DOI:
10.1039/D2EA00156J
(Critical Review)
Environ. Sci.: Atmos., 2023,
3, 782-798
Marine aerosol feedback on biogeochemical cycles and the climate in the Anthropocene: lessons learned from the Pacific Ocean
Received
17th November 2022
, Accepted 11th April 2023
First published on 17th April 2023
Abstract
Human activities have profoundly altered the air quality and the climate on a global scale in the Anthropocene. It is our task to quantitatively evaluate the impact of human activities on marine ecosystems and the climate through various feedbacks in biogeochemical cycles. Atmospheric aerosols over the Pacific Ocean are largely influenced by anthropogenic (e.g., metal production, fossil fuel combustion, and agriculture), marine and terrestrial biogenic, pyrogenic (open biomass burning), and lithogenic (mineral dust) sources. To what extent do oceanic (sea salt) and marine biogenic emissions of aerosols and their precursor gases change the marine cloud properties under the influence of anthropogenic and biogeochemical activities and thereby affect the climate? At the same time, to what extent does atmospheric deposition of nutrients change marine biogeochemistry under the influence of anthropogenic and terrestrial biogeochemical activities and thereby affect marine ecosystems? We summarize the progress in research on organic aerosols, nitrogen, and iron in the atmosphere over the Pacific Ocean. Future perspectives include interdisciplinary research of field observational, laboratory experimental, and numerical modeling studies.
Environmental significance
Marine aerosol feedback on biogeochemical cycles and the climate remains highly uncertain due to the complex interplay. Earth system models need to incorporate more realistic representations of biogeochemical feedback processes in response to the changes in air quality and the climate due to human activities. This review highlights recent advances in our understanding of organic aerosols, nitrogen, and iron as well as cloud properties and marine biogeochemistry in the Pacific Ocean. International collaborations of field observational, laboratory experimental, and numerical modeling studies are essential to foster individual studies into cross-disciplinary research and to inform policymakers toward ocean sustainability.
|
1. Introduction
Rapid expansion of human activities has profoundly transformed Earth's atmosphere, ushering in an era known as the Anthropocene.1 It is important to understand and assess the impacts of those anthropogenic environmental changes on interactions between atmospheric chemistry and biogeochemistry as well as climate feedbacks induced by changes in marine ecosystems (Fig. 1).2 Particularly at high latitudes, the ice-albedo feedback is induced by a decrease in reflected sunlight to space due to the reduction of snow and ice surfaces, resulting in rapid warming (i.e., positive climate feedback). Concurrently, understanding the complex processes in aerosol–cloud interactions is urgently needed to reduce uncertainty in near-term projection of climate change.3
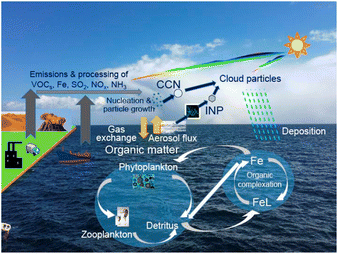 |
| Fig. 1 Schematic representation of marine aerosol feedback on biogeochemical cycles and the climate. Modified from ref. 2 under CC BY 4.0 with permission from Springer Nature, Copyright © The Author(s) 2021. After aerosols fall into the surface ocean, bioaccessible Fe binds with organic ligands (FeL), enters the biogeochemical cycle, or is removed via the scavenging process. | |
An increase in a transient temperature induces an increase in the optical thickness of clouds due to the phase change process from ice clouds to liquid clouds (i.e., negative climate feedback).4 However, the strength of the negative feedback depends on the treatment of mixed-phase clouds with a mixture of cloud condensation nuclei (CCN) and ice-nucleating particles (INPs) in Earth system models.5 In addition to such physical feedbacks, CCN and INP number concentrations in the atmosphere are modulated by aerosols and their precursors of marine biogenic origin.6,7 As a result of various responses of marine biogeochemical activities to increased sea surface temperatures and melting sea ice, the cloud properties in the atmosphere are expected to change and provide feedbacks to climate change. On the other hand, in areas where phytoplankton growth is primarily limited by nutrient deficiency, nutrients supplied by aerosols and precipitation stimulate the growth of phytoplankton communities. Furthermore, an increase in large-scale forest fires due to climate change is projected to increase nutrient supply to the oceans. At the same time, nutrient deposition from industrial and agricultural sources is projected to increase with expected economic growth in some countries to enhance phytoplankton productivity in specific regions of the ocean. This is, in turn, expected to enhance reactive gas and aerosol emissions and the carbon dioxide (CO2) sink by marine biogeochemical activities, thereby acting as negative climate feedback and vice versa. Therefore, in order to consider the effects of climate change on the Earth system, it is necessary to improve our understanding of how these air pollutants and greenhouse gases affect the climate through interactions with terrestrial and marine ecosystems and their biogeochemical activities.
Earth system models that include biogeochemical processes on top of climate models are used to quantitatively assess the effects of climate change and air pollution on the Earth system.8,9 However, there is a large degree of uncertainty in predicting the effects of oceanic (sea salt) and marine biogenic emissions on the climate and the effects of atmospheric deposition of nutrients from anthropogenic (e.g., metal production, fossil fuel combustion, and agriculture), lithogenic (mineral dust), and pyrogenic (open biomass burning) sources on marine ecosystems. Therefore, it is crucial to integrate observational, experimental, and numerical modelling studies to elucidate feedbacks induced by marine aerosols in biogeochemical cycles and climate change in the Anthropocene.
Climate feedbacks via atmospheric aerosols and marine ecosystems over the oceans have been actively studied, mainly on the basis of hypotheses to elucidate aerosol–cloud interactions.9 It has been suggested that increases in dimethyl sulfide (DMS) emission fluxes from marine biogenic sources induce negative climate feedback on warming the climate and vice versa.10 On the other hand, it has been proposed that increases in iron (Fe) deposition fluxes from lithogenic sources enhance the sink of atmospheric CO2 into the ocean during the Last Glacial Minimum (LGM).11 Subsequently, the Fe hypothesis led to a dramatic development in Fe research, focusing on the evaluation of Fe deposition from atmospheric aerosols.10 In addition to Fe, anthropogenic reactive nitrogen (N) (oxidized and reduced inorganic and organic compounds) deposition has been suggested to support up to ∼3% of the marine biological production but this estimate has been revised down to ∼0.4%.12,13
In recent decades, observations in the marine boundary layer (MBL), laboratory studies, and modelling efforts on new particle formation from DMS have led to revaluation of the complex interplay, including organic matter (OM).14 On the other hand, global ocean biogeochemistry models have suggested that the nutrient fertilization effects of atmospheric deposition are limited due to negative biogeochemical feedbacks.15,16 Furthermore, it has been suggested that the majority of ocean primary production in the contemporary climate cannot be explained by Fe supply from lithogenic aerosols only.17
Atmospheric chemistry at the interface between the atmosphere and ocean is governed by the complex interactions of various atmospheric and oceanic processes. In order to gain insight into these processes, this review focuses on the marine aerosols of OM, N, and Fe over the Pacific Ocean. The Pacific Ocean has the largest area of the world ocean (47%), whereas it takes up only 33% of the global CO2 flux mainly due to the large CO2 source flux along the equatorial Pacific during non-El Niño events.18 The subarctic North Pacific is known as the high nutrient, low chlorophyll (HNLC) region where biological production is primarily limited by Fe availability and is controlled by both the atmospheric Fe supplies and oceanic Fe cycles.19 The Pacific Ocean is the region where substantial amounts of dissolved Fe are received from anthropogenic, pyrogenic, and lithogenic Fe sources.2 Indeed, the 2019–2020 Australian megafires supply pyrogenic Fe with higher bioaccessibility than lithogenic Fe and trigger widespread phytoplankton growth in HNLC regions of the South Pacific.20,21
In the following, this review is organized into two main sections: aerosols and their precursor gases emitted from the ocean and their impacts on the climate in Section 2 and nutrients supplied from the atmosphere and their impacts on marine biogeochemistry in Section 3. The review concludes with a summary and directions for future research to be addressed in the interdisciplinary research of atmospheric chemistry and marine biogeochemistry at the air–sea interface.
2. Aerosols and their precursor gases emitted from oceans and their impacts on climate
To better predict the climate impact of marine aerosols using Earth system models, it is essential to understand the biological, chemical, and physical processes and to find optimal parameterization of sea-to-air emission fluxes of aerosols and their precursors (Section 2.1) and subsequent activities of CCN (Section 2.2) and INPs (Section 2.3).
2.1 Aerosols and their precursor gases emitted from oceans
Shipboard measurements have shown that seasonal variation of primary production in the subarctic North Pacific is larger than that in other oceanic regions.18 In the subarctic North Pacific, microbial dynamics (mainly driven by phytoplankton) differ between eastern and western areas, which is closely linked with the difference in the amount of nutrient supply/consumption and air–sea flux of CO2 between the two areas.22 The amount, compositions, and physicochemical properties of reactive gases and aerosols provided from the sea surface to the atmosphere and associated with microbial activity largely depend on prosperity and decline of phytoplankton and origin of OM in surface seawater.23 Consequently, the subarctic North Pacific is an important oceanic region in terms of evaluating sea-to-air emissions of reactive gases and aerosols and the subsequent climate effect. The Western Pacific Air–Sea interaction Study (WPASS) project in the late 2000s was the driving force behind the academic development of the Surface Ocean-Lower Atmosphere Study (SOLAS) in Japan (https://solas.jp/english/).24
To estimate the emission flux of aerosols from ocean surfaces to the atmosphere, sea spray aerosol (SSA) flux and particle size distributions are empirically expressed as functions of surface wind speeds.25,26 Indeed, measurements have shown that the frequency of occurrence of whitecaps tends to increase with increasing surface wind speeds. Variation in estimates of the whitecap fraction as a function of wind speed contributes additional, comparable uncertainty to emission flux estimates, indicating that the emission flux may depend on quantities which are not accounted for in current models.27 Ovadnevaite et al.28 parameterized a new sea spray source function in terms of five lognormal modes and the Reynolds number instead of the more commonly used wind speed, which resulted in better agreement with the observed results. However, those estimates did not take account of OM as a source function.
There is still a lack of understanding of factors that control chemical and physical properties of OM in marine aerosols (e.g., compositions and surface activity of dissolved OM and particulate OM in surface seawater; species, numbers, and activity of microbes, etc.). The amount of OM in SSAs has been expressed as a function of the concentration of chlorophyll a, which is used as a proxy for phytoplankton biomass.29 In such cases, amounts of chlorophyll a are derived from satellite observations and/or Earth system models.30,31
To illustrate the annually averaged contribution of primary organic aerosols (POAs) from sea spray to the total OA concentration over the Pacific, the comparison with POAs from anthropogenic and pyrogenic sources is shown in Fig. 2. Here, we use the Integrated Massively Parallel Atmospheric Chemical Transport (IMPACT) model to estimate the source profile of marine aerosols over the Pacific.32 In the model,20 the POA emissions from the sea surface are calculated as a function of the chlorophyll a concentration, wind speed, sea surface temperature, and aerosol diameter.33 The spatial distribution shows that POAs from sea spray comprise the majority of OAs over the tropical and subarctic North Pacific while POAs from continental sources contribute to the dominant sources over the regions of continental outflow.34,35
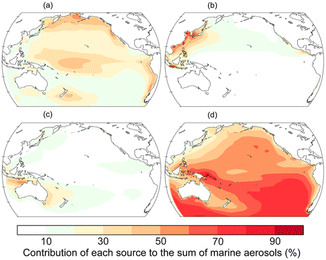 |
| Fig. 2 Relative percentage contribution of POAs from (a) sea spray, (b) anthropogenic, and (c) pyrogenic sources and (d) SOAs to the annually averaged OA mass concentration near the surface ocean in 2019.32 The annual emission fluxes from marine biogenic (0.2 Tg C per year for isoprene and 8.6 Tg C per year for glyoxal) and shipping (0.1 Tg C per year) sources are estimated in the Pacific. | |
Despite that microbial population and community composition vary with phytoplankton bloom, the organic carbon content of SSAs is weakly correlated with satellite-derived measurements of chlorophyll a levels downwind of plankton bloom.36 As one possible explanation, shipboard measurements of aerosols and surface seawater in the subarctic western Pacific demonstrated that the changes in chemical composition occur as a result of freshly injected dissolved organic carbon (DOC) into the atmosphere, which is chemically and/or biologically modified on a timescale of a half to one day.37 Furthermore, the amount of OM was suggested to be closely linked with and partially controlled by senescent algal cells and/or cell lysis (Fig. 3).38
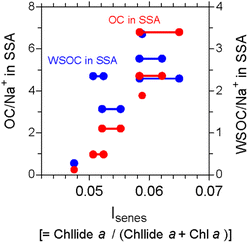 |
| Fig. 3 The organic carbon (OC)/sodium (Na+) and water-soluble OC (WSOC)/Na+ ratios in the SSA samples as functions of an index that represents the senescent status of marine phytoplankton (Isenes) in surface seawater observed in the western North Pacific. The number of data points in the panels is more than six of the SSA samples. The individual surface seawater data points corresponding to the identical aerosol sampling data are connected with a straight line. Adopted from ref. 38 under CC BY 4.0 with permission from Springer Nature, Copyright © The Author(s) 2020. | |
Online shipboard observations of fluorescent aerosol particles over the central Pacific Ocean showed that the number concentration level of atmospheric bioaerosols (5–30 L−1)39 was similar to that in the Kuroshio Extension (10–250 L−1)40 which is the North Pacific western boundary current. Kawana et al.39 suggested empirical equations to estimate bioaerosol number density in the atmosphere by using biogenic proxies (chlorophyll a, transparent exopolymer particles (TEPs), and bacteria) and surface wind speeds (Fig. 4).
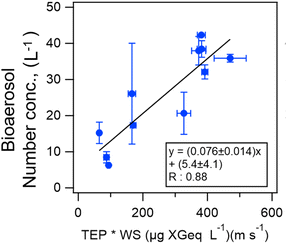 |
| Fig. 4 Scatter plot and equations of number concentrations of bioaerosols in the atmosphere as functions of the product of wind speed (WS) and the biomarker of the TEP concentration over the central Pacific. The black line represents the orthogonal regression line and the error bars represent a standard deviation. Adopted from ref. 39 under CC BY 4.0 with permission from Copernicus Publications, Copyright © The Author(s) 2021. | |
These biological and physicochemical transformation processes of aerosols at the air–sea interface are still keys to our understanding of physicochemical properties of SSAs. To elucidate the climate impact of OM associated with marine microbial activity, further studies are needed to understand the amount, compositions, mixing states, and reactivity of SSAs including inorganic matter.41 It is also required to quantitatively understand factors and mechanisms that determine selectivity of compositions during sea-to-air transfer, which should be linked with biological processes on the ocean surface.
In addition to POAs, the annually averaged contribution of secondary OAs (SOAs) to the total concentration of OAs over the Pacific is shown in Fig. 2(d). Natural SOAs are produced from the oxidation of isoprene, terpenes, and DMS.34,35 In the model, the concentrations of isoprene in the surface ocean layer are calculated from the empirical relationship between the isoprene concentration and chlorophyll a concentration for two separate sea surface temperature bins.42 The sea surface DMS concentration is obtained from the monthly climatological data set.43 The net flux from the ocean to the atmosphere is then calculated from the concentration gradient for isoprene and DMS across the air–sea interface and the transfer velocity.44,45 An extra glyoxal source of 20 Tg C per year over the oceans46 is evenly distributed in a mixing ratio throughout the planetary boundary layer by scaling with marine isoprene sources.50 The photochemical oxidation of volatile organic compounds (VOCs) and their subsequent reaction in the atmosphere form oxidized organic gases in the atmosphere.47 In the presence of cloud droplets or aqueous particles, water-soluble gases (e.g., glyoxal, methylglyoxal, and glycolaldehyde) dissolve in the aqueous phase and are further oxidized to form products with lower volatility (e.g., dicarboxylic acids and oligomers).48 These low volatility products are assumed to remain entirely in the particulate phase as aqueous SOA (aqSOA) compounds (i.e., glyoxylic acid, pyruvic acid, oxalic acid, and two classes of oligomers) when cloud water is evaporated.49 The aqSOA mass concentration formed in cloud water is proportionally distributed to the aqSOA mass concentration on preexisting aerosols. The aqSOA is also formed in externally mixed wet aerosols.50 The surface-limited uptake process is considered for glyoxal and methylglyoxal in sulfate aerosols only.51 The spatial distribution shows that SOAs from marine and terrestrial sources are the majority of OAs over the open ocean (Fig. 2(d)).
Large uncertainty exists on how VOCs other than DMS52 or organic N, which are emitted from ocean surfaces, play a role in new particle formation, secondary aerosol formation, and physicochemical transformation of preexisting particles. Indeed, Thames et al.53 suggested the presence of unmeasured or unknown VOCs and/or oxygenated VOCs associated with ocean emissions. Furthermore, possible effects of nitrogen fixation, which converts dinitrogen (N2) gas to ammonia (NH3), on atmospheric emissions of reactive N (including organic N) have been investigated.54 The atmospheric emission fluxes of organic N or NH3 (or ammonium) from the sea surface are important in terms of estimating atmospheric deposition fluxes of anthropogenic reactive N.55 Reactive gas species (e.g., VOCs or reactive N), which are photochemically produced at the air–sea interface, have been suggested to be involved in new particle formation, although large uncertainties exist in their amount.56 It is important to elucidate the emission flux of the carbon mass of primary emissions by SSAs as well as that of SOAs and to reveal their importance relative to the number and mass of sea salt.
To quantitatively associate the composition of aerosols with biological and physicochemical processes in the ocean, it is essential to understand the processes at molecular levels that potentially contain information about metabolic pathways and the reaction path of producing OM in seawater. This also needs establishing methods for quantification of OM that transfers from the ocean surface to the atmosphere.
2.2 Impact of aerosols on cloud condensation nuclei
The CLAW hypothesis postulated that new particle formation driven by biogenic DMS might have been involved in stabilizing global temperature through modifications of cloud albedo.6 Since the CLAW hypothesis was put forward, the role of DMS in the atmosphere has received substantial attention with respect to global warming. However, aerosol particle formation from DMS as a precursor gas was extremely rare in the MBL where sea salt particles were abundant as preexisting particles.57 On the other hand, to mimic natural marine systems in laboratory experiments, mesocosm experiments have been promoted to artificially reproduce waves and generate SSAs using large waving tanks.58 The results from laboratory experiments suggest that secondary marine aerosols in the presence of OM play a dominant role in affecting submicrometer SSAs, the hygroscopicity of aged SSAs, and thus cloud-forming ability.59 The feedback between marine biological activity and the climate is much more complex than that proposed in the CLAW hypothesis.14 Thus, the individual processes that constitute the CLAW hypothesis remain controversial.
Laboratory experiments suggest that in the pristine atmosphere, the intramolecular hydrogen atom transfer reaction is important in the oxidation process of DMS.60 The relatively stable intermediate HPMTF (hydroperoxymethyl thioformate; HOOCH2SCHO) produced in the process was observed by aircraft measurements over the ocean, confirming the importance of its isomerization reaction.61 Subsequently, numerical modelling studies, which consider isomerization reactions, indicate the need for a better understanding of the dominant processes of new particle formation in the atmosphere and the cloud response to that change.62,63 In addition, Earth system models disagree on the direction of the future trend of the global annual mean DMS concentration.64 The climate-adjusting ability of marine DMS requires further study.
Recent large-scale airborne observations have captured new particle formation sustained in the tropical free troposphere, where preexisting particles and anthropogenic precursor gases are scarce.65 It is postulated that the precursor gas in the MBL is transported to the free troposphere by convective clouds, leading to the formation of new particles, and that the entrainment of the newly generated particles into the MBL contributes to cloud formation in the MBL. However, field observational and modelling studies suggest that additional precursor gases other than DMS such as VOCs are needed to be taken into account, since the particle number concentration is underestimated when only DMS is considered as a precursor gas.14
The role of marine organic aerosols in CCN has attracted attention since the 2000s.23 Field observations and laboratory experiments suggest that hydrocarbons and colloidal substances might significantly contribute to the reduction of hygroscopicity for SSAs and lead to the suppression of CCN activity.31 However, the results from microcosm experiments suggest that changes in chemical composition of SSAs associated with biological activity do not strongly affect the hygroscopicity of primary SSAs.66 Alternatively, OM coating on SSAs reduces the surface tension, enhances the emission flux of the particle number concentration, and thus the CCN concentration.67 Moreover, SSA number size distribution derived from size-resolved hygroscopicity and particle number size distribution indicates that the contribution of submicrometer SSAs to marine CCN might have been underestimated in previous studies.68 These studies highlight the need for further identification of submicrometer SSAs to quantify the source apportionment of CCN in the atmosphere more accurately.
In climate models, empirical thresholds are used when aerosol number concentrations are converted to CCN concentrations to compensate for underestimation of natural aerosol number concentrations and to reduce the cooling effect of aerosol–cloud interactions induced by anthropogenic aerosols.69 Therefore, perturbation of the marine organic aerosol concentration below that threshold has no effect on the climate.30 To estimate the anthropogenic effects on aerosol–cloud interactions more accurately, it is necessary to improve our understanding of marine organic aerosols and to develop models that do not use empirical thresholds. To understand the effects of marine biology on the climate, a parameterization for global models is proposed that uses an empirical relationship between the number fluxes of SSA-derived CCN and seawater nanophytoplankton cell abundances that can be observed from satellites.70 The climate model MRI-ESM2 (Meteorological Research Institute Earth System Model version 2) used SSA-derived CCN as a tuning parameter to adjust the cloud albedo effect to suppress excessive solar radiation over the remote ocean.71
2.3 Impact of aerosols on ice-nucleating particles
In general, freezing of supercooled water droplets requires conditions as low as −40 °C in the absence of impurities, but the presence of INPs promotes freezing at relatively high temperatures (−5 to −25 °C). Through such pronounced ice-nucleation activity, it has been postulated that biogenic primary particles (bioaerosols) may have a substantial impact on the life cycle of clouds in the atmosphere, the dynamics of the cloud-precipitation system, and the radiation budget. Even if the number of INPs in the total aerosol concentration is very small (e.g., one INP in one million particles), they play a pivotal role in the depletion of liquid clouds via several microphysical pathways. Thus, a quantitative understanding of their number concentration and INP activity is needed. However, even qualitative knowledge is limited, and we are far from understanding, quantifying, and modelling the detailed processes.
Bioaerosols (i.e., bacteria, small phytoplankton, their debris, and exudates) are injected from the sea surface to the atmosphere as part of SSAs. The emission flux of bacteria is estimated to be 2–10 Tg per year with a wide range of uncertainty.72 The bacteria aerosol may include ice-nucleation active species. The surface of a marine diatom species73 and organic material associated with phytoplankton cell exudates7 have been shown to promote freezing under conditions relevant for mixed-phase clouds. Aerosol particles, which are active as INPs, have been suggested to be of submicrometer size.7,74,75 On the other hand, the majority of INPs, which originated from freshly produced biological materials entrained in jet drop SSAs, are of supermicrometer size.76 Laboratory studies on marine phytoplankton suggest that both biomolecules and microbes contribute to marine INPs within SSAs.77
A cloud-resolving model suggests that low marine-derived INPs, compared to terrestrial mineral aerosols, suppress the overestimation of ice clouds and thus complement the underestimation of water clouds over remote oceans.78 The contribution to INP concentrations is calculated using the parameterization derived from samples in the sea surface microlayer.7 Thus, physiochemical selectivity in the transfer of OM into the aerosol phase is not accounted for in this parameterization. To account for the effects of chemically selective emission and atmospheric processing implicitly, a parameterization is empirically derived from observations of ambient SSAs.79 In global model studies, the latter parameterization better reproduces INPs associated with marine sources compared to the former parameterization.80,81 Furthermore, coarse and super-coarse dust particles can be transported farther away from the land, and thus the abundance of these particles is substantially underestimated in current models.82 Thus, the relative importance of marine OAs as a source of INPs in comparison to terrestrial sources and their effect on mixed-phase clouds are still open questions. These studies highlight the need for further identification of chemical composition for larger particle sizes to quantify the source apportionment of INPs in the atmosphere more accurately.
Comprehensive observational and modelling studies are needed to elucidate the processes which affect the emission flux of OM in surface seawater to the atmosphere, the INP activity, and the effects of INPs on cloud and precipitation systems. For comprehensive observation, interdisciplinary measurements of oceanic and atmospheric INPs and marine biogeochemistry using ships and aircraft are desirable.
Oceanographic research vessel “Mirai” has conducted automatic continuous measurement of fluorescent atmospheric particles on many research cruises as a unique attempt since 2012. Simultaneous INP measurement has also been conducted on some cruises. A positive correlation between bioaerosol number concentrations and transparent exopolymer particles (TEPs), which are produced from phytoplankton exudations in seawater, has been found (Fig. 4).39 The relationship between INPs and indicators of marine biogeochemical activity is not only limited to chlorophyll a, but also to the number and species of bacteria, TEPs, and CSPs (proteinaceous material), which are useful for clarifying bioaerosol formation processes.83 The relationship between those indicators was shown using the results of incubation experiments as well as field observations.84 However, the formation and loss processes and particle sizes of related marine materials remain unresolved, and a laboratory approach, including incubation experiments, is effective.
Aerosol–cloud interaction studies have been intensively conducted over high-latitude oceans, since the model biases in solar (shortwave: SW) and thermal (longwave: LW) radiation are associated with low-level mixed-phase clouds.85 Over the remote ocean, the number concentration of INPs frozen at −15 °C (NINP (−15 °C)) was once reported to be as high as 3–250 m−3.86 However, recent ship-based surveys have observed a much lower order of magnitude of 0.1–1 m−3.87–89 Globally collected offshore INP data also showed NINP (−15 °C) in the range of 0.1–100 m−3, lower than that over the land.90 However, a comprehensive understanding of the relationship between marine and atmospheric bioaerosols and INPs has not yet been achieved, although the predominance of marine organic species has been reported from atmospheric bacterial community analyses over the remote ocean91 as has the correlation between fluorescent particles and INPs.92 The relative contributions of particle sizes (submicrometer or supermicrometer) to INPs are open questions.7,90 Even at mid and low latitudes, there is a large degree of uncertainty in the radiation budget of overhead clouds such as cirrus clouds. Therefore, it is desirable to expand our knowledge on whether marine bioaerosols play an important role in INPs during ice cloud formation.
Future studies should clarify to what extent lithogenic, terrestrial biogenic, pyrogenic and anthropogenic sources also contribute to INPs in the atmosphere,93 as well as their respective roles and relative contributions in conjunction with marine biogenic sources. It is required to evaluate the role of various sources, such as bioaerosols from large-scale wild fires94 and thermally modified mineral aerosols95 in INP activity and how moisture removal associated with long-range transport in the atmosphere affects the INP/CCN ratio in the atmosphere. Observations by unmanned and manned aircraft (including drones and helicopters) and tethered balloons in combination with ships will be effective methods of obtaining information at cloud altitudes. Collaboration with microbiological scientists to elucidate the role of microlayers where OM is enriched in the calm ocean surface layer96 and linkage with environmental DNA coverage analysis are also promising directions.
Climate and weather research should focus on how marine bioparticles, atmospheric bioaerosols, and INPs change with climate change and to what extent they produce positive or negative climate feedbacks. Numerical models97 incorporating detailed processes of marine aerosol emission, aerosol microphysics, CCN/INP activity, aerosol–cloud interaction, and cloud radiation should be used to evaluate each process and provide integrated knowledge.
3. Nutrients supplied from the atmosphere and their impacts on marine biogeochemistry
Plant growth is primarily controlled by the lowest supply of the essential nutrients given (i.e., Liebig's law of least). In areas and seasons where Liebig's law of least is adapted to nutrients in phytoplankton, trace amounts of increased nutrient concentrations lead to enhanced phytoplankton growth.98 Thus, the atmospheric deposition of essential elements such as N and Fe can lead to increased primary production in the ocean. It is known from observation that nutrient deficiency depends on region, season, and other factors. In particular, the chemical form of Fe is a key factor, because water-soluble Fe in aerosols (hereinafter bioaccessible Fe) is more readily bioavailable than insoluble forms such as crystalline Fe oxides in soils. After aerosol deposition into the surface ocean, bioaccessible Fe may: (1) be chelated with organic ligands (FeL), (2) enter the biogeochemical cycle, or (3) be scavenged into sinking particles (Fig. 1). However, since the residence time of nutrients in surface waters varies with the types and forms of nutrients, concentrations of bioavailable nutrients after atmospheric deposition vary spatially and temporally. Therefore, it is essential to quantify the atmospheric deposition of bioaccessible nutrients over a wide area and a long time period. In the following, we use the IMPACT model to estimate the source contribution to the atmospheric deposition of reactive N (Section 3.1) and bioaccessible Fe (Section 3.2).32
3.1 Reactive nitrogen
Reactive N compounds in gases, aerosols, and rain supply macro-nutrients to the ocean. In particular, atmospheric N compounds emitted by human activities are substantially delivered to the downstream oceans of metropolitan areas.13,99 Therefore, the deposition fluxes and contribution of external nutrients to the marine ecosystem have been discussed through field observations, laboratory experiments, and numerical models. However, there are still large uncertainties in the quantification of the fluxes and their effects on marine biogeochemistry.55
The Pacific is the net importer of reactive N from the atmosphere to the oceans.100 We show the contribution of oxidized and reduced N deposition to the total atmospheric supply of reactive inorganic N in the Pacific (Fig. 5).32 Nitrogen oxide (NOx) emissions are mostly derived from anthropogenic and pyrogenic sources whereas NH3 emissions are dominantly derived from agricultural activities on a global scale. Deposition of oxidized N (12.1 Tg N per year) dominates reduced N (7.1 Tg N per year) in the Pacific. Large contribution of nitrate deposition can be seen in downstream regions of fossil fuel combustion and biomass burning whereas N deposition is dominated by reduced N over the tropical and southern Pacific.100
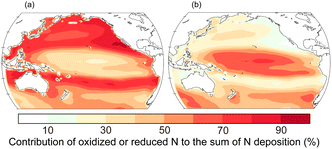 |
| Fig. 5 Contribution of (a) oxidized (12.2 Tg N per year) and (b) reduced (7.2 Tg N per year) deposition to the total atmospheric supply of N into the Pacific in 2019.32 The values in parentheses represent the annual deposition fluxes. The annual emission fluxes from shipping (2.4 Tg N per year for NO) and oceanic (5.0 Tg N per year for NH3) sources are estimated in the Pacific. | |
Combination of an atmospheric chemical transport model and a marine ecosystem model suggests that the atmospheric deposition of inorganic N from East Asia increases marine primary production in the western North Pacific subtropical region.101 Onboard incubation experiments confirm that Chinese haze particles have an overall stimulating effect on phytoplankton growth under representative marine conditions in the western North Pacific Ocean.102 Furthermore, the microcosm experiments suggest that the surplus of reactive N leads to the limitation of phytoplankton growth. In an Fe-containing marine ecosystem model, when a surplus of reactive N is supplied, atmospheric Fe deposition stimulates phytoplankton growth in winter and spring according to Liebig's law of least, with a concomitant increase in particulate organic carbon transport fluxes to the deep sea.103
Sinking and subducted OM is respired in deeper waters and thus the oxygen is consumed in the ocean interior. Numerical model simulations have been conducted to evaluate the effects of pollution-induced enhancement of atmospheric bioaccessible Fe and fixed N deposition on the biological CO2 drawdown and deoxygenation in the tropical Pacific.104 In the Earth system model, anthropogenic nutrient deposition has an offsetting effect on climate change on the climate-induced decrease in CO2 uptake but an accelerating effect on increases in climate-driven deoxygenation since the preindustrial era, while the nutrient effects exceed the climate effects in the North Pacific and in low-latitude regions of the Pacific.105
The field observations have assumed a Redfield ratio to estimate the impact of the deposition flux on marine primary productivity. However, the time scales of lateral transport and the vertical delivery of the nutrients differ in the atmosphere and ocean substantially, making it difficult to comprehensively and quantitatively evaluate the effects of the atmospheric input. Thus, accurate estimates of dry and wet deposition and N fixation from the atmosphere are required in conjunction with the quantitative evaluation of upwelling from the deeper ocean to the surface, vertical eddy diffusion, and lateral transport. The treatment of these differences in temporal and spatial scales is an important issue. Thus, close collaboration between atmospheric and oceanic researchers are needed to elucidate biogeochemical processes in the atmosphere and the ocean.
Various efforts are needed to comprehensively understand the impact of atmospheric deposition of N compounds on marine ecosystems. In field observations, simultaneous observations of atmospheric composition and ocean biogeochemistry should be promoted. Continuous observations should be conducted throughout the year at oceanic sites. A database of dry deposition is established from aerosol measurements averaged over a wide area (5° × 5°) from shipboard observations from 1995 to 2012.106 However, nitrate and ammonium salts in fine particles from anthropogenic sources are highly volatile. Thus, on-line gas and aerosol measurements are simultaneously required for their quantification.107 On the other hand, databases on wet deposition have not been established, due to a scarcity of data. Since large amounts of reactive N may be supplied in a short time period, the impact of wet deposition should be evaluated in field measurements.
The accuracy of the estimation of anthropogenic emissions of reactive N compounds into the atmosphere needs to be improved. In recent years, NOx emissions in China have decreased owing to effective air pollution control.108,109 Thus, the supply of nitrate in the western North Pacific is projected to decrease in the future. On the other hand, agricultural NH3 emissions are projected to increase, but are highly uncertain due to the difficulty of net flux measurements. Accurate estimation of these emissions, including emissions from the ocean and linkage with marine biogeochemistry is a future challenge. Furthermore, changes in atmospheric acidity accompanying changes in emissions of aerosol precursors affect the long-range transport of nutrients and the effects of ocean fertilization.110 Although impact assessments have so far focused on inorganic N due to its high abundance in the atmosphere, it is necessary to accumulate information on the spatiotemporal distribution of organic N and phosphorus (P), including their composition.12,111,112
3.2 Bioaccessible iron
In the subarctic North Pacific, a number of observational studies on dissolved Fe have been conducted to elucidate the distribution and biogeochemical cycles of Fe in the ocean interior.19 An international project, GEOTRACES (An International Study of the Marine Biogeochemical Cycles of Trace Elements and Their Isotopes),113 fosters the investigation of global distribution of trace elements and isotopes and their ocean circulation, which returns nutrient-rich deep waters back to the surface layer. Based on the spatiotemporal distribution of dissolved Fe, an ocean biogeochemistry model suggests a slow removal process of dissolved Fe that brings the basin-scale transport of dissolved Fe from continental shelf sediments into the intermediate layer water in the North Pacific Ocean (particle sedimentation rate: 180–460 m per year).114 On the other hand, quantitative estimation of the dissolved Fe input to phytoplankton communities in the euphotic zone is rather limited. In both rainwater and oceanic microlayers, more studies are needed to investigate the role of organic chelating ligands to protect Fe in a reservoir from loss by scavenging or precipitation when bioaccessible Fe is supplied from the atmosphere.115 At the same time, organic ligands are a product or by-product of marine biological production, leading to a positive feedback between biological production and Fe bioavailability.116,117 Furthermore, when excessive Fe is supplied to the ocean surface layer, nutrient deficiencies other than Fe suppress primary biological production in the ocean. In response to the atmospheric input, however, which nutrients control marine primary production in which areas varies among models because the residence time or recycling rate of nutrients in the ocean surface layer is not well constrained by observations.118,119 Therefore, quantitative evaluation of changes in nutrient concentrations, which are induced by Fe deposition in numerical simulation experiments, requires a better understanding of biogeochemical cycling of both macro- and micro-nutrients over wide regions and long periods. In addition, because of the wide variety of organisms in the ocean, there are multiple trace elements which limit ocean biological production.120 Thus, the effects of micro-nutrients other than Fe on marine biogeochemistry need to be investigated.
An increase in external sources of nutrients supplied to the ocean is projected with an increase in mega forest fires caused by extreme weather events. Oceanic Fe models have been combined with atmospheric Fe models to demonstrate the importance of bioaccessible Fe from pyrogenic sources.2,119 In addition, there are other episodic Fe sources, such as volcanic ash.121,122 However, observational constraints on model responses of phytoplankton growth to atmospheric Fe deposition from such episodic sources are limited. Large-scale fertilization events may be used to constrain dissolved Fe residence time through recycling123 and the role of organic ligands in the feedback between biological production and Fe bioavailability. Therefore, it is desirable to quantify the atmospheric fluxes of nutrient emission, transformation, and deposition during the events in addition to background conditions.
In addition to pyrogenic Fe, there has been growing interest in research on the importance of bioaccessible Fe deposition of anthropogenic Fe and its impact on marine biogeochemistry.2 Observational data show an inverse relationship in which a lower aerosol Fe concentration is associated with higher Fe bioaccessibility (Fig. 6).124,125 When this inverse relationship is expressed on a logarithmic axis, no single conclusion can be drawn from the analysis of a simple one-dimensional model among the proposed hypotheses.126 On the other hand, this inverse relationship can be replicated using random data.115 In other words, meaningful conclusions cannot be drawn solely from the inverse relationship, since the total Fe concentration (horizontal axis) is the denominator of Fe bioaccessibility (vertical axis). However, an international project combining multiple atmospheric Fe models and field observational data draws one conclusion from several proposed hypotheses to explain this inverse relationship (Fig. 6).127 The statistical analyses of the comprehensive data reveal a dominant role of combustion (anthropogenic and pyrogenic) Fe in high Fe bioaccessibility at low concentrations in real environments.127
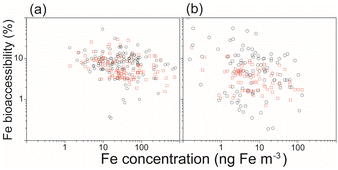 |
| Fig. 6 Relationship between the aerosol Fe concentration (ng m−3) and Fe bioaccessibility (%) for the field data (black circles) and (b) IMPACT model (red squares) over (a) the North Pacific (<66°N) and (b) the South Pacific (>60°S) regions (98° < latitude < 293.75°).32 | |
In addition to the shipboard measurements, a ground-based field study concludes that when estimated aerosol pH is lower than 2, the proton-promoted dissolution of Fe leads to a strong relationship between bioaccessible Fe and pH.128 Meanwhile, a higher bioaccessible Fe concentration under higher pH conditions in aerosol liquid water is often observed with higher oxalate concentrations.128 Laboratory experiments demonstrate that organic ligands such as oxalate enhance aerosol Fe bioaccessibility in acidic solutions (pH 2–3).129 Oxalate may play a key role in releasing Fe from the particle phase via the ligand-promoted dissolution process.130,131 Insights on the roles of the two processes (i.e., proton vs. oxalate dissolution of Fe) can be provided by laboratory experiments conducted at high ionic strengths. The results indicate that high (NH4)2SO4 concentrations in the presence of oxalate suppress the Fe dissolution rate of coal fly ash at low pH < 2.132
Contribution of bioaccessible Fe deposition from anthropogenic, pyrogenic, and lithogenic aerosols into the Pacific is shown in Fig. 7.32 Although the lithogenic source dominates in most regions, the anthropogenic source is the major contributor of bioaccessible Fe deposition in the western North Pacific. Stable Fe isotopic composition of fine aerosols can be used as fingerprints for anthropogenic sources because Fe is highly fractionated during evaporation under high-temperature conditions (>800 °C).133–135 Observations of Fe stable isotopes are therefore considered constraints on the anthropogenic Fe contribution predicted using the models.136,137 Indeed, the model reproduces well the observed stable isotope ratios of Fe over the western North Pacific (Fig. 8).137 The anthropogenic Fe contributes 30–40% of the total bioaccessible Fe flux at 158°W between 35°N and 40°N, which is within a range of estimates based on an isotope mass balance (21–59%).138 These results quantitatively confirm the accuracy of the model-predicted contribution of anthropogenic Fe in the North Pacific.
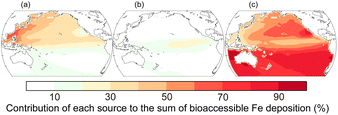 |
| Fig. 7 Contribution of atmospheric deposition of bioaccessible Fe from (a) anthropogenic (13 Gg per year Fe), (b) pyrogenic (4 Gg per year Fe), and (c) lithogenic (48 Gg per year Fe) aerosols into the Pacific in 2019.32 The values in parentheses represent the annual deposition fluxes. | |
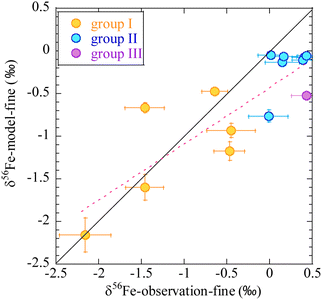 |
| Fig. 8 Comparison of observed (horizontal axis) and numerical model estimates (vertical axis) of Fe stable isotope ratios in fine particles (<2.5 μm) over the western North Pacific.137 The air masses are classified into three broad groups. The first group is mainly transported from the direction of East Asia. The second group is from the central and eastern Pacific. The third group is from the northern North Pacific. Adopted from ref. 137 under CC BY 4.0 with permission from Copernicus Publications, Copyright © The Author(s) 2021. | |
In the South Pacific, the limited amount of observational data shows sporadically high Fe bioaccessibilities in aerosols, which cannot be reproduced using the models (Fig. 6).127 Fe-containing particles co-emitted with sulfur dioxide (SO2) from metal production could substantially contribute to atmospheric bioaccessible Fe deposition in the South Pacific.32 Satellite measurements of SO2 demonstrate hotspots of SO2 emissions from smelters along the west coast of South America and the south coast of Australia.139 However, smelting Fe emission estimates remain highly uncertain.140 A few shipboard observations in the South Pacific hampered accurate estimates of bioaccessible Fe deposition to the ocean. Thus, comprehensive field observation is required to evaluate the source apportionment in the Southern Hemisphere regions near the smelting facilities and downstream of the source, simultaneously. Furthermore, different leaching techniques with different types of Fe extraction solutions have been used for aerosols sampled over the Pacific.141–145 Longer and more extensive observations and laboratory experiments based on the standardization of aerosol Fe measurement methodologies are essential to improve the observational constraints on the models.
4. Summary and outlook
This review focuses on the current state of knowledge of marine aerosols and their interactions with ocean surfaces over the Pacific Ocean. Interdisciplinary research of field observational, laboratory experimental, and numerical modelling studies is essential to foster individual studies into cross-disciplinary research. Comprehensive simultaneous observations on land, ships, and aircraft to collect data on gases, aerosols, clouds, precipitation (snowfall), and surface seawater are effective tools for assessing the emissions of aerosols from the ocean to the atmosphere and climate impacts. Utilization of experimental data obtained under controlled conditions, such as in mesocosm experiments using marine microorganisms, in models is expected to improve the accuracy of model predictions for atmospheric observational data. In order to understand the variability over longer time scales, it is also important to accumulate time-series data on aerosols and related parameters from long-term observations within the ocean-atmosphere boundary layer and to link these data to ocean surface data from satellites.
The research summarized in this review highlights the need to strategically design field campaigns to collect simultaneous data on the isotopic, multi-element, and mineral composition in aerosols, clouds, precipitation (including snowfall), and surface seawater as tools to track the origin of aerosols deposited in the sea surface layer. In this context, dissolved black carbon (DBC) in filtered seawater can be used as a tracer for the origin of anthropogenic combustion sources in seawater.146 The complex scattering amplitude measurement technique147,148 can be applied to the analysis of suspended particles in seawater because it can count solid particles suspended in water as individual particles and is expected to enable quantitative analysis of mass transfer between the atmosphere and ocean. Therefore, it is expected to work effectively as a constraint for numerical models.
Finally, the co-emissions of anthropogenic Fe and SO2 not only supply essential nutrients to the marine environment but also cause health hazards in regions near the source and its downstream. Thus, future research on anthropogenic Fe should contribute to efficient and effective control strategies for air quality management in line with marine environmental conservation. Adaptive management may be applicable to the case in the Southern Hemisphere where mineral resources have been extensively explored and marine natural resources are vital to food security. To do so, more research is needed to quantitatively assess the impact of anthropogenic Fe on marine ecosystems and fisheries. International collaborations are essential to promote the interdisciplinary research of field observations, laboratory experiments, and numerical models and to inform policymakers about ocean sustainability.
Author contributions
A. I. proposed the concept, led workshop discussions at Hokkaido University in 2021 (A. I., Y. M., F. T., Y. I., and Y. K.), and wrote the draft of the introduction and Section 3.2. Y. M. wrote the draft of Section 2.1. Y. I. wrote the draft of Section 2.2. Y. K. wrote the draft of Section 2.3. F. T. wrote the draft of Section 3.1. All authors contributed to revising and finalizing the manuscript.
Conflicts of interest
There are no conflicts of interest to declare.
Acknowledgements
The authors are grateful to Dr Jun Nishioka, Dr Takuma Miyagawa, Dr Mitsuo Uematsu, and members of the Atmospheric Chemistry Society of Japan for their valuable comments and suggestions on this manuscript. Support for this research was provided to A. I. by JSPS KAKENHI Grant Number 20H04329 and by MEXT-Program for the advanced studies of climate change projection (SENTAN) Grant Number JPMXD0722681344. The workshop at Hokkaido University was supported by the joint research program of the Institute of Low Temperature Science, Hokkaido University.
References
- P. J. Crutzen and E. F. Stoermer, The Anthropocene, IGBP Glob. Chang. Newsl., 2000, 41, 17–18 Search PubMed , http://www.igbp.net/download/18.316f18321323470177580001401/1376383088452/NL41.pdf.
- A. Ito, Y. Ye, C. Baldo and Z. Shi, Ocean fertilization by pyrogenic aerosol iron, npj Clim. Atmos. Sci., 2021, 4, 30, DOI:10.1038/s41612-021-00185-8.
- J. Schmale, P. Zieger and A. M. L. Ekman, Aerosols in current and future Arctic climate, Nat. Clim. Change, 2021, 11, 95–105, DOI:10.1038/s41558-020-00969-5.
- J. Mülmenstädt, M. Salzmann, J. E. Kay, M. D. Zelinka, P.-L. Ma, C. Nam, J. Kretzschmar, S. Hörnig and J. Quaas, An underestimated negative cloud feedback from cloud lifetime changes, Nat. Clim. Change, 2021, 11, 508–513, DOI:10.1038/s41558-021-01038-1.
- M. D. Zelinka, T. A. Myers, D. T. McCoy, S. Po-Chedley, P. M. Caldwell, P. Ceppi, S. A. Klein and K. E. Taylor, Causes of higher climate sensitivity in CMIP6 models, Geophys. Res. Lett., 2020, 47, e2019GL085782, DOI:10.1029/2019GL085782.
- R. J. Charlson, J. E. Lovelock, M. O. Andreae and S. G. Warren, Oceanic phytoplankton, atmospheric sulfur, cloud albedo and climate, Nature, 1987, 326, 655–661, DOI:10.1038/326655a0.
- T. W. Wilson, L. A. Ladino, P. A. Alpert, M. N. Breckels, I. M. Brooks, J. Browse, S. M. Burrows, K. S. Carslaw, J. A. Huffman, C. Judd, W. P. Kilthau, R. H. Mason, G. McFiggans, L. A. Miller, J. J. Nájera, E. Polishchuk, S. Rae, C. L. Schiller, M. Si, J. V. Temprado, T. F. Whale, J. P. S. Wong, O. Wurl, J. D. Yakobi-Hancock, J. P. D. Abbatt, J. Y. Aller, A. K. Bertram, D. A. Knopf and B. J. Murray, A marine biogenic source of atmospheric ice-nucleating particles, Nature, 2015, 525, 234–238, DOI:10.1038/nature14986.
- G. M. Flato, Earth system models: an overview, WIREs Clim. Change, 2011, 2, 783–800, DOI:10.1002/Wcc.148.
- M. Kawamiya, T. Hajima, K. Tachiiri, S. Watanabe and T. Yokohata, Two decades of Earth system modeling with an emphasis on Model for Interdisciplinary Research on Climate (MIROC), Prog. Earth Planet. Sci., 2020, 7, 64, DOI:10.1186/s40645-020-00369-5.
- J. H. Martin, Glacial-interglacial CO2 change: the iron hypothesis, Paleoceanography, 1990, 5, 1–13, DOI:10.1029/PA005i001p00001.
- T. D. Jickells, Z. S. An, K. K. Andersen, A. R. Baker, G. Bergametti, N. Brooks, J. J. Cao, P. W. Boyd, R. A. Duce, K. A. Hunter, H. Kawahata, N. Kubilay, J. laRoche, P. S. Liss, N. Mahowald, J. M. Prospero, A. J. Ridgwell, I. Tegen and R. Torres, Global iron connections between desert dust, ocean biogeochemistry, and climate, Science, 2005, 308, 67–71, DOI:10.1126/science.1105959.
- R. A. Duce, J. LaRoche, K. Altieri, K. R. Arrigo, A. R. Baker, D. G. Capone, S. Cornell, F. Dentener, J. Galloway, R. S. Ganeshram, R. J. Geider, T. Jickells, M. M. Kuypers, R. Langlois, P. S. Liss, S. M. Liu, J. J. Middelburg, C. M. Moore, S. Nickovic, A. Oschlies, T. Pedersen, J. Prospero, R. Schlitzer, S. Seitzinger, L. L. Sorensen, M. Uematsu, O. Ulloa, M. Voss, B. Ward and L. Zamora, Impacts of atmospheric anthropogenic nitrogen on the open ocean, Science, 2008, 320, 893–897, DOI:10.1126/science.1150369.
- T. D. Jickells, E. Buitenhuis, K. Altieri, A. R. Baker, D. Capone, R. A. Duce, F. Dentener, K. Fennel, M. Kanakidou, J. LaRoche, K. Lee, P. Liss, J. J. Middelburg, J. K. Moore, G. Okin, A. Oschlies, M. Sarin, S. Seitzinger, J. Sharples, A. Singh, P. Suntharalingam, M. Uematsu and L. M. Zamora, A reevaluation of the magnitude and impacts of anthropogenic atmospheric nitrogen inputs on the ocean, Global Biogeochem. Cycles, 2017, 31, 289–305, DOI:10.1002/2016GB005586.
- P. Quinn and T. Bates, The case against climate regulation via oceanic phytoplankton sulphur emissions, Nature, 2011, 480, 51–56, DOI:10.1038/nature10580.
- A. Krishnamurty, J. K. Moore, N. Mahowald, C. Luo, S. Doney, K. Lindsay and C. Zender, Impacts of increasing anthropogenic soluble iron and nitrogen deposition on ocean biogeochemistry, Global Biogeochem. Cycles, 2009, 23, GB3016, DOI:10.1029/2008GB003440.
- C. J. Somes, A. Landolfi, W. Koeve and A. Oschlies, Limited impact of atmospheric nitrogen deposition on marine productivity due to biogeochemical feedbacks in a global ocean model, Geophys. Res. Lett., 2016, 43, 4500–4509, DOI:10.1002/2016GL068335.
- P. W. Boyd, D. S. Mackie and K. A. Hunter, Aerosol iron deposition to the surface ocean-modes of iron supply and biological responses, Mar. Chem., 2010, 120, 128–143, DOI:10.1016/j.marchem.2009.01.008.
- T. Takahashi, S. C. Sutherland, R. Wanninkhof, C. Sweeney, R. A. Feely, D. W. Chipman, B. Hales, G. Friederich, F. Chavez, C. Sabine, A. Watson, D. C. E. Bakker, U. Schuster, N. Metzl, H. Yoshikawa-Inoue, M. Ishii, T. Midorikawa, Y. Nojiri, A. Kortzinger, T. Steinhoff, M. Hoppema, J. Olafsson, T. S. Arnarson, B. Tilbrook, T. Johannessen, A. Olsen, R. Bellerby, C. S. Wong, B. Delille, N. R. Bates and H. J. W. de Baar, Climatological mean and decadal change in surface ocean pCO2, and net sea-air CO2 flux over the global oceans, Deep Sea Res. Part I, 2009, 56, 2075–2076, DOI:10.1016/j.dsr.2009.07.007.
- J. Nishioka, H. Obata, T. Hirawake, Y. Kondo, Y. Yamashita, K. Misumi and I. Yasuda, A review: iron and nutrient supply in the subarctic Pacific and its impact on phytoplankton production, J. Oceanogr., 2021, 77, 561–587, DOI:10.1007/s10872-021-00606-5.
- A. Ito, M. M. G. Perron, B. C. Proemse, M. Strzelec, M. Gault-Ringold, P. W. Boyd and A. R. Bowie, Evaluation of aerosol iron solubility over Australian coastal regions based on inverse modeling: implications of bushfires on bioaccessible iron concentrations in the Southern Hemisphere, Prog. Earth Planet. Sci., 2020, 7, 42, DOI:10.1186/s40645-020-00357-9.
- W. Tang, J. Llort, J. Weis, M. M. G. Perron, S. Basart, Z. Li, S. Sathyendranath, T. Jackson, E. S. Rodriguez, B. C. Proemse, A. R. Bowie, C. Schallenberg, P. G. Strutton, R. Matear and N. Cassar, Widespread phytoplankton blooms triggered by 2019–2020 Australian wildfires, Nature, 2021, 597, 370–375, DOI:10.1038/s41586-021-03805-8.
- P. J. Harrison, P. W. Boyd, D. E. Varela, S. Takeda, A. Shiomoto and T. Odate, Comparison of factors controlling phytoplankton productivity in the NE and NW subarctic Pacific gyres, Prog. Oceanogr., 1999, 43, 205–234, DOI:10.1016/S0079-6611(99)00015-4.
- C. D. O'Dowd, M. C. Facchini, F. Cavalli, D. Ceburnis, M. Mircea, S. Decesari, S. Fuzzi, Y. J. Yoon and J.-P. Putaud, Biogenically driven organic contribution to marine aerosol, Nature, 2004, 431, 676–680, DOI:10.1038/nature02959.
-
Western Pacific Air–Sea Interaction Study, ed. M. Uematsu, Y. Yokouchi, T. W. Watanabe, S. Takeda and Y. Yamanaka, TERRAPUB, 2014, p. 284 Search PubMed.
-
E. C. Monahan, D. E. Spiel, and K. L. Davidson, A model of marine aerosol generation via whitecaps and wave disruption, in Oceanic Whitecaps and Their Role in Air–Sea Exchange Processes, ed. E. C. Monahan and G. MacNiocaill, Reidel, Dordrecht, Netherlands, 1986, pp. 167–174 Search PubMed.
- S. Gong, L. A. Barrie and J.-P. Blanchet, Modeling sea salt aerosols in the atmosphere. 1: model development, J. Geophys. Res., 1997, 102, 3805–3818, DOI:10.1029/96JD02953.
- G. de Leeuw, E. L. Andreas, M. D. Anguelova, C. W. Fairall, E. R. Lewis, C. O'Dowd, M. Schulz and S. E. Schwartz, Production flux of sea spray aerosol, Rev. Geophys., 2011, 49, RG2001, DOI:10.1029/2010rg000349.
- J. Ovadnevaite, A. Manders, G. de Leeuw, D. Ceburnis, C. Monahan, A.-I. Partanen, H. Korhonen and C. D. O'Dowd, A sea spray aerosol flux parameterization encapsulating wave state, Atmos. Chem. Phys., 2014, 14, 1837–1852, DOI:10.5194/acp-14-1837-2014.
- C. D. O'Dowd, B. Langmann, S. Varghese, C. Scannell, D. Ceburnis and M. C. Facchini, A combined organic–inorganic sea-spray source function, Geophys. Res. Lett., 2008, 35, L01801, DOI:10.1029/2007GL030331.
- A. Ito and M. Kawamiya, Potential impact of ocean ecosystem changes due to global warming on marine organic carbon aerosols, Global Biogeochem. Cycles, 2010, 24, GB1012, DOI:10.1029/2009GB003559.
- B. Gantt and N. Meskhidze, The physical and chemical characteristics of marine primary organic aerosol: a review, Atmos. Chem. Phys., 2013, 13, 3979–3996, DOI:10.5194/acp-13-3979-2013.
- A. Ito and T. Miyakawa, Aerosol iron from metal production as a secondary source of bioaccessible iron, Environ. Sci. Technol., 2023, 57, 4091–4100, DOI:10.1021/acs.est.2c06472.
- B. Gantt, M. S. Johnson, N. Meskhidze, J. Sciare, J. Ovadnevaite, D. Ceburnis and C. D. O'Dowd, Model evaluation of marine primary organic aerosol emission schemes, Atmos. Chem. Phys., 2012, 12, 8553–8566, DOI:10.5194/acp-12-8553-2012.
- S. Myriokefalitakis, E. Vignati, K. Tsigaridis, C. Papadimas, J. Sciare, N. Mihalopoulos, M. C. Facchini, M. Rinaldi, F. J. Dentener, D. Ceburnis, N. Hatzianastasiou, C. D. O'Dowd, M. van Weele and M. Kanakidou, Global modeling of the oceanic source of organic aerosols, Adv. Meteorol., 2010, 939171, DOI:10.1155/2010/939171.
- N. Meskhidze, J. Xu, B. Gantt, Y. Zhang, A. Nenes, S. J. Ghan, X. Liu, R. Easter and R. Zaveri, Global distribution and climate forcing of marine organic aerosol: 1. model improvements and evaluation, Atmos. Chem. Phys., 2011, 11, 11689–11705, DOI:10.5194/acp-11-11689-2011.
- P. K. Quinn, T. S. Bates, K. S. Schulz, D. J. Coffman, A. A. Frossard, L. M. Russell, W. C. Keene and D. J. Kieber, Contribution of sea surface carbon pool to organic matter enrichment in sea spray aerosol, Nat. Geosci., 2014, 7, 228–232, DOI:10.1038/ngeo2092.
- Y. Miyazaki, Y. Yamashita, K. Kawana, E. Tachibana, S. Kagami, M. Mochida, K. Suzuki and J. Nishioka, Chemical transfer of dissolved organic matter from surface seawater to sea spray water-soluble organic aerosol in the marine atmosphere, Sci. Rep., 2018, 8, 14861, DOI:10.1038/s41598-018-32864-7.
- Y. Miyazaki, K. Suzuki, E. Tachibana, Y. Yamashita, A. Müller, K. Kawana and J. Nishioka, New index of organic mass enrichment in sea spray aerosols linked with senescent status in marine phytoplankton, Sci. Rep., 2020, 10, 17042, DOI:10.1038/s41598-020-73718-5.
- K. Kawana, K. Matsumoto, F. Taketani, T. Miyakawa and Y. Kanaya, Fluorescent biological aerosol particles over the central Pacific Ocean: covariation with ocean-surface biological activity indicators, Atmos. Chem. Phys., 2021, 21, 15969–15983, DOI:10.5194/acp-21-15969-2021.
- W. Hu, K. Murata, S. Fukuyama, Y. Kawai, E. Oka, M. Uematsu and D. Zhang, Concentration and viability of airborne bacteria over the Kuroshio extension region in the northwestern Pacific Ocean: data from three cruises, J. Geophys. Res.: Atmos., 2017, 122, 12892–12905, DOI:10.1002/2017JD027287.
- M. Yoshizue, Y. Iwamoto, K. Adachi, S. Kato, S. Sun, K. Miura and M. Uematsu, Individual particle analysis of marine aerosols collected during the north–south transect cruise in the Pacific Ocean and its marginal seas, J. Oceanogr., 2019, 75, 513–524, DOI:10.1007/s10872-019-00519-4.
- S. C. Hackenberg, S. J. Andrews, R. Airs, S. R. Arnold, H. A. Bouman, R. J. W. Brewin, R. J. Chance, D. Cummings, G. Dall'Olmo, A. C. Lewis, J. K. Minaeian, K. M. Reifel, A. Small, G. A. Tarran, G. H. Tilstone and L. J. Carpenter, Potential controls of isoprene in the surface ocean, Global Biogeochem. Cycles, 2017, 31, 644–662, DOI:10.1002/2016GB005531.
- A. Lana, T. G. Bell, R. Simó, S. M. Vallina, J. Ballabrera-Poy, A. J. Kettle, J. Dachs, L. Bopp, E. S. Saltzman, J. Stefels, J. E. Johnson and P. S. Liss, An updated climatology of surface dimethlysulfide concentrations and emission fluxes in the global ocean, Global Biogeochem. Cycles, 2011, 25, 1–17, DOI:10.1029/2010GB003850.
- P. S. Liss and P. G. Slater, Flux of gases across the air–sea interface, Nature, 1974, 247, 181–184, DOI:10.1038/247181a0.
- M. T. Johnson, A numerical scheme to calculate temperature and salinity dependent air–water transfer velocities for any gas, Ocean Sci., 2010, 6, 913–932, DOI:10.5194/os-6-913-2010.
- S. Myriokefalitakis, M. Vrekoussis, K. Tsigaridis, F. Wittrock, N. A. Richter, C. Brühl, R. Volkamer, J. P. Burrows and M. Kanakidou, The influence of natural and anthropogenic secondary sources on the glyoxal global distribution, Atmos. Chem. Phys., 2008, 8, 4965–4981, DOI:10.5194/acp-8-4965-2008.
- A. Ito, S. Sillman and J. E. Penner, Effects of additional nonmethane volatile organic compounds, organic nitrates, and direct emissions of oxygenated organic species on global tropospheric chemistry, J. Geophys. Res., 2007, 112, D06309, DOI:10.1029/2005JD006556.
- B. Ervens and R. Volkamer, Glyoxal processing by aerosol multiphase chemistry: towards a kinetic modeling framework of secondary organic aerosol formation in aqueous
particles, Atmos. Chem. Phys., 2010, 10, 8219e8244, DOI:10.5194/acp-10-8219-2010.
- G. Lin, S. Sillman, J. E. Penner and A. Ito, Global modeling of SOA: the use of different mechanisms for aqueous phase formation, Atmos. Chem. Phys., 2014, 14, 5451e5475, DOI:10.5194/acp-14-5451-2014.
- A. Ito, G. Lin and J. E. Penner, Global modeling study of soluble organic nitrogen from open biomass burning, Atmos. Environ., 2015, 121, 103–112, DOI:10.1016/j.atmosenv.2015.01.031.
- T. M. Fu, D. J. Jacob, F. Wittrock, J. P. Burrows, M. Vrekoussis and D. K. Henze, Global budgets of atmospheric glyoxal and methylglyoxal, and implications for formation of secondary organic aerosols, J. Geophys. Res., 2008, 113, D15303, DOI:10.1029/2007jd009505.
- H. Tanimoto, S. Kameyama, T. Iwata, S. Inomata and Y. Omori, Measurement of air–sea exchange of dimethyl sulfide and acetone by PTR-MS coupled with gradient flux technique, Environ. Sci. Technol., 2014, 48(1), 526–533, DOI:10.1021/es4032562.
- A. B. Thames, W. H. Brune, D. O. Miller, H. M. Allen, E. C. Apel, D. R. Blake, T. P. Bui, R. Commane, J. D. Crounse, B. C. Daube, G. S. Diskin, J. P. DiGangi, J. W. Elkins, S. R. Hall, T. F. Hanisco, R. A. Hannun, E. Hintsa, R. S. Hornbrook, M. J. Kim, K. McKain, F. L. Moore, J. M. Nicely, J. Peischl, T. B. Ryerson, J. M. St. Clair, C. Sweeney, A. Teng, C. R. Thompson, K. Ullmann, P. O. Wennberg and G. M. Wolfe, Missing OH reactivity in the global marine boundary layer, Atmos. Chem. Phys., 2020, 20, 4013–4029, DOI:10.5194/acp-20-4013-2020.
- T. Dobashi, Y. Miyazaki, E. Tachibana, K. Takahashi, S. Horii, F. Hashihama, S. Yasui-Tamura, Y. Iwamoto, S.-K. Wong and K. Hamasaki, Marine nitrogen fixation as a possible source of atmospheric water-soluble organic nitrogen aerosols in the subtropical North Pacific, Biogeosciences, 2023, 20, 439–449 CrossRef.
- K. E. Altieri, S. E. Fawcett and M. G. Hastings, Reactive nitrogen cycling in the atmosphere and ocean, Annu. Rev. Earth Planet. Sci., 2021, 49, 523–550, DOI:10.1146/annurev-earth-083120-052147.
- M. Brüggemann, N. Hayeck and C. George, Interfacial Photochemistry at the ocean surface is a global source of organic vapors and aerosols, Nat. Commun., 2018, 9, 2101, DOI:10.1038/s41467-018-04528-7.
- D. S. Covert, V. N. Kapustin, T. S. Bates and P. K. Quinn, Physical properties of marine boundary layer aerosol particles of the mid-pacific in relation to sources and meteorological transport, J. Geophys. Res., 1996, 101, 6919–6930, DOI:10.1029/95JD03068.
- K. A. Prather, T. H. Bertram, V. H. Grassian, G. B. Deane, M. D. Stokes, P. J. DeMott, L. I. Aluwihare, B. P. Palenik, F. Azam, J. H. Seinfeld, R. C. Moffet, M. J. Molina, C. D. Cappa, F. M. Geiger, G. C. Roberts, L. M. Russell, A. P. Ault, J. Baltrusaitisc, D. B. Collins, C. E. Corrigan, L. A. Cuadra-Rodriguez, C. J. Ebben, S. D. Forestieri, T. L. Guasco, S. P. Hersey, M. J. Kim, W. F. Lambert, R. L. Modini, W. Mui, B. E. Pedler, M. J. Ruppel, O. S. Ryder, N. G. Schoepp, R. C. Sullivan and D. Zhao, Bringing the ocean into the laboratory to probe the chemical complexity of sea spray aerosol, Proc. Natl. Acad. Sci. U.S.A., 2013, 110, 7550–7555, DOI:10.1073/pnas.1300262110.
- J. K. Mayer, X. Wang, M. V. Santander, B. A. Mitts, J. Sauer, C. M. Sultana, C. D. Cappa and K. A. Prather, Secondary marine aerosol plays a dominant role over primary sea spray aerosol in cloud formation, ACS Cent. Sci., 2020, 6, 2259–2266, DOI:10.1021/acscentsci.0c00793.
- T. Berndt, W. Scholz, B. Mentler, L. Fischer, H. Herrmann, M. Kulmala and A. Hansel, Accretion product formation from self- and cross-reactions of RO2 radicals in the atmosphere, Angew. Chem. Int. Ed., 2018, 57, 3820–3824, DOI:10.1002/anie.201710989.
- P. R. Veres, J. A. Neuman, T. H. Bertram, E. Assaf, G. M. Wolfe, C. J. Williamson, B. Weinzierl, S. Tilmes, C. R. Thompson, A. B. Thames, J. C. Schroder, A. Saiz-Lopez, A. W. Rollins, J. M. Roberts, D. Price, J. Peischl, B. A. Nault, K. H. Møller, D. O. Miller, S. Meinardi, Q. Li, J. F. Lamarque, A. Kupc, H. G. Kjaergaard, D. Kinnison, J. L. Jimenez, C. M. Jernigan, R. S. Hornbrook, A. Hills, M. Dollner, D. A. Day, C. A. Cuevas, P. Campuzano-Jost, J. Burkholder, T. Paul Bui, W. H. Brune, S. S. Brown, C. A. Brock, I. Bourgeois, D. R. Blake, E. C. Apel and T. B. Ryerson, Global airborne sampling reveals a previously unobserved dimethyl sulfide oxidation mechanism in the marine atmosphere, Proc. Natl. Acad. Sci. U.S.A., 2020, 117, 4505–4510, DOI:10.1073/pnas.1919344117.
- G. A. Novak, C. H. Fite, C. D. Holmes, P. R. Veres, J. A. Neuman, I. Faloona, J. A. Thornton, G. M. Wolfe, M. P. Vermeuel, C. M. Jernigan, J. Peischl, T. B. Ryerson, C. R. Thompson, I. Bourgeois, C. Warneke, G. I. Gkatzelis, M. M. Coggon, K. Sekimoto, T. P. Bui, J. Dean-Day, G. S. Diskin, J. P. DiGangi, J. B. Nowak, R. H. Moore, E. B. Wiggins, E. L. Winstead, C. Robinson, K. L. Thornhill, K. J. Sanchez, S. R. Hall, K. Ullmann, M. Dollner, B. Weinzierl, D. R. Blake and T. H. Bertram, Rapid cloud removal of dimethyl sulfide oxidation products limits SO2 and cloud condensation nuclei production in the marine atmosphere, Proc. Natl. Acad. Sci. U.S.A., 2021, 118, e2110472118, DOI:10.1073/pnas.2110472118.
- K. M. Fung, C. L. Heald, J. H. Kroll, S. Wang, D. S. Jo, A. Gettelman, Z. Lu, X. Liu, R. A. Zaveri, E. C. Apel, D. R. Blake, J.-L. Jimenez, P. Campuzano-Jost, P. R. Veres, T. S. Bates, J. E. Shilling and M. Zawadowicz, Exploring dimethyl sulfide (DMS) oxidation and implications for global aerosol radiative forcing, Atmos. Chem. Phys., 2022, 22, 1549–1573, DOI:10.5194/acp-22-1549-2022.
- J. Bock, M. Michou, P. Nabat, M. Abe, J. P. Mulcahy, D. J. L. Olivié, J. Schwinger, P. Suntharalingam, J. Tjiputra, M. van Hulten, M. Watanabe, A. Yool and R. Séférian, Evaluation of ocean dimethylsulfide concentration and emission in CMIP6 models, Biogeosciences, 2018, 18, 3823–3860, DOI:10.5194/bg-18-3823-2021.
- C. J. Williamson, A. Kupc, A. Axisa, R. Kelsey, K. R. Bilsback, T. P. Bui, P. Campuzano-Jost, M. Dollner, K. D. Froyd, A. L. Hodshire, J. L. Jimenez, J. K. Kodros, G. Luo, D. M. Murphy, B. A. Nault, E. A. Ray, B. Weinzierl, J. C. Wilson, F. Yu, P. Yu, J. R. Pierce and C. A. Brock, A large source of cloud condensation nuclei from new particle formation in the tropics, Nature, 2019, 574, 399–403, DOI:10.1038/s41586-019-1638-9.
- D. B. Collins, T. H. Bertram, C. M. Sultana, C. Lee, J. L. Axson and K. A. Prather, Phytoplankton blooms weakly influence the cloud forming ability of sea spray aerosol, Geophys. Res. Lett., 2016, 43, 9975–9983, DOI:10.1002/2016GL069922.
- P. A. Alpert, W. P. Kilthau, D. W. Bothe, J. C. Radway, J. Y. Aller and D. A. Knopf, The influence of marine microbial activities on aerosol production: a laboratory mesocosm study, J. Geophys. Res.: Atmos., 2015, 120, 8841–8860, DOI:10.1002/2015JD023469.
- W. Xu, J. Ovadnevaite, K. N. Fossum, C. Lin, R.-J. Huang, D. Ceburnis and C. O'Dowd, Sea spray as an obscured source for marine cloud nuclei, Nat. Geosci., 2022, 15, 282–286, DOI:10.1038/s41561-022-00917-2.
- T. Takemura, T. Nakajima, A. Higurashi, S. Ohta and N. Sugimoto, Aerosol distributions and radiative forcing over the Asian Pacific region simulated by Spectral Radiation-Transport Model for Aerosol Species (SPRINTARS), J. Geophys. Res., 2003, 108(D23), 8659, DOI:10.1029/2002JD003210.
- K. Sellegri, A. Nicosia and E. Freney, Surface ocean microbiota determine cloud precursors, Sci. Rep., 2021, 11, 281, DOI:10.1038/s41598-020-78097-5.
- H. Kawai, S. Yukimoto, T. Koshiro, N. Oshima, T. Tanaka, H. Yoshimura and R. Nagasawa, Significant improvement of cloud representation in the global climate model MRI-ESM2, Geosci. Model Dev., 2019, 12, 2875–2897, DOI:10.5194/gmd-12-2875-2019.
- S. M. Burrows, W. Elbert, M. G. Lawrence and U. Pöschl, Bacteria in the global atmosphere – part 1: review and synthesis of literature data for different ecosystems, Atmos. Chem. Phys., 2009, 9, 9263–9280, DOI:10.5194/acp-9-9263-2009.
- D. A. Knopf, B. Wang, A. Laskin, R. C. Moffet and M. K. Gilles, Stimulation of ice nucleation by marine diatoms, Nat. Geosci., 2011, 4, 88–90, DOI:10.1038/ngeo1037.
- J. Rosinski, P. L. Haagenson, C. T. Nagamoto and F. Parungo, Nature of ice-forming nuclei in marine air masses, J. Aerosol Sci., 1987, 18, 291–309, DOI:10.1016/0021-8502(87)90024-3.
- P. J. DeMott, T. C. Hill, C. S. McCluskey, K. A. Prather, D. B. Collins, R. C. Sullivan, M. J. Ruppel, R. H. Mason, V. E. Irish, T. Lee, C. Y. Hwang, T. S. Rhee, J. R. Snider, G. R. McMeeking, S. Dhaniyala, E. R. Lewis, J. J. Wentzell, J. Abbatt, C. Lee, C. M. Sultana, A. P. Ault, J. L. Axson, M. Diaz Martinez, I. Venero, G. Santos-Figueroa, M. D. Stokes, G. B. Deane, O. L. Mayol-Bracero, V. H. Grassian, T. H. Bertram, A. K. Bertram, B. F. Moffett and G. D. Franc, Sea spray aerosol as a unique source of ice nucleating particles, Proc. Natl. Acad. Sci. U. S. A., 2016, 113, 5797–5803, DOI:10.1073/pnas.1514034112.
- B. A. Mitts, X. Wang, D. D. Lucero, C. M. Beall, G. B. Deane, P. J. DeMott and K. A. Prather, Importance of supermicron ice nucleating particles in nascent sea spray, Geophys. Res. Lett., 2021, 48(3), e2020GL089633, DOI:10.1029/2020gl089633.
- C. S. McCluskey, T. C. J. Hill, F. Malfatti, C. M. Sultana, C. Lee, M. V. Santander, C. M. Beall, K. A. Moore, G. C. Cornwell, D. B. Collins, K. A. Prather, T. Jayarathne, E. A. Stone, F. Azam, S. M. Kreidenweis and P. J. DeMott, A dynamic link between ice nucleating particles released in nascent sea spray aerosol and oceanic biological activity during two mesocosm experiments, J. Atmos. Sci., 2017, 74, 151–166, DOI:10.1175/jas-d-16-0087.1.
- J. Vergara-Temprado, A. K. Miltenberger, K. Furtado, D. P. Grosvenor, B. J. Shipway, A. A. Hill, J. M. Wilkinson, P. R. Field, B. J. Murray and K. S. Carslaw, Strong control of Southern Ocean cloud reflectivity by ice-nucleating particles, Proc. Natl. Acad. Sci. U.S.A., 2018, 115, 2687–2692, DOI:10.1073/pnas.1721627115.
- C. S. McCluskey, T. C. J. Hill, R. S. Humphries, A. M. Rauker, S. Moreau, P. G. Strutton, S. D. Chambers, A. G. Williams, I. McRobert, J. Ward, M. D. Keywood, J. Harnwell, W. Ponsonby, Z. M. Loh, P. B. Krummel, A. Protat, S. M. Kreidenweis and P. J. DeMott, Observations of Ice Nucleating Particles Over Southern Ocean Waters, Geophys. Res. Lett., 2018, 45, 911989–911997, DOI:10.1029/2018GL079981.
- C. S. McCluskey, P. J. DeMott, P. -L. Ma and S. M. Burrows, Numerical representations of marine ice-nucleating particles in remote marine environments evaluated against observations, Geophys. Res. Lett., 2019, 46, 7838–7847, DOI:10.1029/2018GL081861.
- X. Zhao, X. Liu, S. M. Burrows and Y. Shi, Effects of marine organic aerosols as sources of immersion-mode ice-nucleating particles on high-latitude mixed-phase clouds, Atmos. Chem. Phys., 2021, 21, 2305–2327, DOI:10.5194/acp-21-2305-2021.
- A. A. Adebiyi, J. F. Kok, B. J. Murray, C. L. Ryder, J.-B. W. Stuut, R. A. Kahn, P. Knippertz, P. Formenti, N. M. Mahowald, C. Pérez García-Pando, M. Klose, A. Ansmann, B. H. Samset, A. Ito, Y. Balkanski, C. Di Biagio, M. N. Romanias, Y. Huang and J. Meng, A review of coarse mineral dust in the Earth system, Aeolian Res., 2023, 60, 100849, DOI:10.31223/X5QD36.
- K. Kawana, F. Taketani, K. Matsumoto, T. Miyakawa, Y. Tobo, Y. Iwamoto, A. Ito and Y. Kanaya, Roles of marine biota in the formation of atmospheric bioaerosols, cloud condensation nuclei, and ice-nucleating particles over the North Pacific Ocean, Bering Sea, and Arctic Ocean, Atmos. Chem. Phys. Discuss., 2023 DOI:10.5194/acp-2023-39.
- P. J. DeMott, T. C. Hill, C. S. McCluskey, K. A. Prather, D. B. Collins, R. C. Sullivan, M. J. Ruppel, R. H. Mason, V. E. Irish, T. Lee, C. Y. Hwang, T. S. Rhee, J. R. Snider, G. R. McMeeking, S. Dhaniyala, E. R. Lewis, J. J. B. Wentzell, J. Abbatt, C. Lee, C. M. Sultana, A. P. Ault, J. L. Axson, M. D. Martinez, I. Venero, G. Santos-Figueroa, M. D. Stokes, G. B. Deane, O. L. Mayol-Bracero, V. H. Grassian, T. H. Bertram, A. K. Bertram, B. F. Moffett and G. D. Franc, Sea spray aerosol as a unique source of ice nucleating particles, Proc. Natl. Acad. Sci. U.S.A., 2016, 113, 5797–5803, DOI:10.1073/pnas.1514034112.
- G. M. McFarquhar, C. Bretherton, R. Marchand, A. Protat, P. J. DeMott, S. P. Alexander, G. C. Roberts, C. H. Twohy, D. Toohey, S. Siems, Y. Huang, R. Wood, R. M. Rauber, S. Lasher-Trapp, J. Jensen, J. Stith, J. Mace, J. Um, E. Järvinen, M. Schnaiter, A. Gettelman, K. J. Sanchez, C. S. McCluskey, L. M. Russell, I. L. McCoy, R. Atlas, C. G. Bardeen, K. A. Moore, T. C. J. Hill, R. S. Humphries, M. D. Keywood, Z. Ristovski, L. Cravigan, R. Schofield, C. Fairall, M. D. Mallet, S. M. Kreidenweis, B. Rainwater, J. D'Alessandro, Y. Wang, W. Wu, G. Saliba, E. J. T. Levin, S. Ding, F. Lang, S. C. H. Truong, C. Wolff, J. Haggerty, M. J. Harvey, A. Klekociuk and A. McDonald, Observations of Clouds, Aerosols, Precipitation, and Surface Radiation over the Southern Ocean: An Overview of Capricorn, Marcus, Micre and Socrates, Bull. Am. Meteorol. Soc., 2020, 1, 1–92, DOI:10.1175/BAMS-D-20-0132.1.
- E. K. Bigg, Ice nucleus concentrations in remote areas, J. Atmos. Sci., 1973, 30, 1153–1157, DOI:10.1175/1520-0469(1973)030<1153:INCIRA>2.0.CO;2.
- C. S. McCluskey, T. C. J. Hill, R. S. Humphries, A. M. Rauker, S. Moreau, P. G. Strutton, S. D. Chambers, A. G. Williams, I. McRobert, J. Ward, M. D. Keywood, J. Harnwell, W. Ponsonby, Z. M. Loh, P. B. Krummel, A. Protat, S. M. Kreidenweis and P. J. DeMott, Observations of ice nucleating particles over Southern Ocean waters, Geophys. Res. Lett., 2018, 45, 11989–11997, DOI:10.1029/2018GL079981.
- J. Schmale, A. Baccarini, I. Thurnherr, S. Henning, A. Efraim, L. Regayre, C. Bolas, M. Hartmann, A. Welti, K. Lehtipalo, F. Aemisegger, C. Tatzelt, S. Landwehr, R. L. Modini, F. Tummon, J. Johnson, N. Harris, M. Schnaiter, A. Toffoli, M. Derkani, N. Bukowiecki, F. Stratmann, J. Dommen, U. Baltensperger, H. Wernli, D. Rosenfeld, M. Gysel-Beer and K. Carslaw, Overview of the Antarctic Circumnavigation Expedition: Study of Preindustrial-like Aerosols and Their Climate Effects (ACE-SPACE), Bull. Am. Meteorol. Soc., 2019, 100, 2260–2283, DOI:10.1175/BAMS-D-18-0187.1.
- T. Miyakawa, F. Taketani, Y. Tobo, K. Matsumoto, M. Yoshizue, M. Takigawa and Y. Kanaya, Measurements of Aerosol Particles Over the Southern Ocean in the Late Austral Summer of 2017 on board the R/V Mirai: importance of the marine boundary layer structure, Earth Space Sci., 2023, 10, e2022EA00, DOI:10.1029/2022EA002736.
- A. Welti, E. K. Bigg, P. J. DeMott, X. Gong, M. Hartmann, M. Harvey, S. Henning, P. Herenz, T. C. J. Hill, B. Hornblow, C. Leck, M. Löffler, C. S. McCluskey, A. M. Rauker, J. Schmale, C. Tatzelt, M. van Pinxteren and F. Stratmann, Ship-based measurements of ice nuclei concentrations over the Arctic, Atlantic, Pacific and Southern oceans, Atmos. Chem. Phys., 2020, 20, 15191–15206, DOI:10.5194/acp-20-15191-2020.
- J. Uetake, T. C. Hill, K. A. Moore, P. J. DeMott, A. Protat and S. M. Kreidenweis, Airborne bacteria confirm the pristine nature of the Southern Ocean boundary layer, Proc. Natl. Acad. Sci. U.S.A., 2020, 117, 13275–13282, DOI:10.1073/pnas.2000134117.
- J. M. Creamean, J. N. Cross, R. Pickart, L. McRaven, P. Lin, A. Pacini, R. Hanlon, D. G. Schmale, J. Ceniceros, T. Aydell, N. Colombi, E. Bolger and P. J. DeMott, Ice nucleating particles carried from below a phytoplankton bloom to the Arctic atmosphere, Geophys. Res. Lett., 2019, 46, 8572–8581, DOI:10.1029/2019GL083039.
- Z. A. Kanji, L. A. Ladino, H. Wex, Y. Boose, M. Burkert-Kohn, D. J. Cziczo and M. Krämer, Chapter 1: overview of ice nucleating particles, Meteorol. Monogr., 2017, 58, 1–33, DOI:10.1175/AMSMONOGRAPHS-D-16-0006.1.
- R. A. Moore, C. Bomar, L. N. Kobziar and B. C. Christner, Wildland fire as an atmospheric source of viable microbial aerosols and biological ice nucleating particles, ISME J., 2021, 15, 461–472, DOI:10.1038/s41396-020-00788-8.
- L. G. Jahn, M. J. Polen, L. G. Jahl, T. A. Brubaker, J. Somers and R. C. Sullivan, Biomass combustion produces ice-active minerals in biomass-burning aerosol and bottom ash, Proc. Natl. Acad. Sci. U.S.A., 2020, 117, 21928–21937, DOI:10.1073/pnas.1922128117.
-
K. Hamasaki, Marine Microbes, Japanese Marine Life, Springer, Singapore, 2020, 341–346, DOI: DOI:10.1007/978-981-15-1326-8_31.
- I. Steinke, P. J. DeMott, G. B. Deane, T. C. J. Hill, M. Maltrud, A. Raman and S. M. Burrows, A numerical framework for simulating the atmospheric variability of supermicron marine biogenic ice nucleating particles, Atmos. Chem. Phys., 2022, 22, 847–859, DOI:10.5194/acp-22-847-2022.
- J. Martin, R. Gordon and S. Fitzwater, Iron in Antarctic waters, Nature, 1990, 345, 156–158, DOI:10.1038/345156a0.
- S. Itahashi, H. Hayami, I. Uno, X. Pan and M. Uematsu, Importance of coarse-mode nitrate produced via sea salt as atmospheric input to East Asian Oceans, Geophys. Res. Lett., 2016, 43, 5483–5491, DOI:10.1002/2016GL068722.
- R. Vet, R. S. Artz, S. Carou, M. Shaw, C.-U. Ro, W. Aas, A. Baker, V. C. Bowersox, F. Dentener, C. Galy-Lacaux, A. Hou, J. J. Pienaar, R. Gillett, M. C. Forti, S. Gromov, H. Hara, T. Khodzher, N. M. Mahowald, S. Nickovic, P. S. P. Rao and N. W. Reid, A global assessment of precipitation chemistry and deposition of sulfur, nitrogen, sea salt, base cations, organic acids, acidity and pH, and phosphorus, Atmos. Environ., 2014, 93, 3–100, DOI:10.1016/j.atmosenv.2013.10.060.
- F. Taketani, M. N. Aita, K. Yamaji, T. Sekiya, K. Ikeda, K. Sasaoka, T. Hashioka, M. C. Honda, K. Matsumoto and Y. Kanaya, Seasonal response of north western Pacific marine ecosystems to deposition of atmospheric inorganic nitrogen compounds from East Asia, Sci. Rep., 2018, 8, 9324, DOI:10.1038/s41598-018-27523-w.
- C. Zhang, A. Ito, Z. Shi, M. N. Aita, X. Yao, Q. Chu, J. Shi, X. Gong and H. Gao, Fertilization of the Northwest Pacific Ocean by East Asia air pollutants, Global Biogeochem. Cycles, 2019, 33, 690–702, DOI:10.1029/2018GB006146.
- P. Xiu and F. Chai, Impact of atmospheric deposition on carbon export to the deep ocean in the subtropical Northwest Pacific, Geophys. Res. Lett., 2021, 48, e2020GL089640, DOI:10.1029/2020GL089640.
- T. Ito, A. Nenes, M. Johnson, N. Meskhidze and C. Deutsch, Acceleration of oxygen decline in the tropical Pacific over the past decades by aerosol pollutants, Nat. Geosci., 2016, 9, 443–447, DOI:10.1038/ngeo2717.
- A. Yamamoto, T. Hajima, D. Yamazaki, M. N. Aita, A. Ito and M. Kawamiya, Competing and accelerating effects of anthropogenic nutrient inputs on climate-driven changes in ocean carbon and oxygen cycles, Sci. Adv., 2022, 8, eabl9207, DOI:10.1126/sciadv.abd.
- A. R. Baker, M. Kanakidou, K. E. Altieri, N. Daskalakis, G. S. Okin, S. Myriokefalitakis, F. Dentener, M. Uematsu, M. M. Sarin, R. A. Duce, J. N. Galloway, W. C. Keene, A. Singh, L. Zamora, J.-F. Lamarque, S.-C. Hsu, S. S. Rohekar and J. M. Prospero, Observation- and model-based estimates of particulate dry nitrogen deposition to the oceans, Atmos. Chem. Phys., 2017, 17, 8189–8210, DOI:10.5194/acp-17-8189-2017.
- H. Guo, J. Liu, K. D. Froyd, J. M. Roberts, P. R. Veres, P. L. Hayes, J. L. Jimenez, A. Nenes and R. J. Weber, Fine particle pH and gas – particle phase partitioning of inorganic species in Pasadena, California, during the 2010 CalNex campaign, Atmos. Chem. Phys., 2017, 17, 5703–5719, DOI:10.5194/acp-17-5703-2017.
- J. Kurokawa and T. Ohara, Long-term historical trends in air pollutant emissions in Asia: Regional Emission inventory in ASia (REAS) version 3, Atmos. Chem. Phys., 2020, 20, 12761–12793, DOI:10.5194/acp-20-12761-2020.
- J. Ding, K. Miyazaki, R. J. van der A, B. Mijling, J.-I. Kurokawa, S. Cho, G. Janssens-Maenhout, Q. Zhang, F. Liu and P. F. Levelt, Intercomparison of NOx emission inventories over East Asia, Atmos. Chem. Phys., 2017, 17, 10125–10141, DOI:10.5194/acp-17-10125-2017.
- A. R. Baker, M. Kanakidou, A. Nenes, S. Myriokefalitakis, P. L. Croot, R. A. Duce, Y. Gao, C. Guieu, A. Ito, T. D. Jickells, N. M. Mahowald, R. Middag, M. M. G. Perron, M. M. Sarin, R. Shelley and D. R. Turner, Changing atmospheric acidity as a modulator of nutrient deposition and ocean biogeochemistry, Sci. Adv., 2021, 7, eabd8800, DOI:10.1126/sciadv.abd8800.
- A. Ito, G. Lin and J. E. Penner, Reconciling modeled and observed atmospheric deposition of soluble organic nitrogen at coastal locations, Global Biogeochem. Cycles, 2014, 28, 617–630, DOI:10.1002/2013GB004721.
- S. Myriokefalitakis, M. Gröger, J. Hieronymus and R. Döscher, An explicit estimate of the atmospheric nutrient impact on global oceanic productivity, Ocean Sci., 2020, 16, 1183–1205, DOI:10.5194/os-16-1183-2020.
-
GEOTRACES Planning Group, GEOTRACES Science Plan, Baltimore, Maryland, Scientific Committee on Oceanic Research, 2006, https://geotracesold.sedoo.fr/libraries/documents/Science_plan.pdf Search PubMed.
- K. Misumi, J. Nishioka, H. Obata, D. Tsumune, T. Tsubono, M. C. Long, K. Lindsay and J. K. Moor, Slowly sinking particles underlie dissolved iron transport across the Pacific Ocean, Global Biogeochem. Cycles, 2021, 35, e2020GB006823, DOI:10.1029/2020GB006823.
- N. Meskhidze, C. Volker, H. A. Al-Abadleh, K. Barbeau, M. Bressac, C. Buck, R. M. Bundy, P. Croot, Y. Feng, A. Ito, A. M. Johansen, W. M. Landing, J. Q. Mao, S. Myriokefalitakis, D. Ohnemus, B. Pasquier and Y. Ye, Perspective on identifying and characterizing the processes controlling iron speciation and residence time at the atmosphere-ocean interface, Mar. Chem., 2019, 217, 103704, DOI:10.1016/j.marchem.2019.103704.
- J. M. Lauderdale, R. Braakmann, G. Forget, S. Dutkiewicz and M. J. Follows, Microbial feedbacks optimize ocean iron availability, Proc. Natl. Acad. Sci. U.S.A., 2020, 117, 4842–4849, DOI:10.1073/pnas.1917277117.
- C. Völker and Y. Ye, Feedbacks between ocean productivity and organic iron complexation in reaction to changes in ocean iron supply, Front. Mar. Sci., 2022, 9, 777334, DOI:10.3389/fmars.2022.777334.
- A. Ito, Y. Ye, A. Yamamoto, M. Watanabe and M. N. Aita, Responses of ocean biogeochemistry to atmospheric supply of lithogenic and pyrogenic iron-containing aerosols, Geol. Mag., 2020a, 157, 741–756, DOI:10.1017/S0016756819001080.
- D. S. Hamilton, M. M. G. Perron, T. C. Bond, A. R. Bowie, R. R. Buchholz, C. Guieu, A. Ito, W. Maenhaut, S. Myriokefalitakis, N. Olgun, S. D. Rathod, K. Schepanski, A. Tagliabue, R. Wagner and N. M. Mahowald, Earth, Wind, Fire, and Pollution: aerosol nutrient sources and impacts on ocean biogeochemistry, Annu. Rev. Mar. Science, 2022, 14(1), 303–330, DOI:10.1146/annurev-marine-031921-013612.
- T. J. Browning, E. P. Achterberg, A. Engel and E. Mawji, Manganese co-limitation of phytoplankton growth and major nutrient drawdown in the Southern Ocean, Nat. Commun., 2021, 12, 884, DOI:10.1038/s41467-021-21122-6.
- S. Duggen, P. Croot, U. Schacht and L. Hoffmann, Subduction zone volcanic ash can fertilize the surface ocean and stimulate phytoplankton growth: evidence from biogeochemical experiments and satellite data, Geophys. Res. Lett., 2007, 34, L01612, DOI:10.1029/2006GL027522.
- R. C. Hamme, P. W. Webley, W. R. Crawford, F. A. Whitney, M. D. DeGrandpre, S. R. Emerson, C. C. Eriksen, K. E. Giesbrecht, J. F. R. Gower, M. T. Kavanaugh, M. A. Peña, C. L. Sabine, S. D. Batten, L. A. Coogan, D. S. Grundle and D. Lockwood, Volcanic ash fuels anomalous plankton bloom in subarctic Northeast Pacific, Geophys. Res. Lett., 2010, 37, L19604, DOI:10.1029/2010GL044629.
- J. Weis, C. Schallenberg, Z. Chase, A. R. Bowie, B. Wojtasiewicz, M. M. G. Perron, M. D. Mallet and P. G. Strutton, Southern Ocean phytoplankton stimulated by wildfire emissions and sustained by iron recycling, Geophys. Res. Lett., 2022, 49, e2021GL097538, DOI:10.1029/2021GL097538.
- A. R. Baker and T. D. Jickells, Mineral particle size as a control on aerosol iron solubility, Geophys. Res. Lett., 2006, 33, L17608, DOI:10.1029/2006GL026557.
- E. R. Sholkovitz, P. N. Sedwick, T. M. Church, A. R. Baker and C. F. Powell, Fractional solubility of aerosol iron: synthesis of a global-scale data set, Geochim. Cosmochim. Acta, 2012, 89, 173–189, DOI:10.1016/j.gca.2012.04.022.
- N. M. Mahowald, D. S. Hamilton, K. R. M. Mackey, J. K. Moore, A. R. Baker, R. A. Scanza and Y. Zhang, Aerosol trace metal leaching and impacts on marine microorganisms, Nat. Commun., 2018, 9, 2614, DOI:10.1038/s41467-018-04970-7.
- A. Ito, S. Myriokefalitakis, M. Kanakidou, N. M. Mahowald, R. A. Scanza, D. S. Hamilton, A. R. Baker, T. Jickells, M. Sarin, S. Bikkina, Y. Gao, R. U. Shelley, C. S. Buck, W. M. Landing, A. R. Bowie, M. M. G. Perron, C. Guieu, N. Meskhidze, M. S. Johnson, Y. Feng, J. F. Kok, A. Nenes and R. A. Duce, Pyrogenic iron: the missing link to high iron solubility in aerosols, Sci. Adv., 2019, 5, eaau7671, DOI:10.1126/sciadv.aau7671.
- Y. Tao and J. G. Murphy, The mechanisms responsible for the interactions among oxalate, pH, and Fe dissolution in PM2.5, ACS Earth Space Chem., 2019, 3, 2259–2265, DOI:10.1021/acsearthspacechem.9b00172.
- H. Chen and V. H. Grassian, Iron dissolution of dust source materials during simulated acidic processing: the effect of sulfuric, acetic, and oxalic acids, Environ. Sci. Technol., 2013, 47, 10312–10321, DOI:10.1021/es401285s.
- A. Ito, Atmospheric processing of combustion aerosols as a source of bioavailable iron, Environ. Sci. Technol. Lett., 2015, 2, 70–75, DOI:10.1021/acs.estlett.5b00007.
- S. Myriokefalitakis, E. Bergas-Massó, M. Gonçalves-Ageitos, C. Pérez García-Pando, T. van Noije, P. Le Sager, A. Ito, E. Athanasopoulou, A. Nenes, M. Kanakidou, M. C. Krol and E. Gerasopoulos, Multiphase processes in the EC-Earth model and their relevance to the atmospheric oxalate, sulfate, and iron cycles, Geosci. Model Dev., 2022, 15, 3079–3120, DOI:10.5194/gmd-15-3079-2022.
- C. Baldo, A. Ito, M. D. Krom, W. Li, T. Jones, N. Drake, K. Ignatyev, N. Davidson and Z. Shi, Iron from coal combustion particles dissolves much faster than mineral dust under simulated atmospheric acidic conditions, Atmos. Chem. Phys., 2022, 22, 6045–6066, DOI:10.5194/acp-22-6045-2022.
- B. J. Majestic, A. D. Anbar and P. Herckes, Stable isotopes as a tool to apportion atmospheric iron, Environ. Sci. Technol., 2009, 43, 4327–4333, DOI:10.1021/es900023w.
- M. Kurisu, Y. Takahashi, T. Iizuka and M. Uematsu, Very low isotope ratio of iron in fine aerosols related to its contribution to the surface ocean, J. Geophys. Res.: Atmos., 2016, 121, 11119–11136, DOI:10.1002/2016JD024957.
- Y. Wang, L. Wu, W. Hu, W. Li, Z. Shi, R. M. Harrison and P. Fu, Stable iron isotopic composition of atmospheric aerosols: an overview, npj Clim. Atmos. Sci., 2022, 5, 75, DOI:10.1038/s41612-022-00299-7.
- T. M. Conway, D. S. Hamilton, R. U. Shelley, A. M. Aguilar-Islas, W. M. Landing, N. M. Mahowald and S. G. John, Tracing and constraining anthropogenic aerosol iron fluxes to the North Atlantic Ocean using iron isotopes, Nat. Commun., 2019, 10, 2628, DOI:10.1038/s41467-019-10457-w.
- M. Kurisu, K. Sakata, M. Uematsu, A. Ito and Y. Takahashi, Contribution of combustion Fe in marine aerosols over the Northwestern Pacific estimated by Fe stable isotope ratios, Atmos. Chem. Phys., 2021, 21, 16027–16050, DOI:10.5194/acp-21-16027-2021.
- P. Pinedo-González, N. J. Hawco, R. M. Bundy, E. V. Armbrust, M. J. Follows, B. B. Cael, A. E. White, S. Ferrón, D. M. Karl and S. G. John, Anthropogenic Asian aerosols provide Fe to the North Pacific Ocean, Proc. Natl. Acad. Sci. U.S.A., 2020, 117, 27862–27868, DOI:10.1073/pnas.2010315117.
- V. E. Fioletov, C. A. McLinden, D. Griffin, I. Abboud, N. Krotkov, P. J. T. Leonard, C. Li, J. Joiner, N. Theys and S. Carn, Version 2 of the global catalogue
of large anthropogenic and volcanic SO2 sources and emissions derived from satellite measurements, Earth Syst. Sci. Data, 2023, 15, 75–93, DOI:10.5194/essd-15-75-2023.
- S. D. Rathod, D. S. Hamilton, N. M. Mahowald, Z. Klimont, J. J. Corbett and T. C. Bond, A mineralogy-based anthropogenic combustion-iron emission inventory, J. Geophys. Res.: Atmos., 2020, 125, e2019JD032114, DOI:10.1029/2019JD032114.
- C. S. Buck, W. M. Landing, J. A. Resing and G. T. Lebon, Aerosol iron and aluminum solubility in the northwest Pacific Ocean: results from the 2002 IOC cruise, Geochem. Geophys. Geosyst., 2006, 7, Q04M07, DOI:10.1029/2005GC000977.
- J. Wu, R. Rember and C. Cahill, Dissolution of aerosol iron in the surface waters of the North Pacific and North Atlantic Oceans as determined by a semicontinuous flow-through reactor method, Global Biogeochem. Cycles, 2007, 21, GB4010, DOI:10.1029/2006GB002851.
- A. R. Bowie, D. Lannuzel, T. A. Remenyi, T. Wagener, P. J. Lam, P. W. Boyd, C. Guieu, A. T. Townsend and T. W. Trull, Biogeochemical iron budgets of the Southern Ocean south of Australia: decoupling of iron and nutrient cycles in the subantarctic zone by the summertime supply, Global Biogeochem. Cycles, 2009, 23, GB4034, DOI:10.1029/2009GB003500.
- A. R. Baker, M. Thomas, H. W. Bange and E. P. Sánchez, Soluble trace metals in aerosols over the tropical South-east Pacific Offshore of Peru, Biogeosciences, 2016, 13, 817–825, DOI:10.5194/bg-13-817-2016.
- M. M. G. Perron, B. C. Proemse, M. Strzelec, M. Gault-Ringold, P. W. Boyd, E. Sanz Rodriguez, B. Paull and A. R. Bowie, Origin transport and deposition of aerosol iron to Australian coastal waters, Atmos. Environ., 2020, 228, 117432, DOI:10.1016/j.atmosenv.2020.117432.
- M. Nakane, T. Ajioka and Y. Yamashita, Distribution and Sources of Dissolved Black Carbon in Surface Waters of the Chukchi Sea, Bering Sea, and the North Pacific Ocean, Front. Earth Sci., 2017, 5, 34, DOI:10.3389/feart.2017.00034.
- N. Moteki, Capabilities and limitations of the single-particle extinction and scattering method for estimating the complex refractive index and size-distribution of spherical and non-spherical submicron particles, J. Quant. Spectrosc. Radiat. Transfer, 2020, 243, 106811, DOI:10.1016/j.jqsrt.2019.106811.
- N. Moteki, Measuring the complex forward-scattering amplitude of single particles by self-reference interferometry: CAS-v1 protocol, Opt. Express, 2021, 29, 20688–20714, DOI:10.1364/OE.423175.
|
This journal is © The Royal Society of Chemistry 2023 |