heterogeneity of indoor trace gases and aerosols (either directly emitted or formed via chemical reactions).16–19 Marked differences in H2O2 levels may be expected throughout the air volume of a residence. This work presents the first study on the effects of the factors listed above on H2O2 mixing ratios in a real, occupied residence during surface cleaning with an H2O2-based cleaner.
2. Methods
2.1 Instrumentation and measurements
From June 11 to July 24, 2020, continuous real-time measurements of gaseous H2O2 were acquired in an occupied two-storey single-family residence. The total area and interior volume (including basement) were 211 m2 and ∼500 m3, and the area and volume of the main storey (where experiments were primarily conducted) were 84 m2 and ∼200 m3. Hydrogen peroxide mixing ratios were quantified using cavity ring-down spectroscopy (CRDS; PI2114 Hydrogen Peroxide Analyzer; Picarro, Inc.). The limit of detection (LOD) was 0.6 ppbv for 15 s averages. The analyzer was placed on the dining room table throughout the field study, with the 1 cm inlet facing the rear of the house.
2.2 Cleaning activities
Surface cleaning was carried out using dilute H2O2 solutions. A 3% v/v aqueous H2O2 solution (Western brand) was diluted with tap water to 0.75%, 1%, or 1.5% v/v. These concentrations were chosen to represent typical ones found in H2O2 commercial cleaners. The cleaning solution was applied to surfaces in the house following three cleaning methods: (1) “washing”, which consisted of wiping a surface with a clean cloth soaked with the H2O2 solution then leaving the surface undisturbed; (2) “mopping”, which consisted of first spraying the solution over the floor using a spray mop and then distributing it across the floor with the mop; and (3) “spray wiping”, which consisted of first squirting the solution over the surface using a spray bottle and then wiping the surface dry with a clean paper towel or cloth. Surfaces across the first and second floors of the house such as tables, counters, floors, and windows were cleaned.
A total of 61 cleaning events took place. Most of the cleaning events (N = 43) were carried out under controlled conditions. The remaining cleaning events (N = 18) were unconstrained, with variables such as volume of cleaner deposited and surface area cleaned not controlled. In the controlled experiments, aqueous H2O2 solution was diluted to 1% v/v and applied to the surfaces following the “spray wiping” method. Cleaning solution was sprayed 35 times (corresponding to ∼15 mL) onto a 1.2–1.5 m2 section of a surface. For “regular cleaning” the surface was wiped dry with a clean paper towel or cloth after every 2–4 sprays; the cleaning event spanned ∼1–3 minutes. For “deep cleaning” the cleaning solution was left on the surface for 5 minutes following application before wiping it dry. Surfaces that were cleaned during controlled experiments were either stone or wood (both coated with an unknown sealant), with mean distances from the instrument inlet ranging from ∼86 cm to ∼560 cm (Table 1). Ventilation was increased by opening the patio door at the rear of the house. The timing of door opening events was not planned, as they were the result of a young occupant opening, and then failing to close, the door.
Table 1 Description of surfaces cleaned during controlled cleaning experiments
Surface |
Area (m2) |
Distance from inlet (cm) |
Surface material |
Dining room table (DRT) |
1.4 |
25–147 |
Wood |
Vertical front counter (VFC) |
1.4 |
∼172 |
Wood |
Near counter (NC) |
1.5 |
172–242 |
Stone |
Living room floor (LRF) |
1.5 |
147–272 |
Wood |
Kitchen floor (KF) |
1.5 |
242–432 |
Wood |
Far counter (FC) |
1.2 |
432–503 |
Stone |
Family room floor (FRF) |
1.5 |
510–604 |
Stone |
2.3 Data analysis
Peak H2O2 levels were defined as the highest value of the 15 s averaged H2O2 mixing ratios following surface cleaning. Total emitted H2O2 molecules is used as an indicator of exposure. It was calculated by integrating H2O2 mixing ratios over the time period from the onset of signal rise after surface cleaning to the time when H2O2 decreased to below the LOD (i.e., area under the curve) and converting that value to the number of molecules based on the instrument's flow rate of 760 ccm. Decay rate constants of H2O2 (k) were calculated for each surface cleaning event that had H2O2 peak concentrations significantly higher than the background values. Assuming constant background mixing ratios during the decay period, decay rate constants were determined using first-order exponential regression:where Ct is the H2O2 mixing ratio after time t, C0 is the initial (peak) mixing ratio, and Cb is the background mixing ratio during the decay event. First order kinetics were determined to provide the best fit to the decays, and no evidence of multi-exponential decays was observed. Fig. S1 in the ESI† shows a representative log-normal decay. Decay rate constant uncertainty was determined as the standard error of k obtained from the regression. We did not consider high frequency fluctuations in the signal (e.g., as shown in Fig. S2 in the ESI†) in our determination of t = 0.
Steady-state OH concentrations were predicted using a simplified steady-state approximation and concentrations of OH sources and sinks common in residences.18 A full description of calculations and considerations is provided in the ESI.†
3. Results and discussion
3.1 Direct emissions of H2O2 following unconstrained surface cleaning
Direct emissions of H2O2 were observed following surface cleaning. Cleaning surfaces in different rooms on the first storey of the house (e.g., floors, windows, counters, and furniture) consistently led to increases in H2O2 mixing ratios, with peak levels ranging from 12–840 ppbv (Fig. 1). Temporal profiles were similar to those reported for the use of H2O2 and other cleaning solutions on floors in laboratories, residences, and environmental chambers,4,14,20,21 with a rapid increase to a maximum followed by a slower decay to pre-cleaning levels. The mean peak H2O2 concentration observed in this study was statistically lower (at the 95% confidence level based on a paired Student's T-test) than those reported following floor cleaning using a commercial H2O2-based cleaning spray in an environmental chamber (134.8 ± 172.2 vs. 269.9 ± 98.0 ppbv).14 Lower levels in the house are expected compared to in the chamber due to the much larger volume of the house. Dilution due to mixing should be 17 times greater in the house (considering only the first storey) than in the chamber. The fact that mean peak levels were only 50% lower in the house suggests that although the environmental chamber presented a small, controlled environment compared to most indoor settings where surface cleaning occurs, the measurements made in that experiment are applicable to indoor settings and real-life applications of cleaning solution.
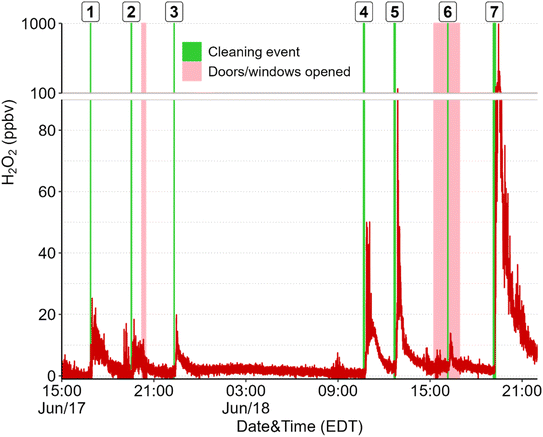 |
| Fig. 1 Temporal variations of H2O2 mixing ratios for select unconstrained cleaning events: event 1 – mopped dining room floor (1% v/v); event 2 – mopped kitchen floor (1% v/v); event 3 – mopped living room floor (1% v/v); event 4 – washed living room furniture (1.5% v/v); event 5 – washed family room door frames and window (1.5% v/v); event 6 – washed dining room table (1.5% v/v); event 7 – washed kitchen counters and furniture (1.5% v/v). | |
Temporal profiles of H2O2 following cleaning were also generally similar to those reported in an environmental chamber, with some variations that are well explained by distance from the inlet and incomplete mixing in the house. For example, for all cleaning that took place on the first floor of the residence (N = 57, including controlled experiments), the mean lag between application of cleaning solution and detection of elevated H2O2 levels was 6.1 (±1.6) min and peak levels were reached after (on average) an additional 4.8 (±3.2) min. Mixing ratios then decreased to pre-cleaning levels over the course of 36.6 (±20.5) minutes. Shorter lag and rise times were observed in the chamber (1.5 ± 0.9 min lag and 42 ± 6 s from rise to peak).14 Additionally, large fluctuations in the form of sharp peaks of ∼40 s duration were often observed during cleaning experiments in the house but not in the chamber (Fig. S2 in the ESI†). The larger range of peak levels observed in the house compared to in the chamber (which had mean peak levels of 76–480 ppbv for 30 s averages) is likely due to a combination of incomplete mixing, varying cleaning procedures, and varying ventilation (due to exterior doors and windows being open during some experiments). For example, the lowest peak H2O2 mixing ratio from cleaning events on the first storey (12.2 ppbv) was observed when windows and doors were opened, and no H2O2 was detected in the two experiments performed on the 2nd storey of the residence, suggesting that H2O2 was diluted or lost to surfaces before it reached the inlet on the first storey. The highest H2O2 levels were measured when surfaces near the inlet (kitchen and dining room floors, counters, and table) were cleaned over a period of 10 minutes (cleaning event 7 in Fig. 1). Although the peak mixing ratio of 840 ppbv was short-lived, it raises concern of exposure risk, as we do not currently know the effects of repeated short-term exposure to high H2O2 levels on human health. Further, people often clean multiple regions of a house or building consecutively, which could lead to repeated exposure of these high levels.
3.2 Factors influencing indoor H2O2 (controlled cleaning experiments)
We investigated the major factors that affect indoor H2O2 levels following cleaning by performing controlled cleaning experiments in which most variables were kept constant between experiments. Fig. 2 shows total H2O2 molecules following regular cleaning of 7 surfaces. While significant variations in total H2O2 molecules emitted were observed between different surfaces, the percent uncertainty for these cleaning events was much smaller compared to the unconstrained cleaning events (110% vs. 231% for peak H2O2 and 83% vs. 194% for total molecules). This suggests that variations in cleaning procedures (e.g., volume of cleaning solution applied, duration of cleaning, area of surface cleaned) are responsible for the large range of H2O2 levels observed during the unconstrained experiments. The following sections discuss individual variables that may be important to indoor H2O2 levels following cleaning.
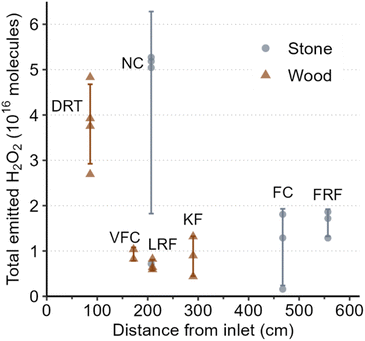 |
| Fig. 2 Total emitted H2O2 as a function of average distance from inlet for controlled regular cleaning events. Abbreviated texts indicate surface types listed in Table 1. Error bars represent the standard deviation about the mean. | |
3.2.1 Distance of surface from inlet.
Fig. 2 shows the distance dependence of total H2O2 emissions following cleaning of wood and stone surfaces under controlled conditions (mean peak H2O2 levels are shown in Fig. S3†). Mean peak mixing ratios (total emitted molecules), shown in Table 2, were 42.7 ± 20.4 ppbv ((10.5 ± 5.2) × 1015 molecules) for all surfaces except the wood and stone surfaces closest to the inlet (dining room table (DRT) and near counter (NC)). These closest surfaces yielded, on average, ∼7 (∼4) times more total molecules than the mean for all other surfaces. Similarly, the decay of H2O2 following cleaning was much faster for surfaces within ∼220 cm of the inlet (Table 2 and Fig. S4†), with mean decay rate constants ranging from 11–18 h−1, compared to 2.6–3.6 h−1 for surfaces more than ∼460 cm from the inlet. In the chamber study discussed previously,14 H2O2 decay rate constants ranged from ∼10–29 h−1, with faster decay measured when the surface area inside the chamber was increased. Surface deposition was hypothesized to be the major H2O2 loss term in the chamber. The rate constants measured for surfaces within ∼220 cm of the inlet in the current work are similar to those reported in the chamber study. This may suggest that surface deposition is also the dominant loss term for H2O2 in the house. However, it is also possible that dilution contributes significantly to H2O2 loss in the house. We recently investigated the spatial and temporal variability of gas-phase hypochlorous acid (HOCl) following bathroom surface disinfection with a commercial sodium hypochlorite bleach spray.19 In that work, HOCl was determined to be well-mixed at distances at least 160 cm from the surface, while air sampled within ∼60 cm of the surface was determined to be poorly mixed. This poor mixing was evidenced by a strong distance dependence for both peak HOCl concentrations and HOCl decay rate constants at short distances from the cleaned surfaces contrasted with a lack of distance dependence further from the surface. The distance dependences for H2O2 concentrations and for k in this study shows a similar pattern. The agreement between k measured near the inlet in this work and k in the chamber study may be coincidental.14 The lowest surface-area-to-volume ratio (S/V) in the chamber was 2.0 m−1. We did not quantify S/V in the house, but the larger rooms and higher ceilings suggest that S/V will be lower, despite the presence of furniture. It is likely that dilution, surface deposition, and air exchange all play a role in the decay rate constants measured in this study.
Table 2 Mean peak H2O2 mixing ratios, total emitted H2O2 molecules, and calculated decay rate constants (k) for regular cleaning experiments
Surface |
D
(cm) |
Peak H2O2 (ppbv) |
Total H2O2 (1016 molecules) |
k (h−1) |
N
|
Mean distance from inlet.
Number in parentheses denotes number of trials used to calculate k.
Stone surface.
|
Dining room table (DRT) |
86 |
254 ± 91 |
38.0 ± 8.8 |
12.7 ± 5.8 |
4 (4) |
Vertical front counter (VFC) |
172 |
70.9 ± 32.7 |
9.30 ± 1.50 |
17.8 |
2 (1) |
Near counter (NC)c |
207 |
323 ± 181 |
40.5 ± 22.3 |
11.8 ± 8.9 |
4 (6) |
Living room floor (LRF) |
209 |
31.0 ± 6.4 |
6.86 ± 1.21 |
11.6 |
3 (1) |
Kitchen floor (KF) |
290 |
31.0 ± 14.5 |
8.86 ± 4.40 |
— |
3 (0) |
Far counter (FC)c |
467 |
35.8 ± 19.7 |
10.8 ± 8.4 |
2.56 |
3 (1) |
Family room floor (FRF)c |
557 |
54.3 ± 6.2 |
16.2 ± 3.0 |
3.51 ± 1.43 |
3 (3) |
If dilution is the dominant loss term for measurements made near the inlet, we might expect to see bi-exponential decay in these experiments, with the first, faster exponential term describing loss via dilution, and second, slower exponential describing loss due to other processes such as surface deposition and air change. Fig. S1 in the ESI† shows a representative log-normalized decay profile for a near counter experiment. In all cases, the data was best fit by a single-exponential decay. A second exponential term may be present but not detectable due to sensitivity limitations. Specifically, we hypothesize that once H2O2 is well mixed within the house, its concentrations will be below the LOD of our method. This is supported by the fact that fewer than half of experiments performed at distances greater than 220 cm from the instrument inlet had sufficient signal to calculate a decay rate constant. Considering that 15 mL of 1% H2O2 solution contains ∼3.8 × 1021 molecules H2O2, we can estimate an upper limit for H2O2 levels in well-mixed air within the house. If 10% of deposited cleaning solution is released to the gas phase following cleaning, the concentration in well-mixed air within the first storey of the house would be ∼30 ppbv. This is a conservative upper limit, as we expect less than 10% of H2O2 to be emitted due to wiping excess solution off of the cleaned surfaces, mixing will not be limited to the first storey of the house, and loss via surface deposition and air change will occur. Regardless, given the frequent fluctuations in H2O2 concentration illustrated in Fig. S2 in the ESI,† nonlinearities in the log-normalized H2O2 decay profile from bi-exponential decay will be difficult to detect and quantify.
3.2.2 Type of surface cleaned.
In addition to observing a distance dependence for H2O2 mixing ratios, we also noted effects of the type of surface cleaned, with higher levels generally observed following cleaning of stone surfaces. For example, despite similar distances from the inlet (∼2.1 m) for NC (stone) and LRF (wood), peak (total) H2O2 levels were on average higher for NC by a factor of 10.4 (5.9) than for LRF (Fig. 2 and Table 2). Compared to the wood surface that was ∼0.35 m closer to the inlet (VFC), average peak (total) H2O2 levels for NC were also higher, by a factor of 4.6 (4.4). In fact, peak and total H2O2 levels following cleaning NC were as high as those for the wood DRT, which was less than half the distance from the instrument inlet. These observations indicate that in addition to distance, the type of surface also had an impact on H2O2 concentrations. As illustrated in Fig. 2, differences in measured H2O2 levels for wood and stone became much less pronounced at greater distances from the inlet, with mean peak (total emitted molecules) H2O2 levels for stone FC and FRF only 15–75% (22–136%) greater than those for wood KF and LRF.
The impact of types of surface cleaned on k appeared to be negligible, at least for surfaces within ∼2 m of the inlet (Fig. S3†). The decay rate constant of 11.8 h−1 measured for stone NC was in good agreement with the range of constants from 11.6–17.8 h−1 for the 3 wood surfaces (DRT, VFC, LRF) within 2.1 m of the inlet. Low peak H2O2 levels combined with large fluctuations in signal at greater distances resulted in very few calculated decay rate constants for stone FC and FRF, and no rate constants for wood KF, so we cannot currently comment on whether there are differences based on surface type. Overall, our observations suggest that cleaning stone surfaces in this house resulted in greater H2O2 emission than cleaning wood surfaces under identical conditions. This may be due to greater uptake of H2O2 by the wood surfaces. However, both types of surfaces in the house were sealed with unknown sealants (applied before the current owners took possession of the house), which can significantly alter the properties of materials. Therefore, we do not know whether the trend of greater emission from stone surfaces compared to wood surfaces is applicable to locations other than this house. Expanded investigations of H2O2 deposition rates to indoor surfaces, such as those reported by Poppendieck et al.,22 may provide further insight and enable generalizations and predictions.
3.2.3 Surface area cleaned.
Regular controlled cleaning involved spraying ∼15 mL of cleaning solution onto a 1.2–1.5 m2 area. We carried out several controlled cleaning experiments in which we sprayed the same volume of cleaning solution onto half of the regular surface area (0.75 m2). Fig. 3, Table S3 and Fig. S5† show the effects of reducing the surface area cleaned on H2O2 mixing ratios. We observed consistently lower H2O2 levels for peak levels (total molecules) when half areas were cleaned by an average of 20% (38%). These reductions may be due to the fact that excess cleaning solution was wiped off the surface immediately after application (following the regular cleaning procedure, as described in Section 2.2), leaving the surface visibly dry. The thickness of the film of cleaning solution left on the surface after wiping should not depend on the volume of liquid applied, so the total number of H2O2 molecules at the surface (either adsorbed to the surface or residing within the thin liquid film at the surface) will be proportional to the area of the surface cleaned. As expected, given that the area cleaned should not affect H2O2 loss kinetics, half area decay rate constants were statistically the same as those for the full area at the 95% confidence level.
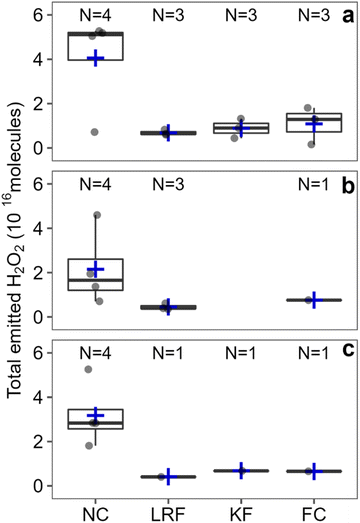 |
| Fig. 3 Statistics of total emitted H2O2 molecules for regular cleaning of four surfaces (near counter (NC), living room floor (LRF), kitchen floor (KF), and far counter (FC)) under different conditions: (a) full area cleaned with closed doors/windows, (b) half area cleaned with closed doors/windows, and (c) full area cleaned with patio doors open. The box and whisker plots show the median (line), mean (blue marker), first and third quartiles (box), and minima and maxima (whiskers). The number at the top of each box and whisker plot indicates the number of cleaning events for that condition. | |
3.2.4 Ventilation.
Hydrogen peroxide levels and decay kinetics discussed so far were all measured when doors and windows were closed. During the study period, the patio doors were occasionally opened by the house's occupants. These events were unscheduled and lasted an average of 44.7 ± 31.9 min. The air change rate (ACR) was not able to be quantified due to a lack of availability of resources during the study period. A rich body of literature has shown that ACR in residences generally varies within the range of 0.2–1 h−1,23,24 and that opening windows/doors can increase ACR by a factor of 1.3–3.9.25,26 Peak (total) H2O2 levels for NC in this study were on average 323.0 ± 180.6 ppbv ((40.5 ± 22.3) × 1015 molecules) when windows/doors were closed (Fig. 3, Table S4, and Fig. S5†). These values decreased by 33% (peak mixing ratios) and 22% (total molecules) respectively when the patio doors were open. Reductions in H2O2 levels were also observed for LRF, KF, and FC (by 24% (peak mixing ratios) and 35% (total molecules) on average), although only one open-door cleaning event was carried out for each of these three surfaces. These observations together suggest that opening doors increased ventilation and decreased H2O2 levels in the house after cleaning, likely due to more rapid physical removal of H2O2 and dilution of indoor air with outdoor air.27 Consistent with this interpretation, opening doors increased measured k by factors of 2.3 (NC) and 1.9 (FC) (Fig. 4 and Table S4†). We were unable to determine k for LRF with doors open due to low signals.
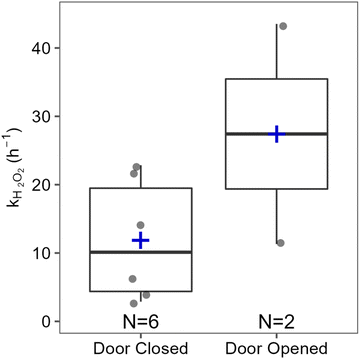 |
| Fig. 4 Statistics of kH2O2 for regular cleaning of the near counter under different ventilation conditions. The box and whisker plots show the median (line), mean (blue marker), first and third quartiles (box), and minima and maxima (whiskers). The number under each box and whisker plot indicates the number of cleaning events for that condition. | |
3.3 Deep cleaning
As illustrated in Fig. S6,† total emitted H2O2 molecules were ∼72% larger for deep cleaning of the near counter (N = 2) and the living room floor (N = 1) compared to regular cleaning of the same surfaces (N = 4 and 3, respectively). Peak levels were also higher for deep cleaning by ∼32% on average for both surfaces (Fig. S7†). The higher total emissions for deep cleaning are due to a combination of higher peak levels and longer residence times; H2O2 levels remained elevated for 65.5 ± 19.9 minutes following deep cleaning compared to 45.8 ± 23.8 minutes following regular cleaning.
These results are consistent with those in the environmental chamber,14 although H2O2 levels remained elevated for much longer and peak H2O2 levels were higher following deep cleaning in the chamber. This is likely due to different cleaning protocols. In the chamber study, the cleaning product manufacturer's protocol for deep cleaning was to spray the solution on the surface and to not wipe it off. In the house, the aqueous H2O2 solution was wiped off of surfaces after 5 minutes, which is a suitable dwell time for H2O2 disinfection.28 The cleaning solution used could also contribute to the difference, as a commercial H2O2-based cleaner, containing numerous species in addition to H2O2, was used in the chamber study.
3.4 Radical production from H2O2 photolysis
Oxidizing capacity indoors is generally expected to be very low. In environments with high ACR, indoor ozone (O3) levels may be as high as ∼85% of outdoor levels.29 However, O3 levels in residences are often very low (∼6 ppbv).30 Indoor OH concentrations are expected to be similar to those outdoors at night; concentrations of 104–105 molecule cm−3 have been calculated and measured indoors.31 The dominant OH formation mechanism is generally reactions between O3 and alkenes. As noted above, O3 levels tend to be very low in residences, leading to minimal OH production.32 In indoor air illuminated by sunlight, and in some cases artificial lights, OH radical concentrations can increase by one or more orders of magnitude. Nitrous acid (HONO) photolysis is the dominant indoor OH source in sunlit indoor air under most conditions.13,33–35 With indoor HONO concentrations averaging ∼4 ppbv, steady-state OH concentrations on the order of 106–107 molecule cm−3 have been predicted and measured.31,36,37
Several components of common cleaning agents are photolabile. For example, HOCl and Cl2 emitted from sodium hypochlorite bleach can form radicals when exposed to sunlight or room lights.18,38,39 Hydrogen peroxide can also photolyze to form OH.13–15 Ambient indoor H2O2 concentrations are low; ambient levels have not been reported in residential buildings, while ∼2 ppbv has been reported in a university.14 Photochemical OH production from H2O2 will be negligible under these conditions. During cleaning, H2O2 concentrations often increased to over 100 ppbv, reaching 840 ppbv in one experiment. We calculated OH formation rates from H2O2 photolysis using wavelength-resolved photon fluxes from Kowal et al.;13 the calculations are described in the ESI.† These rates, along with predicted steady-state OH concentrations ([OH]ss) at different H2O2 concentrations, are reported in Table 3. At the highest H2O2 concentration measured in this study (840 ppbv), we predict H2O2 photolysis to form OH at a rate of ∼6 × 106 molecule cm−3 s−1. This is ∼21% of the formation rate we predict from HONO photolysis under common indoor residential conditions. The predicted OH formation rate from H2O2 photolysis decreases to less than 5% of that predicted from HONO photolysis at the mean peak H2O2 concentration for all cleaning events in this study (135 ppbv), and [OH]ss concentrations are predicted to increase by a maximum of 21% for the highest H2O2 concentration measured in this study (840 ppbv). At a mean peak H2O2 level of 135 ppbv (averaged over the entire campaign), we predict [OH]ss to increase by only ∼3% following cleaning. We note that OH can be lost via reaction with H2O2, and that the rate of loss will increase with increasing H2O2 concentrations. However, as discussed in the ESI,† we believe that this loss term will be minor under common indoor residential conditions due to rapid regeneration of OH via HO2–NO reactions. These predictions suggest that HONO photolysis will be the dominant indoor OH source following the use of H2O2-based cleaners, but that H2O2 photolysis may increase [OH]ss slightly for short periods of time following cleaning. This is consistent with earlier work from our group which used an indoor chemistry box model to predict increases in [OH]ss of ∼10% when cleaning resulted in an H2O2 concentration of ∼313 ppbv.14 While the contribution of H2O2 photolysis to indoor [OH]ss will generally be short-lived, deep cleaning can result in sustained elevated H2O2 levels; under these conditions H2O2 photolysis may make a non-negligible contribution to indoor OH levels.
Table 3 Predicted OH production rates from H2O2 photolysis under unperturbed conditions and at peak H2O2 levels following cleaning, as well as predicted OH steady state concentrations
Condition |
[H2O2] (ppb) |
OH formation ratea (molecule cm−3 s−1) |
[OH]ssb (106 molecule cm−3) |
H2O2 contribution (%) |
The rate considers H2O2 photolysis as the only OH source.
Calculated as described by Zhou et al.18 The steady state concentration in the absence of H2O2 photolysis is predicted to be 2.6 × 106 molecule cm−3, with HONO photolysis as the only significant OH source. Details are provided in the ESI.
|
Background |
1 |
1.4 × 104 |
9.4 |
0 |
Peak (all experiments) |
135 |
9.1 × 105 |
9.7 |
3.4 |
Peak (near counter) |
323 |
2.2 × 106 |
10.4 |
8.1 |
Highest observed peak |
840 |
5.6 × 106 |
11.4 |
21.0 |
4. Implications
Hydrogen peroxide-based cleaners are commonly used to clean and disinfect indoor surfaces as an alternative to bleach, especially in times of COVID-19. In the present work, time-resolved measurements of H2O2 during and following household cleaning activities in an occupied single-family residence are reported for the first time. Elevated H2O2 levels following cleaning activities suggest that the conclusions drawn from H2O2 measurements in an environmental chamber during cleaning are applicable to real residences.14 Similar peak H2O2 levels were measured in the house and the chamber, despite the much larger air volume in the residence. This suggests that the relationship between total indoor volume and indoor H2O2 levels (both peak mixing ratios and total molecules) is not straightforward, and that relying on dilution to reduce H2O2 exposure risks may lead to a false sense of security. Our results also demonstrate the large variations in H2O2 levels that can be experienced at different times and locations within a connected indoor air volume (and even within a single room) following surface cleaning. In some cases, surfaces only a few meters apart resulted in total emissions at the detector inlet that varied by over a factor of 4. As discussed in the context of HOCl emitted from cleaning with NaOCl bleach,19 people who are closer to cleaned surfaces (such as those doing the cleaning or children and animals whose faces are generally closer to the floor than those of adults) may be exposed to much higher levels of emissions from cleaning products than others in the same room. On a more hopeful note, our results also indicate that exposure can be minimized by leaving the area immediately after cleaning a surface.
Large variability in H2O2 levels following unconstrained cleaning (in one case approaching the long-term exposure limit of 1 ppmv) along with measurements performed under controlled conditions demonstrate the importance of several factors on indoor H2O2 levels. Distance and surface type had the largest impact. Increasing the distance to the inlet from 207 to 557 cm reduced total emitted H2O2 molecules by 73%, and levels on the wood surface of the “vertical front counter” were 77% lower than the stone near counter, despite being the same distance from the inlet. Fig. 5 illustrates the effects of other factors investigated on total emitted H2O2. Decreasing the dwell time (wiping immediately vs. leaving the solution for 5 min) reduced the total number of molecules by ∼42%, and opening the patio door in the family room reduced it by ∼35%. Additional measures such as opening doors or windows in the same room as the cleaning activity, increasing ventilation through the use of fans or range hoods, or combining various measures may further reduce H2O2 levels, although this was not investigated in this study. This work increases our understanding of the effects of surface cleaning on indoor air quality, and may assist people in making healthy choices with respect to cleaning practices.
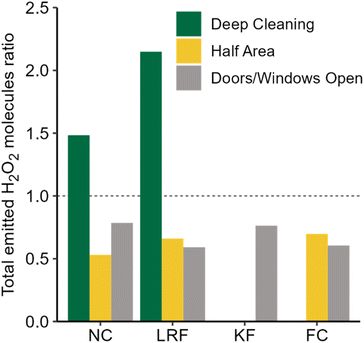 |
| Fig. 5 Ratio of total emitted H2O2 molecules under the cleaning conditions investigated (deep cleaning, half area, and door/windows open) to the standard controlled cleaning condition. | |
Conflicts of interest
There are no conflicts to declare.
Acknowledgements
Funding for this work was provided by the Alfred P. Sloan Foundation CIE program (G-2018-11062). Dr Kahan is a Canada Research Chair in Environmental Analytical Chemistry. This work was undertaken, in part, thanks to funding from the Canada Research Chairs program. The authors thank Dr D. Poppendieck and J. P. D. Abbatt for helpful discussions.
References
- J. P. D. Abbatt and C. Wang, The Atmospheric Chemistry of Indoor Environments, Environ. Sci.: Processes Impacts, 2020, 22(1), 25–48, 10.1039/C9EM00386J.
- C. J. Weschler and N. Carslaw, Indoor Chemistry, Environ. Sci. Technol., 2018, 52(5), 2419–2428, DOI:10.1021/acs.est.7b06387.
- G. Karr, M. Nicolas, F. Maupetit and M. Ramel, Cleaning Product Emissions and Indoor Built Environments: Exposure and Health Risk Assessments from Experiments under Realistic Indoor Conditions, Build. Environ., 2021, 206, 108384, DOI:10.1016/j.buildenv.2021.108384.
- J. P. S. Wong, N. Carslaw, R. Zhao, S. Zhou and J. P. D. Abbatt, Observations and Impacts of Bleach Washing on Indoor Chlorine Chemistry, Indoor Air, 2017, 27(6), 1082–1090, DOI:10.1111/ina.12402.
- J. M. Mattila, P. S. J. Lakey, M. Shiraiwa, C. Wang, J. P. D. Abbatt, C. Arata, A. H. Goldstein, L. Ampollini, E. F. Katz, P. F. DeCarlo, S. Zhou, T. F. Kahan, F. J. Cardoso-Saldaña, L. H. Ruiz, A. Abeleira, E. K. Boedicker, M. E. Vance and D. K. Farmer, Multiphase Chemistry Controls Inorganic Chlorinated and Nitrogenated Compounds in Indoor Air during Bleach Cleaning, Environ. Sci. Technol., 2020, 54(3), 1730–1739, DOI:10.1021/acs.est.9b05767.
- J. Jiang, X. Ding, A. Tasoglou, H. Huber, A. D. Shah, N. Jung and B. E. Boor, Real-Time Measurements of Botanical Disinfectant Emissions, Transformations, and Multiphase Inhalation Exposures in Buildings, Environ. Sci. Technol. Lett., 2021, 8(7), 558–566, DOI:10.1021/acs.estlett.1c00390.
- C. W. White and J. G. Martin, Chlorine Gas Inhalation: Human Clinical Evidence of Toxicity and Experience in Animal Models, Proc. Am. Thorac. Soc., 2010, 7(4), 257–263, DOI:10.1513/pats.201001-008SM.
- H. Schwartz-Narbonne, C. Wang, S. Zhou, J. P. D. Abbatt and J. Faust, Heterogeneous Chlorination of Squalene and Oleic Acid, Environ. Sci. Technol., 2019, 53(3), 1217–1224, DOI:10.1021/acs.est.8b04248.
- G. Kampf, D. Todt, S. Pfaender and E. Steinmann, Persistence of Coronaviruses on Inanimate Surfaces and Their Inactivation with Biocidal Agents, J. Hosp. Infect., 2020, 104(3), 246–251, DOI:10.1016/j.jhin.2020.01.022.
-
World Health Organization, Cleaning and disinfection of environmental surfaces in the context of COVID-19: Interim guidance, https://www.who.int/publications-detail-redirect/cleaning-and-disinfection-of-environmental-surfaces-inthe-context-of-covid-19, accessed 2021-11-17.
-
The National Institute for Occupational Safety and Health (NIOSH), NIOSH Pocket Guide to Chemical Hazards - Hydrogen peroxide. https://www.cdc.gov/niosh/npg/npgd0335.html, accessed 2021-07-29.
- G. Hesam, M. Vahabi Shekarloo, A. Atamaleki, M. Jalali, B. Hajipour-Verdom and Z. Moradpour, Health Risk Assessment of Inhalation Exposure to Dry Fogging of Hydrogen Peroxide in a Dental Clinic during the COVID-19 Pandemic, Environ. Sci. Pollut. Res., 2022 DOI:10.1007/s11356-022-21174-1.
- S. F. Kowal, S. R. Allen and T. F. Kahan, Wavelength-Resolved Photon Fluxes of Indoor Light Sources: Implications for HO x Production, Environ. Sci. Technol., 2017, 51(18), 10423–10430, DOI:10.1021/acs.est.7b02015.
- S. Zhou, Z. Liu, Z. Wang, C. J. Young, T. C. VandenBoer, B. B. Guo, J. Zhang, N. Carslaw and T. F. Kahan, Hydrogen Peroxide Emission and Fate Indoors during Non-Bleach Cleaning: A Chamber and Modeling Study, Environ. Sci. Technol., 2020, 54(24), 15643–15651, DOI:10.1021/acs.est.0c04702.
- Z. Wang, S. F. Kowal, N. Carslaw and T. F. Kahan, Photolysis-Driven Indoor Air Chemistry Following Cleaning of Hospital Wards, Indoor Air, 2020, 30(6), 1241–1255, DOI:10.1111/ina.12702.
- Y. Won, M. Waring and D. Rim, Understanding the Spatial Heterogeneity of Indoor OH and HO 2 Due to Photolysis of HONO Using Computational Fluid Dynamics Simulation, Environ. Sci. Technol., 2019, 53(24), 14470–14478, DOI:10.1021/acs.est.9b06315.
- P. S. J. Lakey, Y. Won, D. Shaw, F. F. Østerstrøm, J. Mattila, E. Reidy, B. Bottorff, C. Rosales, C. Wang, L. Ampollini, S. Zhou, A. Novoselac, T. F. Kahan, P. F. DeCarlo, J. P. D. Abbatt, P. S. Stevens, D. K. Farmer, N. Carslaw, D. Rim and M. Shiraiwa, Spatial and Temporal Scales of Variability for Indoor Air Constituents, Commun. Chem., 2021, 4(1), 110, DOI:10.1038/s42004-021-00548-5.
- S. Zhou and T. F. Kahan, Spatiotemporal Characterization of Irradiance and Photolysis Rate Constants of Indoor Gas-Phase Species in the UTest House during HOMEChem, Indoor Air, 2022, 32(1), e12964, DOI:10.1111/ina.12966.
- A. D. Stubbs, M. Lao, C. Wang, J. P. D. Abbatt, T. C. VandenBoer and T. F. Kahan, Near-Source Hypochlorous Acid Emissions from Indoor Bleach Cleaning, Environ. Sci.: Processes Impacts, 2023, 25, 56–65 RSC.
- T. C. Furlani, P. R. Veres, K. E. R. Dawe, J. A. Neuman, S. S. Brown, T. C. VandenBoer and C. J. Young, Validation of a New Cavity Ring-down Spectrometer for Measuring Tropospheric Gaseous Hydrogen Chloride, Atmos. Meas. Tech., 2021, 14(8), 5859–5871, DOI:10.5194/amt-14-5859-2021.
- T. C. Furlani, R. Ye, J. Stewart, L. R. Crilley, P. M. Edwards, T. F. Kahan and C. J. Young, Development and validation of a new in situ technique to measure total gaseous chlorine in air, Atmos. Meas. Tech., 2023, 16, 181–193, DOI:10.5194/amt-16-181-2023.
- D. Poppendieck, H. Hubbard and R. L. Corsi, Hydrogen Peroxide Vapor as an Indoor Disinfectant: Removal to Indoor Materials and Associated Emissions of Organic Compounds, Environ. Sci. Technol. Lett., 2021, 8(4), 320–325, DOI:10.1021/acs.estlett.0c00948.
- R. Reichman, E. Shirazi, D. G. Colliver and K. G. Pennell, US Residential Building Air Exchange Rates: New Perspectives to Improve Decision Making at Vapor Intrusion Sites, Environ. Sci.: Processes Impacts, 2017, 19(2), 87–100, 10.1039/C6EM00504G.
- S. Zhou, C. J. Young, T. C. VandenBoer and T. F. Kahan, Role of Location, Season, Occupant Activity, and Chemistry in Indoor Ozone and Nitrogen Oxide Mixing Ratios, Environ. Sci.: Processes Impacts, 2019, 21(8), 1374–1383, 10.1039/C9EM00129H.
- Y. Liu, P. K. Misztal, J. Xiong, Y. Tian, C. Arata, W. W. Nazaroff and A. H. Goldstein, Detailed Investigation of Ventilation Rates and Airflow Patterns in a Northern California Residence, Indoor Air, 2018, 28(4), 572–584, DOI:10.1111/ina.12462.
- W. W. Nazaroff, Residential Air-change Rates: A Critical Review, Indoor Air, 2021, 31(2), 282–313, DOI:10.1111/ina.12785.
- C. Howard-Reed, L. A. Wallace and W. R. Ott, The Effect of Opening Windows on Air Change Rates in Two Homes, J. Air Waste Manage. Assoc., 2002, 52(2), 147–159, DOI:10.1080/10473289.2002.10470775.
-
U.S. Environmental Protection Agency (EPA), List N: Disinfectants for Coronavirus (COVID-19), https://cfpub.epa.gov/wizards/disinfectants/, accessed 2021-12-20.
- C. J. Weschler, Ozone in Indoor Environments: Concentration and Chemistry: Ozone in Indoor Environments, Indoor Air, 2000, 10(4), 269–288, DOI:10.1034/j.1600-0668.2000.010004269.x.
- W. W. Nazaroff and C. J. Weschler, Indoor Ozone: Concentrations and Influencing Factors, Indoor Air, 2022, 32, e12942, DOI:10.1111/ina.12942.
- E. Reidy, B. P. Bottorff, C. M. F. Rosales, F. J. Cardoso-Saldaña, C. Arata, S. Zhou, C. Wang, A. Abeleira, L. Hildebrandt Ruiz, A. H. Goldstein, A. Novoselac, T. F. Kahan, J. P. D. Abbatt, M. E. Vance, D. K. Farmer and P. S. Stevens, Measurements of Hydroxyl Radical Concentrations during Indoor Cooking Events: Evidence of an Unmeasured Photolytic Source of Radicals, Environ. Sci. Technol., 2023, 57(2), 896–908, DOI:10.1021/acs.est.2c05756.
- C. J. Young, S. Zhou, J. A. Siegel and T. F. Kahan, Illuminating the Dark Side of Indoor Oxidants, Environ. Sci.: Processes Impacts, 2019, 21(8), 1229–1239, 10.1039/C9EM00111E.
- J. Liu, S. Li, J. Zeng, M. Mekic, Z. Yu, W. Zhou, G. Loisel, A. Gandolfo, W. Song, X. Wang, Z. Zhou, H. Herrmann, X. Li and S. Gligorovski, Assessing Indoor Gas Phase Oxidation Capacity through Real-Time Measurements of HONO and NOx in Guangzhou, China, Environ. Sci.: Processes Impacts, 2019, 21(8), 1393–1402, 10.1039/C9EM00194H.
- E. Gomez Alvarez, D. Amedro, C. Afif, S. Gligorovski, C. Schoemaecker, C. Fittschen, J.-F. Doussin and H. Wortham, Unexpectedly High Indoor Hydroxyl Radical Concentrations Associated with Nitrous Acid, Proc. Natl. Acad. Sci. U. S. A., 2013, 110(33), 13294–13299, DOI:10.1073/pnas.1308310110.
- V. Bartolomei, E. Gomez Alvarez, J. Wittmer, S. Tlili, R. Strekowski, B. Temime-Roussel, E. Quivet, H. Wortham, C. Zetzsch, J. Kleffmann and S. Gligorovski, Combustion Processes as a Source of High Levels of Indoor Hydroxyl Radicals through the Photolysis of Nitrous Acid, Environ. Sci. Technol., 2015, 49(11), 6599–6607, DOI:10.1021/acs.est.5b01905.
- S. Zhou, C. J. Young, T. C. VandenBoer, S. F. Kowal and T. F. Kahan, Time-Resolved Measurements of Nitric Oxide, Nitrogen Dioxide, and Nitrous Acid in an Occupied New York Home, Environ. Sci. Technol., 2018, 52(15), 8355–8364, DOI:10.1021/acs.est.8b01792.
- S. Gligorovski, Nitrous Acid (HONO): An Emerging Indoor Pollutant, J. Photochem. Photobiol., C, 2016, 314, 1–5, DOI:10.1016/j.jphotochem.2015.06.008.
- C. Wang, D. B. Collins and J. P. D. Abbatt, Indoor Illumination of Terpenes and Bleach Emissions Leads to Particle Formation and Growth, Environ. Sci. Technol., 2019, 53(20), 11792–11800, DOI:10.1021/acs.est.9b04261.
- K. E. R. Dawe, T. C. Furlani, S. F. Kowal, T. F. Kahan, T. C. VandenBoer and C. J. Young, Formation and Emission of Hydrogen Chloride in Indoor Air, Indoor Air, 2019, 29(1), 70–78, DOI:10.1111/ina.12509.
Footnotes |
† Electronic supplementary information (ESI) available. See DOI: https://doi.org/10.1039/d2em00434h |
‡ Currently at Department of Earth and Atmospheric Sciences, University of Houston, Houston, TX, USA. |
|
This journal is © The Royal Society of Chemistry 2023 |