DOI:
10.1039/D0FO01659D
(Paper)
Food Funct., 2021,
12, 70-82
High-internal-phase emulsions (HIPEs) for co-encapsulation of probiotics and curcumin: enhanced survivability and controlled release
Received
25th June 2020
, Accepted 2nd October 2020
First published on 10th November 2020
Abstract
Synergistic biological activities of probiotics and curcumin can be achieved based on the gut–brain axis. However, it is still a challenge for utilizing both of them in actual food products due to their high sensitivity to environmental conditions. In the present study, high-internal-phase emulsions (HIPEs) were fabricated to co-encapsulate the probiotics and curcumin in response to the customer demand for convenience. β-Lactoglobulin-propylene glycol alginate composite hydrogel particles (β-lgPPs) with proper size and intermediate wettability were prepared at β-lg to PGA mass ratio of 2
:
1 and employed as particulate emulsifiers. Stable HIPEs with a fixed oil fraction (φ = 0.8) could be formed within a wide range of β-lgPPs concentrations, ranging from 0.1 to 2.0 wt%. Confocal laser scanning microscopy (CLSM) images indicated that the interfacial structure of the oil droplets was composed of both β-lg nanoparticles and a PGA network, which jointly contributed to the gel-like structures in HIPEs. An increase in elasticity and gel strength, as well as centrifugal stability, could be achieved by elevating the particle concentration as determined by diffusing wave spectroscopy and Lumisizer analysis. HIPEs with high particle concentrations showed a high resistance against pasteurization since no obvious flocculation or coalescence could be observed in these emulsions. HIPEs also provoked a significant reduction in the death of LGG as well as the chemical degradation of curcumin: up to 7.91 log CFU cm−3 of LGG and 93.0% of curcumin were retained after pasteurization treatment. Moreover, the HIPEs could also retard the release of curcumin and protect the LGG in simulated gastrointestinal tract conditions. The results from this work provide useful information for developing a promising delivery system for the co-encapsulation of curcumin and probiotics.
1. Introduction
Probiotics are live microorganisms which confer a health benefit on the host when administered in adequate amounts.1 It has been well established that microbiota may exert health-promoting effects in the host through various mechanisms, for instance, provision of nutrients and protection against pathogenic bacteria.2–4 The regulation of intestinal microbiota may be primarily accomplished through dietary means, and for this reason the development of probiotic-based products has been a subject of extensive investigations among the scientific and industrial community. In order to ensure desired results, probiotics should maintain metabolically active and remain present in a sufficiently large concentration (at least 107 CFU g−1) in final products.5 However, most probiotics are sensitive to environmental conditions, making it a challenge for directly utilizing them in practical food systems. Besides, the probiotic cells taken orally are liable to lose their activities during gastrointestinal tract (GIT) passage, especially under acidic stomach conditions, significantly reducing the number of viable cells.6 Currently, extensive approaches for probiotics protection have been explored to preserve the viability of probiotic bacteria during commercialization and gastrointestinal digestion, such as spray drying, gelation and emulsification.7–10
Curcumin extracted from the plant Curcuma longa is a kind of hydrophobic bioactive polyphenolic compound.11 Curcumin can exert a positive effect on the human gut microbiota through the gut–brain axis, which is a continuous communication between the gut and the brain via neurotransmitters.12 Increasing evidences indicate that curcumin and probiotics are capable of performing a synergistic effect for the digestive health, which commonly manifests as gut flora revivification and immunity enhancement.13–17 However, the direct use of curcumin in food products is highly restricted due to its poor water solubility and light sensitivity. In order to achieve a synergistic function and convenience, appropriate encapsulation techniques and formulations are required for the co-encapsulation of both probiotics and curcumin.
The critical factors that affect the viability of probiotics are commonly considered to be both a low relative humidity (RH) and temperature.18 A large proportion of food systems contain an aqueous environment. Moreover, dried microcapsules may be infiltrated promptly by water molecules, resulting in the inactivation or possibly death of the probiotics. Based on the above-mentioned requirements, emulsions seem to be a desired vehicle to elevate the survival rate of probiotics. Moreover, curcumin can be easily dissolved in the oil phase due to its lipid-solubility, providing the possibility of co-encapsulating both probiotics and curcumin.
High-internal-phase emulsions (HIPEs) generally refer to emulsions with an internal phase volume fraction of more than 74% or 64% for hexagonal close packing or random close packing of the droplets, respectively.19 Taking advantage of their special features, such as a large surface area per unit volume of continuous phase and their loading capacity of functional components, HIPEs have potential applications in various industrial fields including the food, pharmaceutical, personal care, and cosmetic industries.20 In many cases, HIPEs are stabilized by large amounts of low-molecular-weight surfactants, which are generally subject to concerns about health and safety. An alternative strategy is to fabricate stable HIPEs using microgel particles. Particles with prominent surface-activity are able to be irreversibly adsorbed onto the oil/water interface.21 The resulting rigid layer around the droplets endows emulsions with an outstanding stability against Ostwald ripening, creaming and coalescence even at high internal phase concentrations.22 In our previous study, we have demonstrated that β-lactoglobulin-propylene glycol alginate composite nanoparticles (β-lgPPs) are effective particulate stabilizers and that highly stable Pickering emulsions can be fabricated with 1.0 wt% β-lgPPs.23 As an extension of that work, HIPEs were fabricated using β-lgPPs particles in the current study, which were evaluated for the co-encapsulation of probiotics and curcumin.
Lactobacillus rhamnosus is a group of facultative anaerobe-lactic acid bacteria extensively used as probiotics in food formulations, healthy foods and functional foods.24 In the current study, Lactobacillus rhamnosus GG (LGG) was employed as a probiotic model and encapsulated in the internal phase of HIPEs that were stabilized by β-lgPPs. To date, little research is ongoing to explore the encapsulation of probiotics, and especially the co-encapsulation of curcumin and LGG in the same system, as is done in this work. Accordingly, this study aimed to explore the physical properties of HIPEs stabilized using β-lgPPs, and to verify the protective effect and controlled release of both curcumin and LGG against pasteurization treatment as well as simulated gastrointestinal conditions. The results from this work provide essential information to develop a simple and effective delivery system for the co-encapsulation of functional components and probiotics.
2. Materials and methods
2.1. Materials
β-Lactoglobulin (β-lg) was obtained from Davisco Foods International Inc. (Le Sueur, USA). Propylene glycol alginate (PGA) (87.9% esterified carboxyl groups, 100 kDa) was kindly donated by Hanjun Sugar Industry Co. Ltd (Shanghai, China) and hydrolyzed in our lab. Curcumin (Cur, 98%) was purchased from the China National Medicine Group (Shanghai, China). A lyophilized culture of Lactobacillus rhamnosus GG (LGG, ATCC 53103) was obtained from China General Microbiological Culture Collection Center. The Live/Dead® BacLight™ Bacterial Viability Kit L7012 was purchased from Thermofisher Scientific, USA. Soybean oil (viscosity = 61 mPa s at 20 °C; containing at least 48.0% linoleic acid, 17.0% oleic acid, and 8.0% palmitic acid) was obtained from Yihai Kerry Oils & Grains Co. (Shanghai, China). All other chemical reagents used in the present study were of analytical grade.
2.2. Encapsulation of Lactobacillus rhamnosus in HIPEs
2.2.1. Preparation of freeze-dried Lactobacillus rhamnosus GG (LGG).
A stock LGG culture was sub-cultured twice by inoculating in MRS broth and incubating in a CO2 incubator (37 °C, 16 h) for microbial activation and adaptation. Cells in the late log phase (28 h) were harvested by centrifugation at 10
000 rpm for 10 min and washed three times using sterile normal saline. Then the suspension was uniformly mixed with the ten-fold volume of freeze-dried protective material (consisting of 18 wt% skim milk powder, 3.0 wt% trehalose and 0.4 wt% sodium acetate) prior to freeze-drying for 24 h using an Alpha 1-2 D Plus freeze-drying apparatus (Marin Christ, Germany). The viable counts of LGG in the lyophilized mixed powder were determined to achieve a population of 10.0 ± 0.1 log cfu g−1. The final powder was stored at −20 °C until use.
2.2.2. Emulsion preparation.
β-Lactoglobulin-propylene glycol alginate composite nanoparticles (β-lgPPs) were prepared based on an electrostatic deposition method as described by Su et al.23 Briefly, β-lg nanoparticles were prepared though heating a 4.0 wt% β-lg solution at pH 5.8 at 95 °C for 5 min. Then, the β-lg nanoparticles dispersion was mixed with an equal volume of a PGA solution (2.0 wt%). This mixture was adjusted to pH 4.0, and stirred continuously for 2 h to ensure the formation β-lgPPs.
HIPEs were then fabricated within a wide range of β-lgPPs concentrations (c = 0.1–2.0 wt%) using a fixed oil volume fraction (φ = 0.8). An oil phase was prepared through suspending the obtained powder of Lactobacillus rhamnosus (1.25%, w/v) in soybean oil containing 0.5 wt% curcumin. The oil phase was slowly added into the β-lgPPs dispersion with the shearing of an Ultra-Turrax (IKA, Germany) at a speed of 12
000 rpm for 5 min to form HIPEs. A total volume of 30 mL was settled for each sample and the obtained emulsions were stored at 4 °C (to minimize microbial growth) before further experiments.
2.3. Physical characterization of HIPEs
2.3.1. Size distribution, micromorphology and wettability of β-lgPPs.
The particle size distribution of the β-lgPPs dispersion was analyzed using a Zetasizer Nano-ZS90 (Malvern Instruments, Worcestershire, UK) through dynamic light scattering (DLS). The micromorphology of the β-lgPPs was observed by an atomic force microscope (AFM) (DI Nanoscope TV, Veeco Company, Plainview, NY) equipped with an E-scanner. The tapping mode was employed with a nominal spring constant of 20–100 N m−1 and nominal resonance frequencies of 10–200 kHz.
The three-phase contact angle (θo/w) of β-lgPPs was determined using an OCA system (DataPhysics, GmbH, Berlin, Germany) based on a sessile drop method.25 The contact angle was calculated automatically with the SCA 20 software according to the Laplace–Young equation. Measurements were averaged over at least ten drops to avoid manual errors.
2.3.2. Drop test.
The type of emulsions was defined immediately after preparation through a drop test method described by Liu & Tang.26 Briefly, a drop of emulsion was dispersed into either deionized water or pure soybean oil. The emulsion was considered to be oil-in-water (o/w) if the droplet dispersed rapidly in the aqueous phase and remained aggregated in the oil phase, whereas it was regarded as a water-in-oil (w/o) emulsion in the opposite case.
2.3.3. Droplet size measurement.
The droplet size of HIPEs was determined using a static light scattering analyzer (LS 13 320, Beckman Coulter, Brea, CA, USA). Results were reported as the volume-weighted mean diameter (D4,3) of the droplets.
2.3.4. Microstructure observation.
The microstructure of HIPEs with various particle concentrations was visualized using an optical microscope (Leica DMD 108, Leica Micro-systems Inc., Germany) equipped with a camera.
For the purpose of confirming the location of the β-lgPPs in HIPEs, a confocal laser scanning microscope (CLSM) (Leica TCS SP5, Leica Microsystems Inc., Germany) was employed according to a previously reported method.27 The samples were stained by a mixed staining solution containing Nile Blue (0.1%) and Nile red (0.1%). The fluorescent stains were excited by either a He–Ne laser at 633 nm or an argon laser at 488 nm for Nile Red and FITC.
2.3.5. Creaming behavior.
The creaming stability of HIPEs was monitored using an analytical centrifugal analyzer (LUMiSizer, GmbH, Berlin, Germany) based on the procedure described by Wang et al.28 Data were recorded at 25 °C and 3000 rpm with a time interval of 10 s for 3600 s and analyzed using the SEP view 5.1 software.
2.3.6. Microrheological properties.
The microrheological properties of HIPEs were characterized using a Rheolaser Master (Formulaction, France). A microrheology test of 6 h was carried out on the emulsion samples at room temperature. The Brownian motion of the droplets was calculated through the dynamic speckle images and reported as the mean square displacement (MSD) versus time. The Solid–Liquid Balance (SLB), macroscopic viscosity index (MVI) and elasticity index (EI) parameters of the samples were also calculated using the software RheoSoft Master 1.4.0.0.
2.4. Evaluation of stability against pasteurization
2.4.1. Pasteurization conditions.
HIPEs were subjected to a specific heat treatment to simulate low temperature long time (LTLT) pasteurization. Briefly, freshly prepared samples were heated at 63 °C for 30 min in a water bath prior to cooling down to room temperature in an ice bath. The survival rate of LGG and the retention rate of curcumin were determined after the simulated pasteurization process.
2.4.2. Flocculation and coalescence degree.
The droplet size of the HIPEs after pasteurization treatment was measured with the methods described in 2.3.3. Samples were diluted with either distilled water or a 2.0% (w/v) sodium dodecyl sulphate (SDS) solution to dissociate weak bonds. The flocculation degree (FD) and coalescence degree (CD) of the emulsion gel samples were calculated according to the following equations:
2.4.3. Quantification of curcumin.
The curcumin content of HIPEs was quantified according to the method described previously using a spectrophotometer (UV-1800, Japan).29 The content of curcumin was expressed as relative concentration (RC, in %), which was obtained through dividing the determined curcumin content by the actual added amount of curcumin.
2.4.4. Enumerated viable cells of LGG.
Flow cytometry analysis was conducted using a flow cytometer (FACSCalibur 2, USA) according to a previously reported method10 to enumerate the viable cells of LGG. Diluted samples were centrifuged at 1 × 105 g and 4 °C for 30 min to release the cells from the oil phase, then the sediment was collected and washed twice using phosphate buffered saline (pH 7). The obtained cell dispersions were subsequently dyed by a Dead/Alive kit (commercial LIVE/DEAD® BacLight™) prior to incubating at 37 °C for 15 min and kept on ice until analysis. Excitation was accomplished using an argon ion laser at a wavelength of 488 nm, and green fluorescence and red fluorescence were detected above 530/30 nm (FL1) and 670 nm (FL3), respectively. The acquired data were analyzed using the BD Cell Quest Pro software. A positive control was prepared by heating naked bacteria at 63 °C for 30 min and a negative control was prepared using free bacteria. At least 40
000 bacteria were collected for each analysis.
2.5. Survival of Lactobacillus rhamnosus against gastrointestinal conditions
2.5.1. Simulated gastrointestinal digestion.
To simulate the conditions of the human gastrointestinal tract (GIT), a two-stage gastrointestinal model was employed according to the method reported previously.30 Each emulsion was passed through a GIT model that consisted of gastric and small intestine phases. The incubation temperature was maintained at 37 °C using a water bath. Measurements of the content of curcumin and of the survival rate of LGG were carried out after the samples were exposed to each stage. The digestive fluid was regularly sampled with an interval of 30 min.
Stomach phase.
30 mL of the sample was mixed with 30 mL of simulated gastric fluid (SGF) containing 3.2 mg mL−1 pepsin prior to adjusting to pH 1.2. Then the mixture was placed in a water bath at 37 °C with continuous swirling at 100 rpm for 1 h of simulated gastric digestion.
Small intestine phase.
30 mL of the obtained gastric digestion was adjusted to pH 7.0 and mixed with an equal volume of simulated intestinal fluid (SIF) that consists of 10.0 mg mL−1 bile salt and 0.4 mg mL−1 pancreatin. Mixtures were then incubated at 37 °C with continuous swirling at 100 rpm for 2 h to simulate small intestine digestion.
2.5.2. Quantification of curcumin.
The retention rate of curcumin in the emulsion gels after SGF and SIF digestion was determined based on the method described in 2.4.3.
2.5.3. Enumerated viable cells of LGG.
The survival rate of LGG in HIPEs after simulated gastrointestinal digestion was measured by plate counting. Sequential dilutions were performed using peptone (0.1%, w/v) and each dilution was plated on a MRS. The colonies were grown under anaerobic conditions (48 h, 37 °C) prior to enumerate the bacteria in triplicate using the traditional microbial plating method.
2.6. Statistical analysis
Data were subjected to analysis of variance (ANOVA) using the software package SPSS 18.0 (SPSS Inc., Chicago, USA). Statistical differences were determined by one-way analysis of variance (ANOVA) with Duncan procedure and a significant level of p < 0.05 was chosen.
3. Results and discussion
3.1. Physical properties of β-lgPPs
Instead of forming microgel particles with the aid of chemical cross-linking agents, the fabrication process of composite microgel particles in the current work can be divided into two steps. (1) Heating an aqueous solution of β-lg was heated above its denaturation temperature under specific conditions to form β-lg microgel particles through both hydrophobic and covalent interactions. (2) Utilization of electrostatically deposited onto the β-lg particles to prepare β-lg-PGA composite particles with improved wetting ability. According to the report of Donato et al.,31 heating 1 wt% β-lg solution at pH 5.7–5.9 and a temperature of 70 to 85 °C resulted in the formation of well-defined β-lg particles with a finite size between 200 and 300 nm, a low polydispersity index (PDI) and a spherical to ellipsoidal structure. However, the formed β-lg particles are primarily hydrophobic, seriously affecting their emulsifying capacity.32 As a consequence, negatively-charged PGA molecules were employed to adsorb onto the surface of the positively charged β-lg particles in an acid environment, leading to the formation of composite particles with a core–shell structure and proper wettability via electrostatic deposition, as described into more detail in one of our recent publications.23
The stability of the emulsions containing microgel particles is highly correlated to the physical attributes of the particles, especially their size and wettability. As presented in Fig. 1A, the β-lgPPs followed a unimodal size distribution with a mean diameter of 276 nm and a polydispersity index (PDI) of 0.11. The PDI value is a parameter that reflects the size distribution of colloidal particles: a small PDI value (generally lower than 0.3) indicates a narrow size distribution.33 The relatively low PDI value indicated that the β-lgPPs fabricated in the current work had a narrow size distribution and could be considered as a homogeneous dispersion. An AFM image of the β-lgPPs is also depicted in Fig. 1A. Apparently, the β-lgPPs exhibited a well-defined spherical structure with a particle size ranging from 250 nm to 300 nm, which is consistent with the dynamic light scattering results. The AFM image also indicated that the β-lgPNPs were microgel particles with a hard core since no obvious shrinking could be observed. Moreover, drop shape analysis was conducted to investigate the wettability characteristics of the β-lgPPs by quantifying the three-phase contact angle (θ). In order to facilitate the efficient packing of the colloidal particles at the oil/water interface, the particles should be equally wetted by both oil and water, which generally is manifested as a three-phase contact angle (θo/w) that approximates 90°.34 As shown in Fig. 1B, the three-phase contact angle θ formed at the boundary of β-lgPPs, aqueous phase and oil phase was 90.8 + 0.1°, suggesting that the β-lgPPs showed a desirable wettability and could be employed as well-performing particulate emulsifiers.
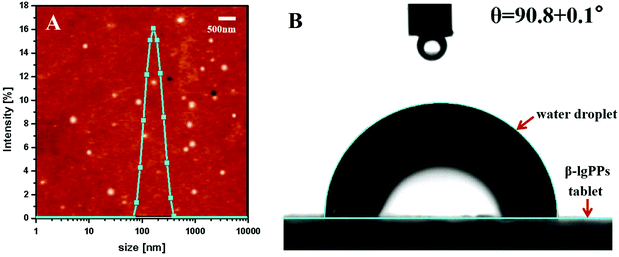 |
| Fig. 1 Particle size distribution (blue curve in part A) and AFM image (background image of part A), as well as typical three-phase contact angle (θ) (B) of β-lgPPs at a β-lg to PGA mass ratio of 2 : 1 at pH 4.0. | |
3.2. Droplet characterization and interfacial structure observation
A drop test was performed on the HIPEs stabilized by β-lgPPs with various particle concentrations (c) at a fixed oil volume fraction (φ = 0.8) to determine whether they were either oil-in-water (o/w) or water-in-oil (w/o) emulsions. At a β-lgPPs concentration (c) of 0.05%, a droplet of HIPE could maintain its spherical structure when dispersed in the water phase, but readily dispersed in the oil phase, implying that the HIPE existed as a w/o emulsion in such condition. A similar result was also reported by Xiao et al.,35 who also found that emulsions stabilized by Kafirin nanoparticles easily underwent phase inversion with a low particle concentration and a high oil fraction. Upon increasing the β-lgPPs concentration from 0.1 to 2.0%, all the emulsion droplets were easily dispersed in the aqueous phase, suggesting the existence of these HIPEs as o/w emulsions. Fig. 2A displays the typical appearance of HIPEs stabilized by β-lgPPs with various particle concentrations at a fixed oil volume fraction (φ = 0.8). Apparently, the emulsified phase of the HIPEs occupied the vast majority of the volume of the whole sample, and HIPEs with a β-lgPPs concentration of 1.0 and 2.0% contained an emulsified phase without clear serum phase. As expected, the samples remained intact when inversely placed after 3 h of storage (Fig. 2B), signifying that the HIPEs transformed from a liquid-like state to a solid-like state. Prolonging the storage time to 4 weeks at 4 °C, no sign of oil release was observed in all samples (Fig. 2C), revealing that HIPEs fabricated via β-lgPPs exhibited a remarkable storage stability even at such an extremely high oil volume fraction.
 |
| Fig. 2 Visual appearance 3 h (A and B) and 4 weeks (C) after preparation and optical microscopy images (D) of HIPEs stabilized by various concentrations of β-lgPPs (c, expressed in %) with a fixed oil volume fraction (φ = 0.8). CLSM images of HIPEs (E) and enlarged image of droplets (F) stabilized by β-lgPPs with a particle concentration of 1.0 wt%: soybean oil was stained with Nile Red (appearing in red) and β-lgNPs was stained by Nile Blue (appearing in green), whereas PGA was labeled with FITC (appearing in blue). | |
In view of the fact that the stability of emulsions is largely affected by the size of the emulsion droplets, the mean droplet size of these HIPEs was determined immediately after preparation. As shown in Table 1, the volume-weighed average diameters (D4,3) showed a significantly decreasing trend from 78.1 μm to 43.7 μm upon increasing the particle concentration from 0.1 to 2.0 wt%. It may therefore be speculated that the dimensions of the emulsion droplets tend to become smaller when a sufficiently large number of particulate emulsifier particles were provided in the system.36 Provided that all other conditions are invariant, emulsions with smaller droplet size are generally considered to be more stable. On the other hand, smaller emulsion droplets have a larger surface area, which requires more emulsifier for the stabilization of the oil–water interfaces. In view of the small amount of stabilising particles (i.e. max 2% for 80% of oil), large emulsion droplets were generated in order to ensure the adequate surface coverage of the oil–water interfaces by β-lgPPs. As such, the chances of emulsion instability phenomena, such as aggregation and coalescence, was minimised. Inversely, the presence of a sufficiently large concentration of β-lgPPs might facilitate the formation of emulsion droplets with smaller size, as the β-lgPPs are capable of adequately covering a larger interfacial area. It is also noteworthy that the volume-weighed mean diameter (D4,3) of all the samples showed little variation after a 4-week storage, indicating that no obvious flocculation or coalescence occurred in these emulsions within the range of all tested particle concentrations.
Table 1 Volume-weighted mean diameter (D4,3) of the HIPEs as a function of particle concentration at a fixed oil volume fraction with or without the addition of SDS, as well as flocculation degree (FD) and coalescence degree (CD) of HIPEs after pasteurization
Particle concentration (wt%) |
D
4,3 of the samples (μm) |
FD (%) |
CD (%) |
D
4,3
|
D
4,3 after 4-week storage
|
D
4,3 with SDS
|
D
4,3 after pasteurization
|
D
4,3 with SDS after pasteurization
|
Values are means ± standard deviation of triplicate analyses. Different superscript letters in the same column indicate significant differences (p < 0.05). |
0.1 |
78.1 ± 0.4a |
79.3 ± 0.7a |
77.8 ± 0.7a |
93.5 ± 0.3a |
91.7 ± 0.5a |
2.19a |
17.99a |
0.3 |
70.8 ± 0.8b |
70.5 ± 0.5b |
70.4 ± 0.6b |
79.2 ± 0.4b |
78.3 ± 0.6b |
1.28b |
11.22b |
0.5 |
59.3 ± 0.5c |
60.2 ± 0.4c |
57.9 ± 0.7c |
64.3 ± 0.2c |
62.9 ± 0.8c |
2.42c |
8.64c |
1.0 |
55.4 ± 0.3d |
54.8 ± 0.5d |
55.2 ± 0.4d |
58.4 ± 0.3c |
57.1 ± 0.9d |
2.36d |
3.44b |
2.0 |
43.7 ± 0.3e |
44.6 ± 0.3e |
42.8 ± 0.3e |
44.7 ± 0.2d |
43.9 ± 0.2e |
1.87e |
2.57d |
The microstructure of the β-lgPPs stabilized HIPEs is displayed in Fig. 2D. The droplet size obviously decreased with increasing particle concentration at a constant oil volume fraction, as evidenced by the static light scattering results. In order to confirm the location of the β-lgPPs at the oil/water interface, a HIPE stabilized by 1.0 wt% β-lgPPs was selected to conduct a CLSM observation. Images were captured from the same region of the HIPE through exciting either a single stain or all stains. Fig. 2E shows the CLSM image of the HIPEs in the green fluorescence field (showing the β-lgNPs, part a), the red fluorescence field (showing the soybean oil, part b), the blue fluorescence field (showing the PGA, part c) and the overlapping fluorescence field (d), respectively. The red spherical regions were surrounded by green hollow circles, indicating that a densely packed layer was formed at the boundary of the oil droplets, sterically hindering the coalescence of droplets. It is also noteworthy to mention that the image in the blue fluorescence field occupied the free space between the droplets, which might be explained by the fact that the outer PGA molecules of β-lgPPs could cross-link together to form a network-like framework in the continuous phase.37 Enlarging one of the droplets to further investigate the adsorption behavior at the oil/water interface (Fig. 2F), the β-lgPPs were found to adsorb at the oil/water interface, confirming their role as particulate emulsifiers.
3.3. Microrheological properties of HIPEs
HIPEs stabilized by particulate emulsifiers commonly exhibit a solid-like flow behavior with both viscous and visoelastic properties, which are responsible for the extraordinary stability of HIPEs against coalescence.38 The mean square displacement (MSD) curves are able to reflect the viscous and viscoelastic properties of the samples through monitoring the Brownian motion of droplets in HIPEs. Representative curves of MSD versus decorrelation time for HIPEs with different particle concentrations are displayed in Fig. 3. It is obvious that the MSDs changed with the decorrelation time (τ). In the initial stages, all samples gave a MSD curve that scaled linearly with τ: MSD = Aτ, indicating a purely viscous attribute of the samples. However, the MSD curves were found to be more broadened with the advent of obvious plateau regions, which suggested that samples experienced a changing process from a rather dispersed fluid state to a highly structured lipid state. The Solid–Liquid Balance (SLB) value, which can quantify the ratio between the solid-like and liquid-like behavior of the sample, was also calculated to further confirm the changes in these samples. Generally speaking, a SLB value higher than 0.5 signifies that the liquid behavior dominates, while a SLB value close to 0 indicates that the sample is visoelastic/solid-like. As shown in Table 2, all the HIPEs with particle concentrations ranging from 0.1 to 2.0 wt% were measured to be less than 0.48, suggesting a more solid behavior of these samples. Moreover, the decreasing trend in SLB values with increasing particle concentration further confirmed that the elastic strength and particle concentration displayed a positive correlation in these HIPEs.
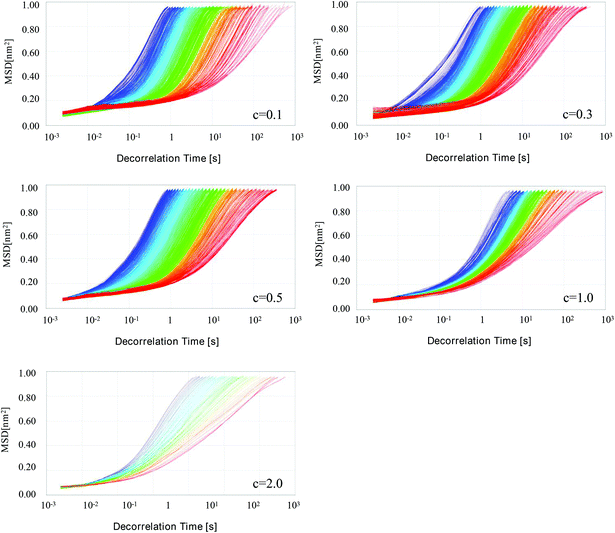 |
| Fig. 3 Typical examples of the Mean Square Displacement (MSD) versus time curves for β-lgPPs stabilized HIPEs with various particle concentrations ranging from 0.1 wt% to 2.0 wt% at a fixed oil volume fraction (φ = 0.8). Different colors refer to different scanning times during the MSD analysis, from blue over green to red. | |
Table 2 The Solid Lipid Balance (SLB), Macroscopic Viscosity Index (MVI) and Elasticity Index (EI) values for HIPEs with a fixed oil volume fraction (φ = 0.8) as a function of particle concentration
Particle concentration (wt%) |
SLB |
MVI (10−1) |
EI (10−1) |
Different superscript letters in the same column indicate significant differences (p < 0.05). |
0.1 |
0.48 ± 0.003a |
3.97 ± 0.002a |
2.16 ± 0.001a |
0.3 |
0.37 ± 0.003b |
6.26 ± 0.002b |
3.98 ± 0.001b |
0.5 |
0.26 ± 0.002c |
8.33 ± 0.003c |
5.41 ± 0.003c |
1.0 |
0.15 ± 0.002d |
12.38 ± 0.004d |
6.26 ± 0.004d |
2.0 |
0.11 ± 0.001e |
14.56 ± 0.004e |
7.03 ± 0.005e |
The macroscopic viscosity index (MVI) and elasticity index (EI) parameters were automatically calculated from the MSD curves to provide more information about the rheological characteristics of these HIPEs (Table 2). The MVI value corresponds to the inverse of the diffusional speed of the particles for long times, quantifying the macroscopic zero-shear viscosity, whereas the EI value is relevant to the travelling distance of droplets required before getting trapped in the network, which reflects the elasticity strength of the samples. Both MVI and EI values revealed a general increasing trend, indicating an increase in both viscous and viscoelastic properties of the HIPEs as the particle concentration increased from 0.1 to 2.0 wt%. Such phenomenon could be easily comprehended with the following explanation based on a “cage” model.39 Presumably, emulsion droplets enjoy a relatively mobile freedom to move inside a “cage”, which is fabricated through interactions with neighbouring droplets. An increased particle concentration led to a decrease in the droplet size, and hence an increase in the number of droplets, facilitating the rearrangement of droplets in the emulsions. As the oil volume fraction was constant, the droplets became more and more densely packed in the HIPEs. Therefore, the movement of droplets, including moving range and velocity, was strictly limited due to the reduced space of the “cage”, resulting in the an enhanced viscosity and elasticity in the emulsions. A previous research reported that a greater gel strength and viscosity could be induced through increasing the oil fraction in HIPEs stabilized via ovotransferrin/gum arabic particles.33
3.4. Creaming stability of HIPEs
The influence of particle concentration on the creaming stability of HIPEs was evaluated by monitoring the transmission changes by means of the LUMiSizer. Fig. 4A exhibits the time-dependent integral transmission profiles of HIPEs at different particle concentrations for a visual comparison. In these profiles, the position at 130 mm corresponds to the bottom of the sample, whereas the position at approximately 105 mm represents the top of the sample. Generally, a high transmission indicates a low concentration of emulsion droplets, whereas a low transmission signified a high concentration of droplets. As presented in Fig. 4A, all the first profiles were located at the bottom (red lines) and the last profiles existed in the top (green line). This could be explained by the fact that the oil droplets in HIPEs had a tendency to move upwards under the effect of centrifugal force due to the density difference between the emulsified droplets and the aqueous phase. The differences between the profiles recorded at different times during the centrifugation became smaller with increasing particle concentration, indicating an increased creaming stability of the HIPEs. Specifically, only minor changes in the normalized transmission (expressed in %) could be observed in the bottom layer of the samples with the highest particle concentration (2.0 wt%), suggesting that only some slight creaming might occur in these emulsions. Moreover, there was hardly any oil released from the emulsified droplets, implying a high resistance of these emulsions against coalescence even in a centrifugal field. Theoretically speaking, the emulsified oil phase in an emulsion will move to the top under the effect of centrifugal force, leading to obvious phase separation. However, the HIPEs fabricated in the current work exhibited a remarkable stability against centrifugation, which was considered to be triggered by the remarkable emulsifying and emulsion stabilising capacity of β-lgPPs, as well as the presence of a PGA network in these HIPEs.
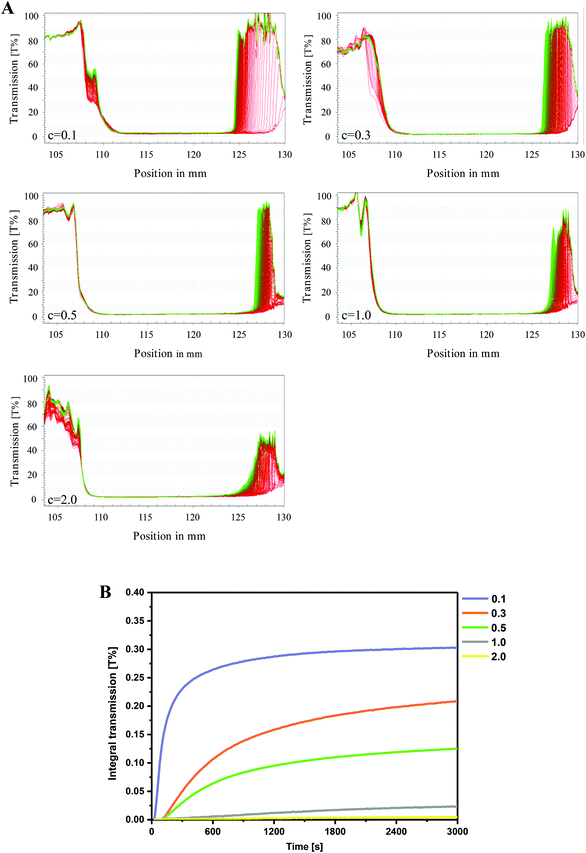 |
| Fig. 4 Time-dependent integral transmission profiles (A) and creaming rate (B) as a function of particle concentration (c = 0.1–2.0 wt%) for β-lgPPs stabilized HIPEs with a fixed oil volume fraction (φ = 0.8). The creaming rate was expressed as the slope of the integrated transmission-time plot determined during centrifugation at 3000 rpm during 3600 s at 25 °C. | |
For the purpose of quantifying the extent of creaming, the integral transmission (%) plotted against the measuring time is summarized in Fig. 4B. The slope of this curve is positively related to the “creaming rate”, which reflects the physical stability of the emulsion: the lower the creaming rate, the higher the stability.40 It was obvious that a rising particle concentration led to a decrease of the creaming rate, which meant an increase in emulsion stability. Particularly, an extremely slow increase could be found in the creaming rate of HIPEs with high particle concentrations (c = 1.0–2.0 wt%) over centrifugal time, accompanied by a relatively low final value no more than 0.025, further suggesting the relatively high physical stability of these HIPEs. Two explanations may account for this phenomenon. Firstly, the oil droplets in HIPEs with higher particle concentration have been determined to have a smaller droplet size, and might be in close proximity to each other in a dense manner, resulting in a higher resistance against coalescence or demulsification. Moreover, the more compact trapping network formed by PGA molecules contributed to strengthen the gel-like structures, which can ultimately retard the movement of the oil droplets and lead to a higher stability in the case of high-speed centrifugation.
3.5. Stability assessment of HIPEs against pasteurization
Pasteurization is extensively utilized in food processing to kill many disease-causing organisms and make food safe for consumption. However, it may also lead to the occurrence of flocculation and coalescence of emulsions, which may commonly manifest as the increase in the diameter of emulsion droplets and demulsification of the emulsions.41 In this section, samples were subjected to a heat treatment at 63 °C for 30 min to simulate a pasteurization procedure, and the mean droplet size was determined in order to investigate the physical stability of HIPEs against pasteurization. For the purpose of distinguishing the flocculation and coalescence effects, HIPEs with or without pasteurization treatment were diluted with either deionized water or a SDS solution (2.0 wt%).42 The flocculation degree (FD) and coalescence degree (CD) were then calculated to quantify these instability phenomena in HIPEs. As shown in Table 1, the volume-weighted mean diameter (D4,3) of freshly prepared HIPEs with various particle concentrations had almost no change after the addition of SDS, revealing the inexistence of obvious flocculation and coalescence in these HIPEs. However, when subjected to pasteurization processing, a significant increase was observed in the D4,3 of HIPEs with relatively low particle concentrations (c = 0.1–0.3 wt%). Especially for the HIPEs that contained only 0.1 wt% of particles, the D4,3 remarkably increased from 78.1 μm to 93.5 μm and 91.7 μm in deionized water and SDS solution, respectively. The resulting high CD values also suggested that the increase in droplet size was mostly due to coalescence rather than flocculation of the droplets. However, with higher particle concentrations (c = 0.5–2.0), only small changes were observed in the D4,3 values after pasteurization treatment, accompanied by both lower FD and CD values, implying a relatively high tolerance to both flocculation and coalescence of these HIPEs against pasteurization. This also suggested that the physical stability against pasteurization of the HIPEs stabilized by β-lgPPs was enhanced by a further increase of particle concentration.
Pasteurization may also lead to the partial death of the probiotics with a significant decrease in cell viability, as well as hydrolytic degradation of curcumin, making the utilization of both curcumin and LGG in actual food products a problem that urgently needs to be solved.41 For the purpose of investigating the protective effect of HIPEs on both LGG and curcumin, flow cytometry was utilized in order to enumerate the viable cells in HIPEs. Results were displayed in dot plots (Fig. 5A). Dots in the top right quadrant (Q2) are on behalf of dead cells that are positive for both Syto 9 and PI, while dots in the bottom right quadrant (Q3) indicate viable cells, which were Syto 9 positive, but PI negative. Apparently, almost all of the dots existed in Q3 for the freshly prepared emulsions with various particle concentrations, confirming that the encapsulated LGG cells were in a good state. However, at relatively low particle concentration (0.1–0.5 wt%), most of the dots moved from Q3 to Q2 under thermal processing conditions, indicating an obvious increase in the amount of dead cells in these samples. Inversely, large amounts of dots remained in Q3 in the scatter diagram of the HIPEs with higher particle concentrations, implying that a considerable amount of LGG cells were still metabolically viable after pasteurization. The number of viable cells and the survival rate of LGG in HIPEs were also calculated for further comparison. As shown in Fig. 5B, only 7.10, 7.36 and 7.58 log CFU mL−1 of LGG cells were found to be alive in HIPEs with particle concentrations of 0.1, 0.3 and 0.5 wt%, respectively, suggesting that at least 87.3%, 77.9% and 61.7% were killed during the pasteurization process. However, with higher particle concentrations of 1.0 and 2.0 wt%, up to 79.3% and 82.0% of LGG cells survived in HIPEs, corresponding to 7.90 and 7.91 log CFU mL−1 of viable LGG cells, which was significantly higher than the minimum effective concentration (7.0 log CFU mL−1). This phenomenon was largely due to the greater gel strength and viscosity in these HIPEs, which exerted an extremely high resistance against flocculation and coalescence. Fig. 5C displayed the retention (%) of curcumin in samples upon thermal processing. It can be easily found that HIPEs stabilized with a higher particle concentration showed a significantly (P < 0.05) higher retention rate of curcumin than HIPEs with a relatively low particle concentration after pasteurization. Up to 91.7% and 93.0% of curcumin was retained in HIPEs with a particle concentration of 1.0 and 2.0 wt%, resp. Again, this can be attributed to the relatively higher physical stability against pasteurization, which contributed to prevent the occurrence of demulsification and oil release in these HIPEs.
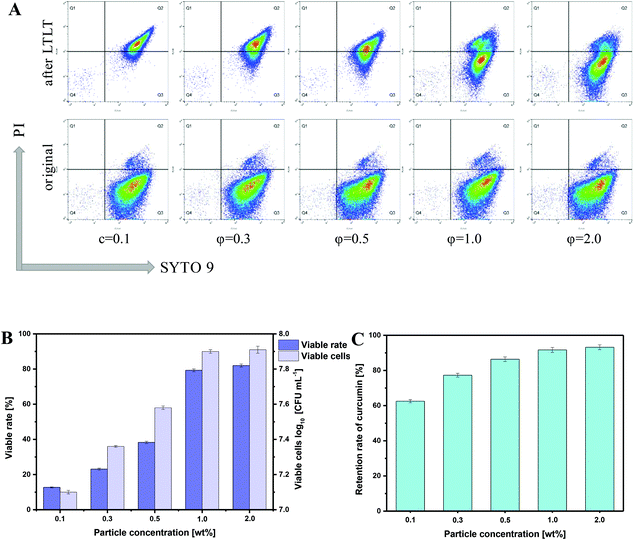 |
| Fig. 5 Representative images (A) of SYTO 9/PI double-staining analysis of LGG cells by flow cytometry. Top right quadrant (Q2), SYTO 9(+)/PI(+), dead cells; bottom right quadrant (Q3), SYTO 9(+)/PI(−), viable cells. Viable rate (%) and viable cell counts in log CFU mL−1 (B) and retention rate of curcumin (C) in HIPEs as a function of particle concentration (c = 0.1–2.0 wt%). | |
3.6. Protective effects on LGG and curcumin during in vitro simulated digestion
In order to achieve desired effects, probiotics should not only survive from the acidic gastric juice, but also remain abundant and active after arriving at the intestines.37 Moreover, the encapsulated curcumin in HIPEs is also required to possess a high bioavailability. Therefore, the viability of LGG and the bioavailability of curcumin were investigated through performing an in vitro digestion procedure on the samples. A HIPE with a fixed particle concentration of 1.0 wt% was selected on the basis of the experimental results presented above, since it has been confirmed to have a relatively high physical stability as well as remarkable protective effects on curcumin and LGG against pasteurization. As a control sample, an oil–water mixture containing LGG and curcumin was also prepared using the same method without the addition of β-lgPPs. As shown in Fig. 6A, most deaths of LGG cells in the control sample occurred during the SGF incubation, which might be ascribed to the relatively low tolerance of LGG against gastric acid. Only 3.61 log CFU mL−1 of LGG cells were metabolically viable in the SIF juice when added as a simple oil–water mixture, whereas up to 7.02 log CFU mL−1 of viable LGG could be detected in the digestive juice after SGF and SIF exposure of the HIPE, revealing its protective effect on the LGG cells during in vitro digestion. The release rate of curcumin in the HIPE and control sample during SGF and SIF digestion was also determined and the results were presented in Fig. 6B. It could be found that the curcumin was rapidly released from a simple oil–water mixture in the first 30 min and most Cur was released in the SGF (0–60 min). However, a low release rate of curcumin was observed for the HIPE during 0–60 min, revealing that the encapsulation of curcumin in HIPEs contributed to retard its release in the SGF stage. However, the curcumin was released continuously with a relatively low release rate when the HIPE was transferred from the SGF to the SIF. It was also noteworthy that the cumulative release of curcumin (68.2%) for the HIPE approximated that of the control sample (71.3%), indicating that the encapsulation of curcumin had no adverse impact on the release of curcumin in SGF and SIF.
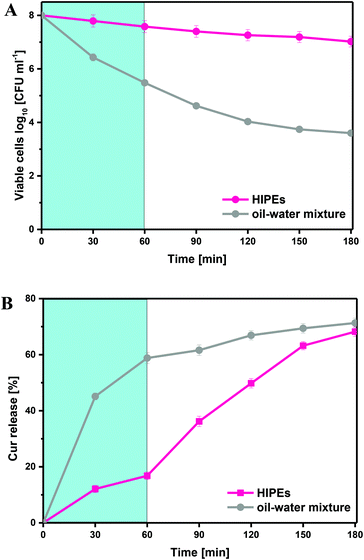 |
| Fig. 6 Viable cell counts in log CFU mL−1 (A) and release of curcumin (B) in a HIPE stabilized by 1.0 wt% β-lgPPs and an oil–water mixture during simulated gastrointestinal digestion as a function of time. | |
4. Conclusion
In conclusion, β-lgPPs with proper size and near-neutral wettability were utilized as particulate emulsifiers to fabricate stable HIPEs. The CLSM images fully confirmed the adsorption of the β-lgPPs onto the oil/water interface, which formed a thick interfacial layer around the droplets and hindered the coalescence of the droplets. Increasing the particle concentration resulted in a decrease in droplet size, and HIPEs at higher particle concentration had a higher gel strength and physical stability during centrifugation. Moreover, HIPEs stabilized by a higher concentration of β-lgPPs exhibited a significantly higher physical stability as well as better protecting effects on LGG cells and curcumin against pasteurization treatment. In comparison with an oil–water mixture, HIPEs also significantly improved the survival rate of LGG cells as well as the curcumin bioaccessibility during in vitro simulated gastrointestinal digestion. These findings provide the possibility for the application of HIPEs for the co-encapsulation of hydrophobic functional components and probiotics that demonstrate synergistic effects in real food products.
Abbreviations
HIPEs | High internal-phase emulsions |
β-lgPPs | β-Lactoglobulin-propylene glycol alginate composite nanoparticles |
LGG |
Lactobacillus rhamnosus GG |
MSD | Mean square displacement |
MVI | Macroscopic viscosity index |
EI | Elasticity index |
SLB | Solid–liquid balance |
FD | Flocculation degree |
CD | Coalescence degree |
GIT | Gastrointestinal tract |
SGF | Simulated gastric fluid |
SIF | Simulated intestinal fluid |
CLSM | Confocal laser scanning microscopy |
SEM | Scanning electron microscopy |
Conflicts of interest
There are no conflicts to declare.
Acknowledgements
This work was supported by the National Key R&D Program of China [2016YFD0400804] and the National Natural Science Foundation of China [No.31371836]. We also highly appreciate the testing support from Beijing Zhongkebaice Technology Service Co., Ltd as well as the China Scholarship Council for Jiaqi Su.
References
- N. Ishibashi and S. Shimamura, Bifidobacteria: research and development in Japan, Food Technol., 1993, 47(6), 126–136 Search PubMed
.
- R. K. Pundir, S. Rana, N. Kashyap and A. Kaur, Probiotic potential of lactic acid bacteria isolated from food samples: an in vitro study, J. Appl. Pharm. Sci., 2013, 85–93 Search PubMed
.
- G. R. Gibson, H. M. Probert, J. V. Loo, R. A. Rastall and M. B. Roberfroid, Dietary modulation of the human colonic microbiota: updating the concept of prebiotics, Nutr. Res. Rev., 2004, 17, 259–275 CrossRef CAS
.
- R. Rieder, P. J. Wisniewski, B. L. Alderman and S. C. Campbell, Microbes and mental health: A review, Brain, Behav., Immun., 2017, 66, 9–17 CrossRef CAS
.
-
FAO/WHO, Joint FAO/WHO (Food and Agriculture Organization/World Health Organization) Working Group Report on Drafting Guidelines for the Evaluation of Probiotics in Food. Guidelines for the Evaluation of Probiotics in Food. Joint Working Group Report on Drafting, London, Ontario, 2002 Search PubMed
.
- L. d. S. Simões, D. A. Madalena, A. C. Pinheiro, J. A. Teixeira, A. A. Vicente and Ó.L Ramos, Micro- and nano bio-based delivery systems for food applications: In vitro behavior, Adv. Colloid Interface Sci., 2017, 243, 23–45 CrossRef
.
- A. Alehosseini, E. M. G. del Pulgar, M. J. Fabra, L. G. Gómez-Mascaraque, A. Benítez-Páez, M. Sarabi-Jamab, B. Ghorani and A. Lopez-Rubio, Agarose-based freeze-dried capsules prepared by the oil-induced biphasic hydrogel particle formation approach for the protection of sensitive probiotic bacteria, Food Hydrocolloids, 2019, 87, 487–496 CrossRef CAS
.
- E. Ananta, M. Volkert and D. Knorr, Cellular injuries and storage stability of spray-dried Lactobacillus rhamnosus GG, Int. Dairy J., 2005, 15, 399–409 CrossRef CAS
.
- T. Riaz, M. W. Iqbal, M. Saeed, I. Yasmin, H. A. M. Hassanin, S. Mahmood and A. Rehman, In vitro survival of Bifidobacterium bifidum microencapsulated in zein-coated alginate hydrogel microbeads, J. Microencapsulation, 2019, 36, 192–203 CrossRef CAS
.
- P. Singh, B. Medronho, M. G. Miguel and J. Esquena, On the encapsulation and viability of probiotic bacteria in edible carboxymethyl cellulose-gelatin water-in-water emulsions, Food Hydrocolloids, 2018, 75, 41–50 CrossRef CAS
.
- T. Esatbeyoglu, P. Huebbe, I. M. Ernst, D. Chin, A. E. Wagner and G. Rimbach, Curcumin–from molecule to biological function, Angew. Chem., Int. Ed., 2012, 51, 5308–5332 CrossRef CAS
.
- P. Forsythe, W. A. Kunze and J. Bienenstock, On communication between gut microbes and the brain, Curr. Opin. Gastroenterol., 2012, 28, 557–562 CrossRef
.
- W. Zam, Gut Microbiota as a Prospective Therapeutic Target for Curcumin: A Review of Mutual Influence, J. Nutr. Metab., 2018, 2018, 1–11 CrossRef
.
- C. T. Peterson, A. R. Vaughn, V. Sharma, D. Chopra, P. J. Mills, S. N. Peterson and R. K. Sivamani, Effects of Turmeric and Curcumin Dietary Supplementation on Human Gut Microbiota: A Double-Blind, Randomized, Placebo-Controlled Pilot Study, J. Evidence-Based Integr. Med., 2018, 23, 1–8 Search PubMed
.
- M. Clapp, N. Aurora, L. Herrera, M. Bhatia, E. Wilen and S. Wakefield, Gut microbiota’s effect on mental health: the gut-brain axis, Clin. Pract., 2017, 7, 131–136 Search PubMed
.
- B. Maha, Antidiabetic Potential of Turmeric with/without Fermented Milk Enriched with Probiotics in Diabetic Rats, Am. J. Biomed. Life Sci., 2013, 1, 1 CrossRef CAS
.
- R. Yan, C. T. Ho and X. Zhang, Interaction Between Tea Polyphenols and Intestinal Microbiota in Host Metabolic Diseases from the Perspective of the Gut-Brain Axis, Mol. Nutr. Food Res., 2020, 2000187 CrossRef CAS
.
- J. Su, X. Wang, W. Li, L. Chen, X. Zeng, Q. Huang and B. Hu, Enhancing the Viability
of Lactobacillus plantarum as Probiotics through Encapsulation with High Internal Phase Emulsions Stabilized with Whey Protein Isolate Microgels, J. Agric. Food Chem., 2018, 66, 12335–12343 CrossRef CAS
.
- Y.-T. Xu, C.-H. Tang and B. P. Binks, High internal phase emulsions stabilized solely by a globular protein glycated to form soft particles, Food Hydrocolloids, 2020, 98, 105254 CrossRef CAS
.
- D. Wu, F. Xu, B. Sun, R. Fu, H. He and K. Matyjaszewski, Design and Preparation of Porous Polymers, Chem. Rev., 2012, 112, 3959–4015 CrossRef CAS
.
- I. Tavernier, W. Wijaya, P. Van der Meeren, K. Dewettinck and A. R. Patel, Food-grade particles for emulsion stabilization, Trends Food Sci. Technol., 2016, 50, 159–174 CrossRef CAS
.
- C. C. Berton-Carabin and K. Schroen, Pickering emulsions for food applications: background, trends, and challenges, Annu. Rev. Food Sci. Technol., 2015, 6, 263–297 CrossRef CAS
.
- J. Su, Q. Guo, S. Yang, H. Li, L. Mao, Y. Gao and F. Yuan, Electrostatic deposition of polysaccharide onto soft protein colloidal particles: Enhanced rigidity and potential application as Pickering emulsifiers, Food Hydrocolloids, 2021, 110, 106147 CrossRef CAS
.
- J. A. Hawrelak, D. L. Whitten and S. P. Myers, Is Lactobacillus rhamnosus GG effective in preventing the onset of antibiotic-associated diarrhoea: a systematic review, Digestion, 2005, 72, 51–56 Search PubMed
.
- L. J. Wang, Y. Q. Hu, S. W. Yin, X. Q. Yang, F. R. Lai and S. Q. Wang, Fabrication and characterization of antioxidant pickering emulsions stabilized by zein/chitosan complex particles (ZCPs), J. Agric. Food Chem., 2015, 63, 2514–2524 CrossRef CAS
.
- F. Liu and C. H. Tang, Phytosterol colloidal particles as Pickering stabilizers for emulsions, J. Agric. Food Chem., 2014, 62, 5133–5141 CrossRef CAS
.
- J. Su, Q. Guo, Y. Chen, W. Dong, L. Mao, Y. Gao and F. Yuan, Characterization and formation mechanism of lutein pickering emulsion gels stabilized by β-lactoglobulin-gum arabic composite colloidal nanoparticles, Food Hydrocolloids, 2020, 98, 105276 CrossRef CAS
.
- X. Wang, X. Li, D. Xu, Y. Zhu, Y. Cao, J. Wang and B. Sun, Comparision of heteroaggregation, layer-by-layer and directly mixing techniques on the physical properties and in vitro digestion of emulsions, Food Hydrocolloids, 2019, 95, 228–237 CrossRef CAS
.
- B. R. Shah, Y. Li, W. Jin, Y. An, L. He, Z. Li, W. Xu and B. Li, Preparation and optimization of Pickering emulsion stabilized by chitosan-tripolyphosphate nanoparticles for curcumin encapsulation, Food Hydrocolloids, 2016, 52, 369–377 CrossRef CAS
.
- F. Liu, C. Ma, R. Zhang, Y. Gao and D. J. McClements, Controlling the potential gastrointestinal fate of beta-carotene emulsions using interfacial engineering: Impact of coating lipid droplets with polyphenol-protein-carbohydrate conjugate, Food Chem., 2017, 221, 395–403 CrossRef CAS
.
- L. Donato, C. Schmitt, L. Bovetto and M. Rouvet, Mechanism of formation of stable heat-induced β-lactoglobulin microgels, Int. Dairy J., 2009, 19, 295–306 CrossRef CAS
.
- M. Audebrand, M.-H. Ropers and A. Riaublanc, Disappearance of intermolecular beta-sheets upon adsorption of beta-lactoglobulin aggregates at the oil–water interfaces of emulsions, Food Hydrocolloids, 2013, 33, 178–185 CrossRef CAS
.
- Z. Wei and Q. Huang, Edible Pickering emulsions stabilized by ovotransferrin–gum arabic particles, Food Hydrocolloids, 2019, 89, 590–601 CrossRef CAS
.
- J. Xiao, Y. Li and Q. Huang, Recent advances on food-grade particles stabilized Pickering emulsions: Fabrication, characterization and research trends, Trends Food Sci. Technol., 2016, 55, 48–60 CrossRef CAS
.
- J. Xiao, X. a. Wang, A. J. P. Gonzalez and Q. Huang, Kafirin nanoparticles-stabilized Pickering emulsions:
Microstructure and rheological behavior, Food Hydrocolloids, 2016, 54, 30–39 CrossRef CAS
.
- Z. Wei, Y. Cheng and Q. Huang, Heteroprotein complex formation of ovotransferrin and lysozyme: Fabrication of food-grade particles to stabilize Pickering emulsions, Food Hydrocolloids, 2019, 96, 190–200 CrossRef CAS
.
- L. J. Wang, Y. Q. Hu, S. W. Yin, X. Q. Yang, F. R. Lai and S. Q. Wang, Fabrication and characterization of antioxidant pickering emulsions stabilized by zein/chitosan complex particles (ZCPs), J. Agric. Food Chem., 2015, 63(9), 2514–2524 CrossRef CAS
.
- T. Narita, K. Mayumi, G. Ducouret and P. Hébraud, Viscoelastic Properties of Poly(vinyl alcohol) Hydrogels Having Permanent and Transient Cross-Links Studied by Microrheology, Classical Rheometry, and Dynamic Light Scattering, Macromolecules, 2013, 46, 4174–4183 CrossRef CAS
.
- M. Corredig and M. Alexander, Food emulsions studied by DWS: recent advances, Trends Food Sci. Technol., 2008, 19, 67–75 CrossRef CAS
.
- G. Petzold, C. Goltzsche, M. Mende, S. Schwarz and W. Jaeger, Monitoring the stability of nanosized silica dispersions in presence of polycations by a novel centrifugal sedimentation method, J. Appl. Polym. Sci., 2009, 114, 696–704 CrossRef CAS
.
- Y. Zhang, J. Lin and Q. Zhong, The increased viability of probiotic Lactobacillus salivarius NRRL B-30514 encapsulated in emulsions with multiple lipid-protein-pectin layers, Food Res. Int., 2015, 71, 9–15 CrossRef CAS
.
- C.-H. Tang and F. Liu, Cold, gel-like soy protein emulsions by microfluidization: Emulsion characteristics, rheological and microstructural properties, and gelling mechanism, Food Hydrocolloids, 2013, 30, 61–72 CrossRef CAS
.
|
This journal is © The Royal Society of Chemistry 2021 |