DOI:
10.1039/D2RE00521B
(Review Article)
React. Chem. Eng., 2024,
9, 10-25
Batch and continuous flow mechanochemical synthesis of organic compounds including APIs
Received
25th November 2022
, Accepted 21st August 2023
First published on 5th September 2023
Abstract
Mechanochemistry is becoming an enabling technology for the synthesis of organic and inorganic compounds as well as for the synthesis of polymers as it underlines sustainability in a significant manner. Continuous mechanochemical synthesis further adds value to the approach through consistency, smaller footprint, and better energy efficiency. This review gives an indepth view of the present status of this subject along with critical engineering aspects that one needs to measure and monitor as eventually synthesis needs to be transformed into a process. The examples covered herein include the synthesis of organic compounds, viz., APIs, agrochemical intermediates, catalysts, and polymers. In the end, we also discuss the safety aspects of mechanochemical synthesis and recommendations for exploring this field further.
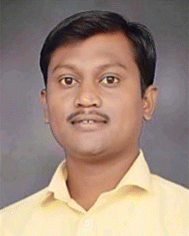 Ranjit Atapalkar | Mr. Ranjit Shabu Atapalkar received his B.Sc. (Shivaji University, Kolhapur) and M.Sc. (Organic Chemistry) from PAH Solapur University, Solapur, India with first class in 2014. He cleared the CSIR-NET exam with (AIR) All India Rank 62 in 2016 and was awarded a Junior as well as Senior Research Fellowship by the CSIR, New Delhi. He is currently pursuing his Ph.D. at CSIR-National Chemical Laboratory, Pune. His research is mainly focused on solid phase flow synthesis, mechanochemistry, flow synthesis of large organic molecules and continuous anionic polymerization and multi-step Synthesis of Active Pharmaceuticals Ingredients. |
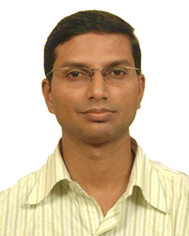 Amol A. Kulkarni | Dr. Amol A. Kulkarni is a Chief Scientist in the Chemical Engineering & Process Development Division at the CSIR-National Chemical Laboratory (NCL), Pune, India. He is a chemical engineer by training and did Ph.D. in chemical engineering (2003) from the Institute of Chemical Technology ICT- Mumbai. He did his Post-Doctorate at the Max Planck Institute-Magdeburg (Germany) (2004) and at Massachusetts Institute of Technology, USA (2010). He works in the area of design and development of continuous flow reactors and explores their applications for continuous syntheses of pharmaceutical intermediates, dyes, agrochemicals, perfumery chemicals and nanomaterials. He has developed several scalable continuous processes for important organic compounds and inorganic functional materials including many solvent free synthesis protocols. He also works on experimental and numerical analysis of multiphase fluid dynamics in presence of diffusion and reaction. |
1. Introduction
Mechanochemistry is defined as the reaction induced by the inputs of mechanical energy by impacts within vibratory and planetary ball mill. Recently, IUPAC has declared mechanochemistry as one of the 10 world-changing chemistry innovations.1 The mechanochemistry concept was developed by Faraday, Ostwald, and Spring and Lea in 1820, which was further expanded in inorganic chemistry, organic chemistry, minerals, polymer chemistry, nanoparticles, metal organic frameworks, catalysts, etc.2 Although the field finds more applications in the area of materials science, organic synthesis, polymer synthesis, polymer processing, and food industries, exploring continuous mechanochemistry using screw and twin screw reactors for greener, economical, and fast synthesis has become an important milestone toward sustainability. Over the last few decades, developing a solid–solid reaction approach for the chemical synthesis and transformation initiated by mechanical force has been known more in mortar–pestle and grinders for simple chemistries and less in the synthesis of complex molecules. Mechanochemistry offers new opportunities for making new materials and molecules in a solvent-free or with minimal solvent domain, making synthesis more sustainable.3 Mechanochemical processes have been recently explored for making drug molecules, agrochemicals, dyes, and intermediates.4,5
The mere contact of two reactants does not always lead to a chemical reaction. The reactants have to be brought to a specific range of conditions that allow necessary activation energy to be provided by several means, viz., light, heat, pressure, electricity, and/or mechanical force. Mechanochemistry makes it happen through mechanical forces in the form of shear, deformation, and impact, increase in the reactivity of solid reactants by creating crystal defects, transition states, or by direct bond breakage. This implies that wherever possible, it is possible to make solid reactants react directly in the absence of a solvent or with minimal solvent (that just helps making a thick paste of reactants), thereby eliminating the need for the energy-intensive processes of solvent handling, recovery, purification, and reuse. Conventionally, a batch process needs large quantity of solvents when substrates undergoing reactions are solids, which facilitates stirring for mixing, heat transfer, absorbing certain heat, and easy charging and discharging of reactors. However, it is an overall expensive operation as using solvents slows down the reactions due to lower concentrations; one needs to have large inventory of solvents, which increases the overall plant footprint. Eliminating or minimizing solvent quantities will greatly reduce the reactor volume. These issues strongly support going for mechanochemistry and more for a continuous version of it.
Mechanochemistry is emerging rapidly to perform organic reactions in the absence of solvent or minimization of solvent quantity,3,4,6–8 especially for different organic syntheses9,10 and for some reactions involving biomass,5,11–13 advanced application of OLED material,14 and solvent-free asymmetric organo-catalysis.15 Amide synthesis by ball milling is also a well-known method.16,17 Mechanochemistry actually does more efficient and effective transfer energy to the reacting molecules.18–21 Translating green synthetic methodologies to the field of natural products, peptide synthesis, and drug discovery will also facilitate obtaining active pharmaceutical ingredients (APIs), final drug molecules, and in general, commodity chemicals in a sustainable manner.22 Very recently, Browne and co-workers reported the direct amidation of esters in a ball mill in a relatively short time.23 Using a ball mill is a known and traditional method for bringing solids in contact with each other. Large scale ball mills are found in minerals and mining industries, where the objective of the process is only size reduction with no reactions or heat generation, which poses a significant challenge for scale up of the reaction in a ball mill. On the other hand, a large number of syntheses have been recently reported using continuous mechanochemistry.24 These reports show that in contrast to using scalar quantities such as pressure and temperature to control a chemical reaction, the vectorial character of the mechanical force can be used to guide the reaction in a certain desired pathway by selective bond fission, leading to molecular configurations not obtainable by commonly used thermal and photochemical means. Primarily, it facilitates the reaction at intrinsic kinetics with the only limitation of solid–solid interaction and surface scavenging, which, to a certain extent, is overcome by the mechanical energy.
1.1 History
The history of mechanochemistry is divided into three main era's, viz., discovery period, development period, and understanding and utilization period.2 Faraday reported the reduction reaction of silver chloride by grinding with different metals such as zinc, tin, copper, and iron in a mortar method in 1820.25,26 Ostwald reported the chemical effects of mechanical energy.27 In 1919, Ostwald published a mechanochemistry textbook together with electrochemistry, thermochemistry, and photochemistry, details of which are cited in excellent Review papers by Takacs.28,29 Spring and Carey reported mechanochemical reactions in 1886.30 However, when it comes to continuous mechanochemistry, the adaptation of well-known tools viz. screw extruders (single or twin screws), while excellent early stage developments can be seen in the literature, it is still a very early stage and we have elaborated the challenges towards the end of this manuscript.
Important terminologies.
There are specific terminologies that one needs to use when referring or exploring mechanochemistry. These include the following.
1. Mechanochemistry: the chemical reaction induced by input of mechanical energy by impacts or vibrations or shear in a confined zone.
2. Grinding is a process of breaking up particles that results in fine powder.
3. Liquid-assisted grinding requires some amount of liquid as an additive to increase the contact of the solids and to improve the reactivity of the material or to avoid particle surface decomposition due to inertial temperature rise due to impact/mechanical force.
4. Ball milling is a grinding technique where a cylinder is filled with a mixture of reactants and balls, which provide impact force upon constant rotation or vibration. Many organic reactions can be run in solvent-free conditions using a ball mill. The impact of the balls generates high local temperature up to the 100 °C for a short time during impact (i.e., few ms) and thus imparts necessary energy for reactions.
5. Continuous mechanochemistry: is the process where external mechanical energy is used for mixing, shearing, unidirectional movement of solids or thick slurries or viscous liquids continuously fed by a single screw (SSC) or twin screw, and it is also known as reactive extrusion.
2. Requirement and criteria for batch and continuous mechanochemistry
2.1 The mechanical force and its interaction with solids
The energy supplied to the solid particles partly contributes to motion, and the rest is supplied in the form of shear and compression and/or deformation energy. If the stress due to shear is more than the yield strength of the material, the solid breaks, and some of the stored deformation energy is used to make a new surface. The rest of the energy settles as frictional heat, defect formation energy (in metals and ionic solids, etc.), bond transition, and/or breakage energy in covalent solids. Mechanical energy alters the chemical nature of a substance differently compared to heat and does not need to be converted to heat to be a mode of activation; it can directly cause a change in the electron distribution and thereby a deviation from the stable ground state. The total mechanical energy supplied to the solid decides the degree of activation and hence the reaction. In certain cases, charge transfer gets induced by mechanical action between the driving surface (balls, pins, or screw motion) and the particles or between particles of different densities. As the distribution of mechanical energy given to a mixture of particles is not even, efficient mixing is necessary to homogenize the powders/granules, which is easily possible if the vibration frequency and intensity is optimized for an impact or vibration system and if the rotation speed and design of all the elements of a screw are designed properly.
2.2 Advantage of solvent-free environment
Mechanochemical reactions offer improved reactivity and selectivity. Going beyond the Arrhenius criteria that allows the quantitative analysis of the rate of reaction, when compared with the solution phase, solid phase mechanochemical reaction rates are expected to be higher due to the higher concentration of reaction mass.31,32 More importantly, while in many reactions solvents play a significant role of bringing the dissolved species in close contact with each other, facilitate reactions by taking an active part to create solvation dynamics-based complexes (viz. Grignard reaction), or safety (using sulphuric acid in aromatic nitration to absorb the water that gets generated and also to catalyze the generation of nitronium ions), etc., however, there is never a compulsory reason to have solvents for every reaction. This makes a synthesis rapid, with smaller footprint, efficient in terms of energy usage, and thus aligned with the Green Chemistry principles (Fig. 1).
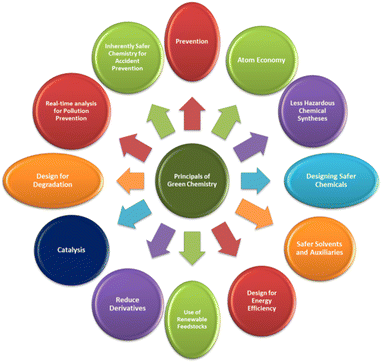 |
| Fig. 1 Twelve principles of green chemistry.33 | |
2.3 Methods/equipment
Mechanochemistry has been traditionally done by mortar and pestle method (hand grinding), vibratory and planetary ball milling (impact), attrition ball mall, liquid assisted hand grinding, and ionic liquid-assisted hand grinding of various types in the batch mode. The screw extruder, single screw drilling, and twin screw extruder is used as a reactor for continuous flow processing, as shown in Fig. 2.33
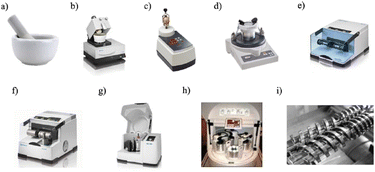 |
| Fig. 2 Common grinding and milling apparatus: (a) Mortar and pestle. (b) Automatic mortar (Retsch). (c) Vertical vibrational mini-mill (Fritsch). (d) Vibratory micro-mill (Fritsch). (e) Vibrational ball-milling (Retsch). (f) Vibrational ball-milling with temperature control (Retsch, Cryomill). (g) Planetary ball-milling (Retsch). (h) Multi-sample milling (Automaxion). (i) Twin-screw used for continuous mechanochemical processes. Adapted with permission from ref. 33. Copyright 2020, American Chemical Society. | |
2.4 Application and advantages of mechanochemistry
The efficiency of mechanochemical methods in pharmaceutical developments is worthy due to the solvent-free nature or low solvent of such methods, compatible with the needs of academia and modern industry. The similar observation of pharmaceutical salt or the co-former in a pharmaceutical co-crystal used a mechanochemical approach.34,35 Mechanochemistry has different applications in the field of organic synthesis, inorganic synthesis, catalysis, reactive intermediates, extraction, waste management, biomass valorization, hydrometallurgy, nanoparticle synthesis, polymer synthesis, pharmaceutical industry, food and cosmetic, and many more.36 The great advantages of mechanochemical procedures compared to solution-based methods are milder reaction conditions, high yield and high throughput, higher selectivity, shorter reaction time, less energy required, less side product formation, and no need of purification by column chromatography.8 The comparison between solution-based and mechanochemical methods37 is shown in Fig. 3 below.
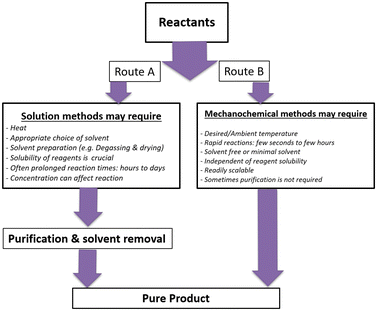 |
| Fig. 3 Comparative studies of solvent-based and mechanochemical methodologies via route A and B, respectively. | |
3. Batch mechanochemistry of organic compounds including APIs
Mechanochemistry can be implemented for almost all sorts of organic compounds and APIs.
3.1 Organic compounds by mechanochemistry (ball milling)
In 2009, Lamaty and co-workers reported a solvent-free peptide synthesis by the mechanochemical approach.38 Compound (1) and phenylalanine (2) were treated with NaHCO3 for 1 h reaction time to obtain dipeptide compound (3), as shown in Scheme 1. They have reported 17 examples with mild to good yield. Furthermore, the authors have extended this protocol to the synthesis of aspartame with 84% yield.
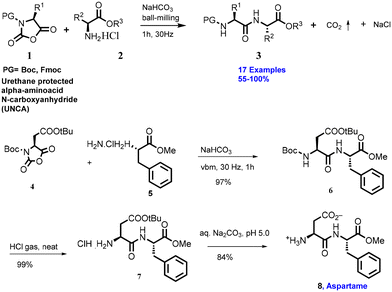 |
| Scheme 1 Peptide and aspartame synthesis by the mechanochemical route. | |
Aspartame synthesis.
In 2020, Dalidovich et al., reported the synthesis of amides by uranium-based coupling reagents by the mechanochemical approch.39 Preparation of amide (11) uses a carboxylic acid (9) and amines (10), coupling of (1-cyano-2-ethoxy-2-oxoethylidenaminooxy)-dimethyl-aminomorpholinocarbeniumhexafluorophosphate (I) (COMU) or N,N′,N′-tetramethylchloroformamidinium hexafluorophosphate (II) (TCFH) using K2HPO4 as a base, as shown in Scheme 2.
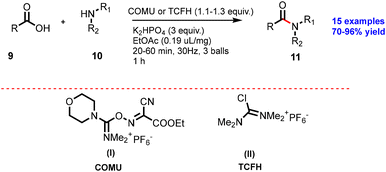 |
| Scheme 2 Direct acid amidation by the mechanochemical approach. | |
They have reported the synthesis several of amide derivatives (15 examples) in good yield (70–96%) with fast reaction rates within 1 h. This synthesis strategy is a solvent-free, atom-economic, and mechanochemically-activated reaction, which makes is more sustainable.
In 2016, Browne and co-workers reported the selective mechanochemical fluorination of 1,3-dicarbonyl compounds using Selectfluor.40 The diketone compound (12) reacted with Selectflour (I) in the presence of 10% ACN solvent without using base, and monofluorinated compound (13) was achieved in excellent (100%) yield. Contacting the diketone compound (12) with Selectflour (I) in the absence of ACN solvent and using Na2CO3 as a base achieved difluorinated compound (14) in good yield as shown in Scheme 3. Importantly, the reduction of reaction time (2 h) via the mechanochemical approach is significant as compared to traditional solution-phase batch chemistry (24 h). The mechanochemical fluorination reaction increased the selectivity of the reaction with excellent yield within a shorter reaction time, as shown in Table 1.
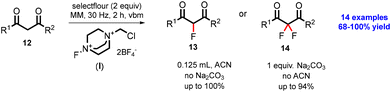 |
| Scheme 3 Fluorination reaction by the mechanochemical approach using Selectfluor. | |
Table 1 Conventional and mechanochemical electrophilic fluorination of 1,3-diketones (12)
Solvent based method for 3.5 h |
Solvent based method for 24 h |
Mechanochemical reaction for 2 h |
Mechanochemical reaction with LAGsa for 2 h |
Liquid assisted grindings (LAGs): decreased reaction time and increased selectivity.
|
Stirring in solution |
Stirring in solution |
Solid state grinding |
Solid state grinding |
Selectfluor, 2 equiv. |
Selectfluor, 2 equiv. |
Selectfluor, 2 equiv. |
Selectfluor, 2 equiv. |
— |
Na2CO3, 1 equiv. |
Na2CO3, 1 equiv. |
Milled 30 Hz |
20 mL ACN |
20 mL ACN |
|
R.T. |
R.T. |
R.T. |
R.T. |
With 10% ACN |
Without ACN |
88% yield |
93% yield |
93% |
98% |
95% |
13 : 14 |
13 : 14 |
13 : 14 |
13 : 14 |
13 : 14 |
>50 : 1 |
1 : 7 |
1 : 17 |
50 : 1 |
1.5 : 1 |
Colacino et al. have developed a synthesis of N,O-dibenzylcarbamate under solvent-free conditions.41 Benzyl alcohol (15) was treated with 2 equiv. 1,1-Carbonyldiimidazole (CDI) at room temperature, which could be used to synthesize compound (16) within 15 min reaction time by a vibratory ball mill, which upon further treatment with benzylamine (17) using K2CO3 as a base, gives 18 in good to excellent yields (Scheme 4). Authors have reported 23 examples with excellent yield (73–99%) without further purification.
 |
| Scheme 4 Synthesis of N,O-dibenzylcarbamate (18) under solvent-free conditions. | |
Ranu and co-workers have reported the solvent-free synthesis of coumarine via Pechmann condensation,42 the reaction of phenol derivatives (19) treated with beta-ketoester (20) using a ball mill at room temperature in the presence of stoichiometric MsOH (0.1 equiv.) as a mild acid catalyst to generated coumarine and its derivatives (21) without purification by column chromatography, as shown in Scheme 5. The approach ensures to follow most of the green chemistry aspects for 42 examples with good regioselectivity, solvent-free conditions, mild reaction, and energy saving.
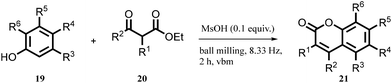 |
| Scheme 5 Solvent-free synthesis of coumarine and its derivatives. | |
In 2013, the synthesis of N-protected amino acids with less solvent by the milling methodology was reported by Konnert et al.43 Amino acid (22), K2CO3, and NaCl were added in a 12 mL stainless jar and the reaction medium was ground for 2 h with further addition of Boc2O and grinding for 3 h to achieve the final protected amino acid (23) in powder form, as shown in Scheme 6. The approach was further extended for 29 substrates with moderate to good yield in 50–95% yield in a short reaction time.
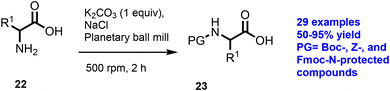 |
| Scheme 6
Tert-butyloxycarbonyl N-protected amino acids. | |
Heterocyclic compounds.
Many heterocycles are synthesized by the LAGs method with less reaction time. Kumar and co-workers developed a LAGs-promoted method for the preparation of arylideaminoquinazolin-(1H)-one derivatives for solid aldehyde and ketone synthons.44 In this protocol, few drops of DMSO were added to the reaction medium, followed by reaction of 24 with aldehyde/ketone (25) to afford quinazolinone product (26) in Scheme 7 with 96% yield in only 2–5 min. 38 derivatives were reported with excellent yield in a short reaction time. Banerjee and co-workers45 have described the condensation reaction of 1,2-diamines (27) and 1,2-carbonyl (28) to obtain quinoxaline (29) product (Scheme 7). The authors have showed wide range of substrate scope (30 examples) with excellent yield in a short reaction time of 10–30 min. Using LAGs-accelerated protocol, Sharma and co-workers have reported multicomponent reactions. The novel synthesis of pyranochromenones with multicomponent cascade reactions has been reported,46 wherein aldehyde (30) and malononitrile (31) were subjected to naptha-coumarine compound (32) in the presence of solid DABACO as a base-catalyzed reaction, affording pyranochromenones (33) with excellent yield (72–91%) within a short time. They reported 11 examples, as shown in Scheme 7.
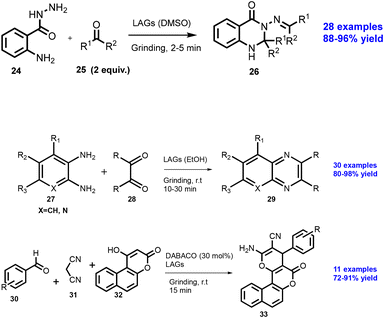 |
| Scheme 7 Synthesis of heterocycles by LAGs mechanochemistry. | |
3.2 Active pharmaceutical ingredients (APIs) by mechanochemistry
In 2012, Lamaty and co-workers developed the synthesis of teriflunomide.47 They reported amide synthesis (21 examples) via the mechanochemical approach. Teriflunomide (36) was synthesized in two steps using 5-methyl-isoxazolecarboxyl acid (34), carbonyldiimidazole, and aniline hydrochloride (35). The product was hydrolyzed with aq. HCl solution for 24 h with good yield (81%) (Scheme 8). The Colacino group described the preparation of hydantoin-based APIs as a phenytoin48 by the mechanochemical approach via the condensation reaction of diphenylglycine methyl ester. HCl (37) was treated with tetramethyl silyl isocynate over 3 h to obtain compound (38) in 84% yields, as shown in Scheme 9. Furthermore, in extensions of this work in a similar manner by the Colacino group in 2016,49 the starting amino methyl ester (39) was reacted with CDI using a ball mill at 450–750 rpm to give an intermediate, which when reacted with ethyl amine hydrochloride (1 equiv.) and potassium carbonate (3 equiv.) for 60–120 min gave intermediate (II). This intermediate was in situ cyclized to afford 3-ethyl-5-benzyl hydantoin (40) in (84%) good yield (Scheme 10). Colacino et al. have further reported the mechanochemical synthesis of nitrofurantoin and dantrolene.50 1-Aminohydantoin·HCl (41) was treated with aldehyde (42) in the absence of base and solvent to afford compound (43), selectively the E-isomer, and high yield (87–98%) with 10 examples within 10–15 min. Furthermore, they have extended their methodology for the synthesis of nitrofurantoin (44) and dantrolene (45), achieving an excellent yield (95% and 90%, respectively) after 2 h milling using a ball mill (Scheme 11).
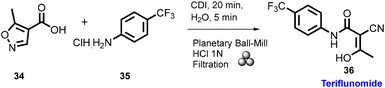 |
| Scheme 8 Preparation of teriflunomide. | |
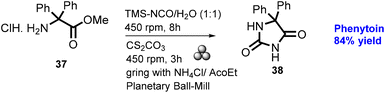 |
| Scheme 9 Synthesis of active pharmacological phenytoin by the mechanochemical approach. | |
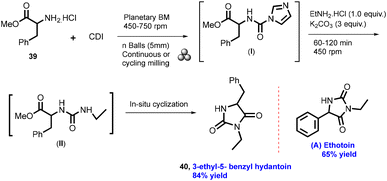 |
| Scheme 10 Mechanochemical synthesis of 3-ethyl-5-benzyl hydantoin. | |
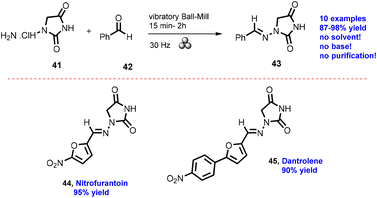 |
| Scheme 11 Synthesis of nitrofurantoin and dantrolene under solvent-free conditions. | |
Friscic, et al. have reported a mechanical synthesis of tolbutamide and chlorpropamide under solvent-free conditions.51 In this strategy, 5 mol% copper catalysts were employed for the reaction between arylsufonamide (46) treated with isocynates (47) to afford tolbutamide (48) and chlorpropamide (49). The tolbutamide and chlorpropamide was synthesized with excellent yield of 90–92% of the desired product in 2 h using 5 mol% CuCl catalysts (Scheme 12). A similar protocol was employed for the synthesis of glibenclamide, where substituted benzoic acid (50) was reacted with arylsulfonamides (51) and EDC·HCl in pyridine and ground for 1 h to afford condensation product (52), which was further treated with cyclohexylisocyanate (53) and 5 mol% CuCl as a catalyst to obtain glibenclamide (54) with 70% overall yield (Scheme 13). The comparative studies of the solution-based and mechanochemical methods for the synthesis of glibenclamide are given below. The conventional synthesis pathways for glibenclamide used high boiling and bulk amount of solvent (toluene, DCE, DCM, DMF, etc.), room to refluxing temperature, and long reaction time (>2 h) with moderate to good yield (50–98%).
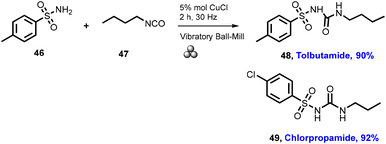 |
| Scheme 12 Synthesis of tolbutamide and chlorpropamide under solvent-free protocol. | |
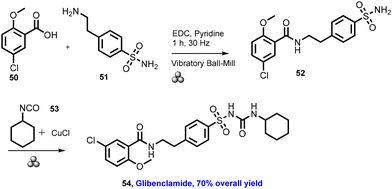 |
| Scheme 13 Synthesis of glibenclamide. | |
Strukil group reported the synthesis of procainamide and paracetamol using the mechanochemical approach52 in 2018. In this protocol, 4-nitrobenzol chloride (55) and N,N-diethylethylenediamine (56) with potassium carbonate as the base and silica as a grinding auxiliary were used to obtain amide (57), which on catalytic transfer hydrogenation using ammonium formate and palladium with MeOH as a LAGs affords procainamide (58). Furthermore, the same strategy was employed for the important bioactive drug paracetamol. 4-Nitrophenol (59), when treated with ammonium formate and palladium in the absence of solvent, affords the desired amine (60), which was further reacted with acetyl acetate (61) to obtain paracetamol (62) (Scheme 14). The comparative studies of the solution-based and mechanochemical methods are given below for the synthesis of procainamide and paracetamol. The conventional pathways were used for the synthesis of procainamide and paracetamol, which used bulk amount of solvents (MeOH, CH3CN, H2O, etc.), room to refluxing temperature, needed multi-step and complex purification step, large excess amount of H source, and >1 h reaction time, providing good to excellent yield (35–100%).
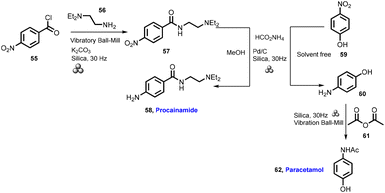 |
| Scheme 14 Procainamide and paracetamol synthesis by mechanochemical force. | |
Liquid-assisted grinding.
In 2016, Jiang et al., reported the synthesis of fenbufen by high-speed ball milling.53 In this method, ethyl-3-(4-chlorobenzoyl)propionate (63) was subjected to phenylboronic acid (64) with palladium acetate catalyst and potassium carbonate (5 equiv.) as a base with MeOH as LAGs to obtained fenbufen ethyl ester (65) in excellent yield (91%) after a long reaction time (Scheme 15). The comparative studies of the solution-based and mechanochemical methods are given below for the synthesis of fenbufen ethyl ester. The synthesis of fenbufen ethyl ester by conventional route used bulk amount of organic solvent (nitrobenzene, DCE, dioxane, etc.), room to refluxing temperature, tedious operation, and >1 h reaction time, giving good yield (60–99%). Various dipine categories of molecules are important as anti-cholesterol drugs and synthesized through multistep batch synthesis. Recently, Ye and co-workers developed54 the synthesis of felodipine under ball-milling conditions. This protocol showed reaction between 2,3-dichlorobenzaldehyde (66), methyl acetoacetate (67), ethyl-3-aminocrotonate (68), and lipozyme as a catalyst with NaCl salt to afford felodipine (69) as a bioactive molecule within 3 h and good yield (68%) by ball milling (1,4-dihydropyridine (1,4-DHP)) (Scheme 16). Zhang et al. reported an environmentally-benign solvent-free synthesis of fenbendazole.55 They showed that the mechanochemical method proceeded with the condensation of o-phenylenediamine (70) and aldehyde in the presence of acetic acid as the catalyst by mortar–pestle in situ cyclization reaction resulted in benzimidazole (71) with high yields (∼85%). Upon subjecting benzimidazole (71) to methyl chloroformate (72) in CS2CO3 as a base, it resulted in fenbendazole (73) with 84% yield (Scheme 17). In 2016, Zhang and co-workers reported an I2-catalyzed amination and cyclization of ketone using 2-aminopyridines under solvent-free conditions. They focused on solvent and metal-free synthesis of antiulcer drug as a zolimidine.56 This work reports the reaction of 1-(4-(methylsulfonyl) phenyl)ethanone (74) with 2-aminopyridines (75) using an I2- and DMAP-catalyzed reaction at room temperature for 90 min to obtain zolimidine (76) in 86% yield (Scheme 18). Very recently, the Browne group developed a new method for the direct amidation of ester by ball milling.23 The authors have described a simple-to-operate procedure requiring an ester, amine, and stoichiometric KOtBu to synthesize a broad library of 78 amides derivatives with good to excellent yield. The methyl or ethyl ester (77) reacted with primary or secondary amine (78) and stoichiometric KOtBu for 1 h to give amide compound (79) in a vibratory ball mill, giving 52–98% yield, as shown in Scheme 19.
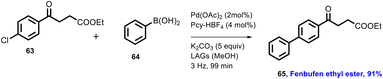 |
| Scheme 15 Synthesis of fenbufen. | |
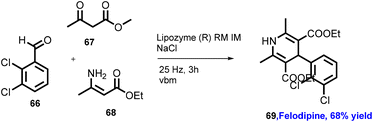 |
| Scheme 16 Mechanoenzymatic synthesis of felodipine. | |
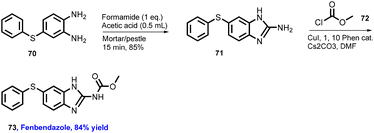 |
| Scheme 17 Solvent-free benzimidazole formation or fenbendazole synthesis. | |
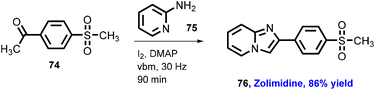 |
| Scheme 18 Solvent-free zolimidine synthesis. | |
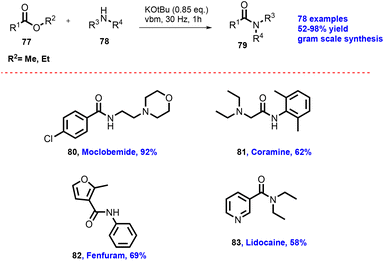 |
| Scheme 19 Direct amidation of ester by ball milling. | |
Browne's group has also explored reactions with broad substrate scope for other APIs including moclobemide (80) with 92% yield (anti-depressant), coramine (81) with 62% yield (stimulant), fenfuram (82) with 69% yield (fungicide), and lastly lidocaine (83) with 58% yield (anaesthetic).
4. Continuous mechanochemistry of organic compounds including APIs
While the batch mode of mechanochemical syntheses is facilitated using vibration or impact kind of ball mills, the real advancement of mechanochemistry is when it is operated in the continuous mode. Continuous mechanochemistry such as in single screw reactor (SSR), twin screw reactor (TSR), and reactive extruder (RE) is useful in the synthesis as well as development of formulations. Reports on several organic compounds synthesized by continuous mechanochemical approaches are summarized as follows.
4.1 Organic compounds by continuous mechanochemistry
In 2019, Kulkarni and co-worker developed a clean, safe, sustainable protocol for the continuous mechanochemical synthesis of organic compounds, such as aldol condensation, oxidation, nucleophilic substitutions, protection, acylation, heterocycle synthesis, and coupling reactions.57 They achieved good yield (52–97%) with short residence time in few seconds (15–300 s) (Scheme 20). Importantly, each reaction was carried out at the 5 g scale. Almost all the reported categories of 9 organic reactions were purified by simple filtration or crystallization, avoiding column chromatography, thus making the process greener and more sustainable. The key features of the synthesis methodology are as follows: (i) 9 different organic reactions, (ii) 52–97% yield, (iii) 15–300 s reaction times, (iv) 5 g scale synthesis, (v) purification by filtration, (vi) 90–450 rpm, (vii) temperature range 0–100 °C, and (viii) 1 kg Sudan dye.
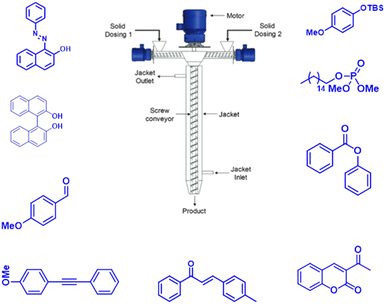 |
| Scheme 20 Continuous mechanochemical synthesis of organic compounds using screw reactor. | |
4.1.1 Scale up of Sudan dyes.
This work was extended for the synthesis of 1 kg Sudan dye in laboratory scale (Fig. 4). The synthesized compound has versatile application in coloring various foodstuffs, curry, and chilli powder, and is especially used to color hydrocarbon solvents, oil, fats, waxes, shoes, and floor polishes. In 2017, James and co-workers developed the synthesis for different types of condensation reactions such as Knoevenagel condensation, imine formation, Michael addition, and aldol condensation reactions with small reaction times of few min and good yield using twin screw extrusion (TSE). Details are shown in Schemes 21–26. Authors have studied58 the reaction of aldehyde (84) with barbituric acid (85) at 160 °C to afford product (86) with good conversion within 90 min. They compared 3 aldehydes: vanillin was treated with barbituric acid to obtain product (87), veratraldehyde required 90 min to obtain product (88), and 5-bromovanillin was treated with barbituric acid to obtain product (89), with 93%, 100%, and 77.3% conversion, respectively (Scheme 21).
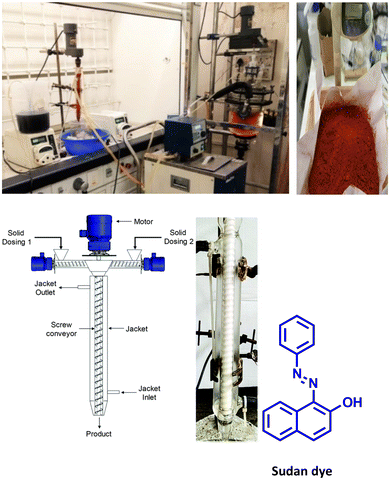 |
| Fig. 4 Setup for continuous synthesis of azodyes on kilogram scale using screw reactor. | |
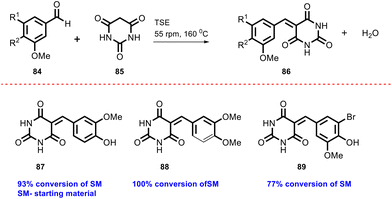 |
| Scheme 21 Continuous mechanochemical synthesis of organic compounds by TSE. | |
 |
| Scheme 22 Knoevenagel condensation under solvent-free conditions. | |
 |
| Scheme 23 Knoevenagel condensation reaction to prepare product (95) by TSE. | |
 |
| Scheme 24 Imine formation by ball milling and TSE. | |
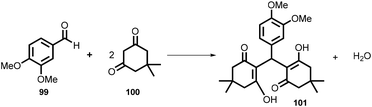 |
| Scheme 25 Michael addition carried out mechanochemically to form product (101) by TSE. | |
 |
| Scheme 26 Aldol condensation reaction to form substituted 1,3-indandione (104) by TSE. | |
The Knoevenagel condensation of vanillin (90) and malononitrile (91) was carried out using TSE at 55 rpm to afford condensed product (92) (Scheme 22). The Knoevenagel condensation reaction of vanillin (93) and ethyl-cyanoacetate (94) using sodium carbonate as a base was also carried out by TSE at 55 rpm to afford condensed product (95) (Scheme 23). The authors have reported the formation of imine by ball milling and TSE method without the addition of solvent. 4,4′-Oxydianiline (96) treated with o-vanillin (97) by ball milling and TSE affords the imine product (98) (Scheme 24). 100% conversion to the final product was observed. The authors reported the reaction of vetraldehyde (99) and dimedone (100) by twin screw extrusion to afford product (101) (Scheme 25). The reaction temperature was 160 °C for 12 min, and almost 100% conversion of the starting materials was observed. Ninhydrin (102) was reacted with dimedone (103) (in volumetric flow rate) by TSE at 55 rpm to obtain a substituted 1,3-indandione (104) (Scheme 26). 100% conversion to the product was observed within 12 min reaction time. These reports strongly support using single screw extrusion (SSE) and twin screw exstrusion (TSE) for the sustainable synthesis of various important organic molecules.
In 2020, Pineiro and co-workers reported an advanced mechanochemical device for sustainable synthetic processes using single-screw drill (SSD).59 Aldehyde (105) was treated with ketone (106) in the presence of 10 mol% KOH as a base to afford chalcone product (107) within 5 min reaction time, giving 71–99% yield, as shown in Scheme 27. The authors have reported 11 examples avoiding the column chromatographic purification technique. The aldehyde and ketone were treated with urea in the presence of NaOH solution using SSD device for 5 min to afford dihydropyrimidinones (108) (4 examples) in 47–80% yield. The aldehyde and methyl acetoacetate were reacted with urea in the presence of acid catalyst p-toluenesulfonic acid using an SSD for 20 min to afford dihydropyrimidinethiones (109) (4 examples) in 96–98% yield. In the reaction of 4-iodobenzaldehyde, acetophenone, phenylhydrazine, and solid powder of KOH were brought in contact using a drilling machine for 1 h to obtain product pyrazolines (110) in 42% yield without using solvent and avoiding column chromatography; the final product was isolated after recrystallization in ethanol.
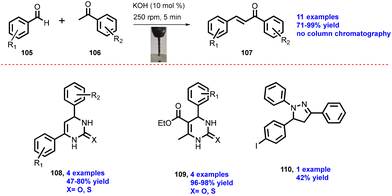 |
| Scheme 27 Synthesis of chalcone, dihydropyrimidinones, dihydropyrimidinethiones, and pyrazolines using SSD reactor. | |
4.1.2 Greener dye synthesis.
In 2020, James and co-workers described a solvent-free dye synthesis by TSE.60 3,4,9,10-Perylenetetracarboxylic dianhydride (PTCDA) (111) was treated with alkyl/benzyl substituted amine (112) by TSE at 55 rpm and 110 °C to obtain perylene diimides (PDIs) (113) in 8 min, giving 51–95% yield with broad substrate scope (12 examples) (Scheme 28). Similarly, the same protocol was employed for PTCDA (111) subjected to aniline-substituted amine (114) with potassium carbonate as a base by TSE at 15 rpm and 200 °C to obtain perylene diimides (PDIs) (115) in 8 min with moderate 23–73% yield (10 examples) (Scheme 28). The authors have given good applications of pigment black 31, pigment black 32, and pigment red 190. Joshi and co-workers have developed the synthesis of 3,4-dihydropyridin-2(1H)-ones/thiones by TSE under solvent- and catalyst-free conditions.61
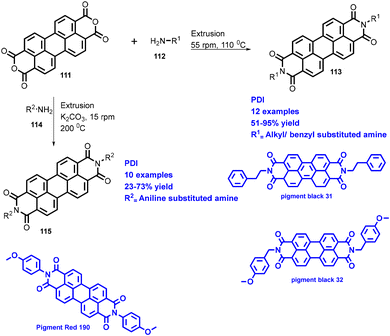 |
| Scheme 28 Alkyl/benzyl and aniline-substituted perylene diimides (PDIs) synthesis using TSE. | |
The Biginelli reaction was performed by reacting benzaldehyde (116) with ethyl acetoacetate (117) and urea/thiourea (118) to afford 3,4-dihydropyridin-2(1H)-ones/thiones (119) by TSE at 50 rpm and 120 °C with good yield (91%) within short reaction time (9–11 min) (Scheme 29). Dastager and co-workers have described a solid phase continuous fluorination reaction,62 where the heterogeneous NBu4(Bac-cell–OH)–F (120) complex reacted with halide compounds (121) in a flow reactor in-house built screw reactor to form a fluorinated compound (122) in 25 s and 70 °C in excellent yield (65–96%). Furthermore, the authors have reported wide substrate scope (12 examples, 122a–122I), as shown in Scheme 30.
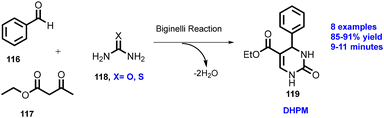 |
| Scheme 29 Solvent-free Biginelli reaction using TSE. | |
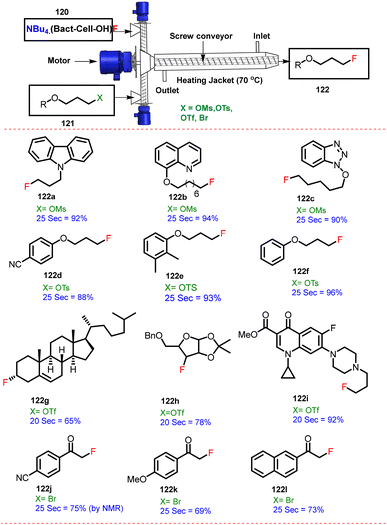 |
| Scheme 30 Continuous solvent-free fluorination reaction by screw reactor. | |
In 2018, Browne's group transformed an organic synthesis from mixture mill to continuous TSE.63 The authors have for the first time reported organic fluorination by TSE. The diketone (123) reacted with Selectfluor with sodium carbonate as a base by TSE at 280 rpm to achieve 83% of di-fluorinated (125) and 3% yield mono-fluorinated (124) product at 70 °C. The yield of mono-fluorinated product was found to increase when increasing the amount of acetonitrile up to 2 mL, leading to 100% conversion of the starting material. The yield of mono-fluorinated (126) and di-fluorinated (127) product was 81% and 14%, respectively, at 60 °C, as shown in Scheme 31.
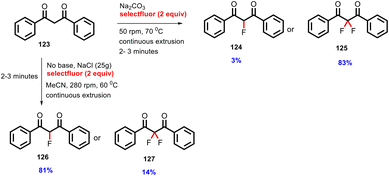 |
| Scheme 31 Selectivity of the mono and di-fluorination reaction by TSE. | |
4.2 Active pharmaceutical ingredients (APIs) by continuous mechanochemistry
Very recently, Patil et al. in 2021 have reported a multistep synthesis of miltefosine with continuous mechanochemistry64 within 300 s using screw reactor with 58% overall yield without difficult processing and purification. The first three steps were reported in plug flow reactor with solution-based chemistry, while the last step was reported using continuous mechanochemical approach at 90 °C with screw reactor (Scheme 32). The optimized reaction conditions of miltefosine using screw reactor is shown in Table 2. It noted that the overall reaction time (4 steps) was very short (34 min), shorter than that of the conventional batch protocol (∼15 h).
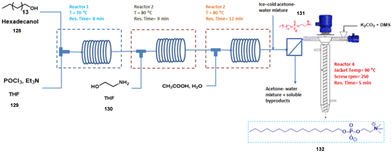 |
| Scheme 32 Telescopic continuous flow synthesis of miltefosine. | |
Table 2 Optimization conditions of miltefosine using screw reactor at different reactions times (t)
Sr. No. |
Solvent (mL) |
DMS : K2CO3 (eq.) |
Temp (°C) |
RPM |
t (min) |
Observation |
1 |
THF : H2O |
3 : 3 |
RT |
500 |
1 |
No product formation |
2 |
THF : H2O |
3 : 3 |
50 |
300 |
2 |
3 |
THF : H2O |
3 : 3 |
70 |
300 |
2 |
4 |
THF : H2O |
5 : 5 |
60 |
250 |
5 |
5 |
THF : H2O |
5 : 5 |
80 |
250 |
5 |
Some product formation |
6
|
THF : H2O |
5 : 5 |
90
|
250
|
5
|
Significant product formation
|
Peptide (aspartame) synthesis by extruder.
Recently, Yeboue et al. have described an aspartame synthesis via a solvent-free mechanochemical route.65 In this protocol, a mixture of Boc–Asp(OBn)–OSu (133) and HCl·NH–Phe–OMe (134) was treated with NaHCO3 and few drops of acetone were added in to the extruder at 40 °C, 150 rpm to form crude dipeptide Boc–Asp(Bn)–Phe–OMe (135) as a white paste within 1.5 min, as shown in Scheme 33. Then, dipeptide (135) was reduced using H2, Pd/C in methanol at room temperature for 1.5 h with 98% yield to form product (136), followed by the deprotection of the Boc group using gaseous HCl to form aspartame (137) in 90% yield and 81% overall yield over three steps (Scheme 33). In 2020, Crawford et al. reported a solvent-free synthesis of hydrazine-related bioactive compound using twin screw exstrusion,66 and post synthetic work up was not required. In this advanced strategy, cyclic amide (138) reacted with benzaldehyde (139) to afford imine product (140) by TSE at 55 rpm and 150 °C in 2 min to obtain the E-major isomer selectively (Scheme 34). The same protocol was employed for the synthesis of nitrofurantoin (141) and dantrolene (142) with good conversion and E-isomer was selectively obtained.
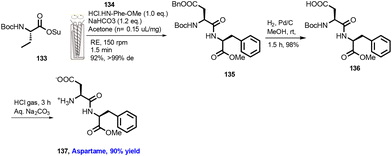 |
| Scheme 33 Aspartame synthesis using TSE. | |
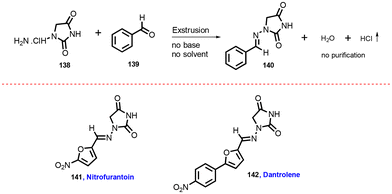 |
| Scheme 34 Solvent-free synthesis of nitrofurantoin and dantrolene by TSE. | |
5. Safety in mechanochemistry
We have highlighted safety issues in batch and continuous mechanochemistry for first time. The highlighting points are mentioned below.
i. Mechanochemical reactions should always be carried out inside fume hoods and appropriate quenching agents must be used to treat excess reagent or unreacted chemicals. Typical reactions involving only powders or pastes or slurries that exit the continuous screw reactors are in high concentration and can be reactive. Reactions involving chlorinating agents, nitric acid, etc., have significant hazards unless quenched immediately.
ii. Typically, the ball mills used for communition offer less than 25% energy efficiency, while an extruder (depending on the design) offers 45–75% efficiency. Similar numbers are valid even for mechanochemistry. Due to lower efficiency in ball mills, longer milling times or higher vibration frequencies are necessary to achieve the desired conversion while ensuring the impact temperatures under limit. Higher frequency as well as intensity can be detrimental, especially for the reactions where gases get generated or the impact temperature is higher than the boiling point of any of the substrates. In such cases, using a continuous mechanochemical approach is safer as well as better, provided the reaction times are not too long.
iii. In a solvent-free environment, highly exothermic reactions usually proceed through potentially dangerous intermediates such as reactive intermediates that can undergo self-accelerating thermal decomposition (viz., diazotization, aromatic nitration, n-BuLi, Grignard reaction, sensitive metal catalyzed reactions, etc.). Considering the eventual scalability of the approach, heat duty must be estimated for such cases and appropriate temperature controls must be in place along with lower shear rates (i.e., lower rotation speeds) and appropriate shields. Using a pressure transmitter to monitor pressure build-up and a few temperature transmitters connected to an alarm will help avoid run-away conditions. Our experience of designing large scale solvent-free industry scale mechanochemical systems (>5000 kg per day) clearly suggest that appropriate measures must be evolved right from the laboratory scale.
iv. In the absence of solvents, the reaction rates get accelerated due to higher concentrations. In conventional synthesis, using excess reagents is a usual protocol to reduce the order of the reaction. In mechanochemical synthesis, such an option actually defies the purpose; hence, it is always useful to have stoichiometric quantifies of the reagents. Also, since the kinetics for solid–solid reactions may not follow the similar rate laws, more studies on the role of excess substrates for such cases would help to get a better understanding of the kinetics.
v. A few notable examples can be seen in the literature where ball milling is used for thermally unstable azide synthesis.73 However, for reagents/substrates/products that are shock-sensitive or shear-sensitive (viz. diazonium salts, nitro-aromatics, viz., alkyl nitrates such as nitrocellulose and nitroglycerine, acetylenic compounds, heavy metal salts of acetylenes, acyl nitrates, etc.) it is not recommended to use a ball mill or even a screw system. Since the concentrations are always high, translation of reaction waves into the media is not as easy as for homogeneous liquid, liquid–liquid, or gas–liquid reactions. The possibility of absorption or dissipation of a shock wave are very less. Hence, new designs that allow vents along the screw length will help.
vi. Dust release during the operation becomes important if the ball mills of a screw are not rightly concealed. Prevention of dust release after using a ball mill is necessary to avoid respiratory issues. It is necessary to allow a sufficiently long settling time for the particles before opening a ball mill. Similarly, for a screw system, the outlet should be enclosed and be connected to a collector. Typically, as the scale of operation increases, the severity of dusting becomes a reality and needs special equipment, viz., vacuum filtration systems or special dust masks. For a continuous single or twin screw-based systems where dry powder is an outcome from a truly solid–solid reaction, it is necessary to have proper ducting in the vicinity of the set-up to avoid the spreading of toxic dust.
vii. Pressure generation due to evaporation of low boiling byproducts or even a solvent is a serious issue and one must use mechanical seals on the system to avoid backpressure leading to leakage into the motor shafts. Such situations can be unsafe if the phase getting released from the system can be hot and under pressure. Details can be found in the literature on the design of twin screw systems.74
viii. Usually, in twin screw systems, metallic heating elements are placed along the screw length and they work in ON/OFF mode. For exothermic solvent-free reactions in screw systems, it is necessary to maintain a specific temperature profile along the screw length. ON/OFF mode systems are not suitable in such cases and local cooling systems are a must. This is typically true for fusion kind of reactions or even for aromatic nitration of solid substrates and hence must be done with enough care. Reactions such as chlorination using thionyl chloride or sulfuryl chloride tend to generate solid products and gaseous HCl. Handling three phases in reactive extrusion screw systems with necessary temperature controls is essential to avoid unsafe situations.
ix. Variation in the flow regimes inside the screw system due to change in the physiochemical properties is typically not accounted while using such systems. As a result, the voidage created due to the generation of vapors of gases remains stagnant and needs to be taken seriously as it can affect the selectivity significantly.
x. It is always necessary to monitor the power drawn by the mechanochemical system. If the power drawn continues to increase, it indicates excessive friction of the screw with the barrel, while continuous lowering power drawn indicates no work being done due to erosion of the screw. The latter can result in the erosion of the screw and can damage the barrel internally, which can compromise on the safe operation of the screw reactor. While this aspect seems trivial and more of a mechanical alignment of the screw, the orientation of the screw and uniformity in the thread depth cannot be ignored.
6. Conclusions
Batch and continuous mechanochemistry has the potential to change the way organic synthesis is done over the last many decades. Using large quantities of solvents has been a practice and the approach has continued even for commercial scale manufacturing. This review gives an update on the status of the subject, different synthesis approaches, and safety concerns in continuous mechanochemistry.
Solvents are neither products not reactants, they are merely reagents that facilitate dissolution to get homogeneous reaction mass or help keep reactants in contact through suspension or by helping to create a dispersion. Depending on the sensible heat as well as latent heat needed for the recovery of solvents through distillation and condensation, a very large amount of energy is spent in commercial plants. Irrespective of the efficiency of the recovery systems, eventually, it all gets into the atmosphere as volatile organic compounds or in the effluent or solid matrix later used for landfill, all of which contaminate the environment. Mechanochemistry or reactive extrusion helps minimize or eliminate solvents almost completely from a synthesis, thereby making a process compact, green, and efficient.
To date, a vast class of reactions have been reported successfully through mechanochemistry (viz., fluorination using various fluorinating agents, synthesis of heterocyclic compounds, Biginelli reaction, Sonogashira coupling, diazotization, alcohol oxidation, direct amidation, peptide synthesis, acylation, nucleophilic substitution, TBS protection, oxidative dimerization, chalcone synthesis, imine formation, Knoevenagel condensation, aldol condensation, Michael addition, hydantoin hydrazone, perylene diimides synthesis, etc.). The real impact of mechanochemistry can be realized only when it is taken from the pilot scale to commercial scale manufacturing. This also requires chemists and chemical engineers to work together right from the beginning. Just showcasing every chemistry in mechanochemical way is not sufficient; establishing a detailed mass and energy balance are a must, and such details are not seen in the recent literature.
A properly optimized process in a reactive extrusion can be controlled very well through the control of temperature and mixing along the length of screw. Particle sizes, their stiffness, crystalline or amorphous nature, melting point, and ability of the device to remove or scrap the reacted surface to facilitate new surface undergo reaction govern the reaction rates, thus making it an interesting alternative to conventional synthesis protocols.
The scale-up of such systems for reaction needs further exploration. The guidelines for extruder scale-up need to be used with additional information from the heat of reaction to design such systems for specific set of reactants.
7. Future directions/challenges
Solid–solid reactions have been studied in depth almost 5 decades ago. With the available tools and techniques handy at that time, different reaction mechanisms were proposed and used to justify the design approach. However, the field did not progress to a great extent until recently, when the sustainability aspect has forced us to avoid solvents and conduct neat reactions. Here, we highlight a few challenges that will decide the direction of progress of this field.
a) Mechanisms: understanding the exact reaction mechanism (molecular scale) as well as phenomenological mechanism (bulk scale) would be a challenge in mechanochemistry. The known literature gives conventional framework from classical solvation dynamics-based molecular interactions while the bulk scale reaction engineering67 approaches use concepts similar to two film theory for diffusion, surface renewal, spot contact mechanism, or shrinking core models, etc. However, finding the correct reaction mechanism and subsequently the reaction kinetics will be key for the design of mechanochemical reactors as the Arrhenius approach of defining the rate constant may or may not hold.
b) Uncharted reactions: a recent wok by Patel et al.75 reports the use of CaF as an excellent fluorinating agent when used in solvent-free environments at much milder conditions and over a wide range of substrates. Such efforts will help new reactions to be explored in a rather more selective manner.
c) In situ analysis: inline analysis of the solid phase for monitoring the reaction progress is an important challenge as the segregation of particles in the matrix, formation of sticky agglomerate, or inefficient removal of the product from the surface of reactants and particle size variation during reaction need more quantification depending on individual chemistries. Tools such as in-line IR and spatiotemporal characterization can be helpful.
d) Optimization: multistep mechanochemical synthesis of organic compounds that need very long reaction time and continuous isolation and separation of products is a complex task. Optimization of the reaction to complete conversion with almost atom-economic selectivity will be the key drivers for such systems.
e) Widespread adaption in R&D labs: the development and spread of mechanochemistry as a synthesis tool in the last 5 years has happened at a much higher rate than what it was for flow chemistry. Simple tools such as mortar–pestle helped the quick screening of various reactions, followed by the use of laboratory scale ball mills and now continuous reactive extrusion. The first and the second tools have certain limitations in terms of the type of reactions that can be performed (viz., high pressure, integration with photo, electro, and microwave energy), while continuous mechanochemistry will overcome most of these limitations including material of wetting phase or even the handling of gases or vapors. It will be better if the synthesis community moves away from ball milling and adapts the latter approach, which will bring a significant and sustainable technology disruption.
f) Screw design: developing innovative screw designs with elements for mixing, heat transfer, reaction, handling of phase change, etc., are known to some extent. Such designs for single screw, twin screw, and even for a triple screw system need to be studied extensively to find their suitability for carrying out reactions.
g) Reliable design guidelines settings: quantitative estimation of the design parameters, viz., mixing efficiency, heat and mass transfer characteristics, and reaction time distribution, is very important as it will help quickly select the desired screw for a given reaction.68 At this moment, such design principles are known only for extrusion purpose without any intrinsic heat duty or need for efficient radial mixing. If continuous mechano-chemistry is to be deployed for pilot or commercial scales, then developing design guidelines is a must.
h) Scalability (and the main limitations): the scalability of a single screw or a twin screw system is known for extrusion purpose. While those rules and guidelines can be applicable for reactive extrusion, the main difference is in terms of the spatial temperature variation and more importantly, spatial variation in the physical properties, mainly the thermal conductivity. It is essential to know the reaction kinetics even for such reaction systems to get to know a possible rate curve along the screw length, provided the design offers excellent ‘mixing within the cavity of threads’ and ‘no adhesion to wall’. There can be rare cases of the second kind, but a twin screw or a tri-screw system can remove such deposition. Thus, it is very much possible to apply the classical approach for the design of continuous solvent-free synthesis in a screw reactor, provided the assumptions, viz., product formed on the solid surface gets removed due to shear and exposes the new surface for further reaction, hold true. The field is evolving and in the near future, detailed design procedures will definitely appear in the literature.
In the recent literature, a few thoughts on future directions and challenges72 on batch and continuous mechanochemistry have been reported. Some of these include mechanochemistry for carrying out the impossible reactions,69 green metrics in mechanochemistry,70 and mechanochemical rearrangement for organic synthesis with eco-friendly approach, especially for active pharmaceutical and complex natural products.71 Recent reports on direct continuous mechanochemical solvent-free amidation76,77 cover an important step toward solvent-free manufacturing.
Thus, while continuous mechanochemistry has a huge potential to address many of the principles of green chemistry and green engineering, ample works needs to be done to make it a reality. Authors have set-up large pilot scale (∼50 kg per day) and commercial scale (100 kg per day to 5000 kg per day) solvent-free continuous manufacturing plants for a few important specialty chemicals and intermediates. Many of the points mentioned in Section 6 and 7 are based on those experiences. Looking at the large number of literature reports and industry's interest in exploring as well as adapting continuous mechanochemistry in recent times, it is quite certain that this approach will have a noticeable impact on sustainable chemical manufacturing. The “rush-to-publish-first” to report a new transformation in batch as well as continuous mechanochemistry is analogous to what was seen in the first decade of this century for “Flow Chemistry” or in the decade before that in “microwave chemistry” and is quite expected. The real impact will be only through large scale manufacturing. A combination of continuous flow synthesis and mechanochemistry offers an excellent opportunity to chemists and chemical engineers to change the landscape of chemical manufacturing in a true sense, before large scale biomanufacturing or large-scale organic transformations using mechanochemistry becomes a reality in a couple of decades.
Author contributions
Ranjit Atapalkar: investigation, methodology, writing – original draft, writing – review & editing. Amol Kulkarni: conceptualization, supervision, review & correcting, funding acquisition.
Conflicts of interest
There are no conflicts of interest to declare.
Acknowledgements
Ranjit S. Atapalkar acknowledges the PhD fellowship funding from the CSIR (31/011(1005)/2017-EMR-I). Authors acknowledge the funding from CSIR NCP project (MLP100926).
Notes and references
- P. Ying, J. B. Yu and W. K. Su, Adv. Synth. Catal., 2021, 363, 1246–1271 CrossRef CAS.
- W. Jones and M. D. Eddleston, Faraday Discuss., 2014, 170, 9–34 RSC.
- J. L. Do and T. Friscic, ACS Cent. Sci., 2017, 3, 13–19 CrossRef CAS.
- L. Takacs, Chem. Soc. Rev., 2013, 42, 7649–7659 RSC.
- S. L. James, C. J. Adams, C. Bolm, D. Braga, P. Collier, T. Friscic, F. Grepioni, K. D. M. Harris, G. Hyett, W. Jones, A. Krebs, J. Mack, L. Maini, A. G. Orpen, I. P. Parkin, W. C. Shearouse, J. W. Steed and D. C. Waddell, Chem. Soc. Rev., 2012, 41, 413–447 RSC.
- R. B. N. Baig and R. S. Varma, Chem. Soc. Rev., 2012, 41, 1559–1584 RSC.
- C. Jimenez-Gonzalez, D. J. C. Constable and C. S. Ponder, Chem. Soc. Rev., 2012, 41, 1485–1498 RSC.
- S. Mateti, M. Mathesh, Z. Liu, T. Tao, T. Ramireddy, A. M. Glushenkov, W. R. Yang and Y. I. Chen, Chem. Commun., 2021, 57, 1080–1092 RSC.
- C. Hardacre, H. F. Huang, S. L. James, M. E. Migaud, S. E. Norman and W. R. Pitner, Chem. Commun., 2011, 47, 5846–5848 RSC.
- V. Strukil, B. Bartolec, T. Portada, I. Dilovic, I. Halasz and D. Margetic, Chem. Commun., 2012, 48, 12100–12102 RSC.
- J. L. Howard, Q. Cao and D. L. Browne, Chem. Sci., 2018, 9, 3080–3094 RSC.
- A. Stolle, T. Szuppa, S. E. S. Leonhardt and B. Ondruschka, Chem. Soc. Rev., 2011, 40, 2317–2329 RSC.
- E. C. Gaudino, G. Cravotto, M. Manzoli and S. Tabasso, Chem. Soc. Rev., 2021, 50, 1785–1812 RSC.
- X. Ma, G. K. Lim, K. D. M. Harris, D. C. Apperley, P. N. Horton, M. B. Hursthouse and S. L. James, Cryst. Growth Des., 2012, 12, 5869–5872 CrossRef CAS.
- J. G. Hernandez and E. Juaristi, Chem. Commun., 2012, 48, 5396–5409 RSC.
- T. Dalidovich, K. A. Mishra, T. Shalima, M. Kudrjašova, D. G. Kananovich and R. Aav, ACS Sustainable Chem. Eng., 2020, 8, 15703–15715 CrossRef CAS.
- T. X. Metro, J. Bonnamour, T. Reidon, A. Duprez, J. Sarpoulet, J. Martinez and F. Lamaty, Chem. – Eur. J., 2015, 21, 12787–12796 CrossRef CAS.
- G. F. Han, F. Li, Z. W. Chen, C. Coppex, S. J. Kim, H. J. Noh, Z. Fu, Y. Lu, C. V. Singh, S. Siahrostami, Q. Jiang and J. B. Baek, Nat. Nanotechnol., 2021, 16, 325–330 CrossRef CAS.
- C. L. He, Q. D. Li, X. Y. Zhang, Y. X. Lu, D. Qiu, Y. Chen and X. L. Cui, ACS Sustainable Chem. Eng., 2022, 10, 746–755 CrossRef CAS.
- M. Felderhoff, Joule, 2021, 5, 297–299 CrossRef CAS.
- Y. J. Li, S. P. Li, X. Y. Xu, J. Gu, X. J. He, H. Meng, Y. Z. Lu and C. X. Li, ACS Sustainable Chem. Eng., 2021, 9, 9221–9229 CrossRef CAS.
-
P. T. Anastas and T. C. Williamson, Green Chemistry: frontiers in benign chemical syntheses and processes, Oxford University Press, New York, NY, 1999, vol. 360 Search PubMed.
- W. I. Nicholson, F. Barreteau, J. A. Leitch, R. Payne, I. Priestley, E. Godineau, C. Battilocchio and D. L. Browne, Angew. Chem., 2021, 60, 21868–21874 CrossRef CAS PubMed.
- R. R. A. Bolt, J. A. Leitch, A. C. Jones, W. I. Nicholson and D. L. Browne, Chem. Soc. Rev., 2022, 51, 4243–4260 RSC.
- K. S. Suslick, Faraday Discuss., 2014, 170, 411–422 RSC.
- L. Takacs, J. Therm. Anal. Calorim., 2007, 90, 81–84 CrossRef CAS.
-
W. Ostwald, Die chemische Literatur und die Organisation der Wissenschaft, Akad. Verlag. Gesel., 1919, vol. 1 Search PubMed.
- L. Takacs, Bull. Hist. Chem., 2003, 28(1), 26–34 CAS.
- L. Takacs, J. Mater. Sci., 2004, 39, 4987–4993 CrossRef CAS.
-
(a) W. Spring, Bull. Acad. R. Med. Belg., 1880, 49(2), 323 Search PubMed;
(b) W. Spring, Bull. Soc. Chim. Fr., 1883, 39, 641 Search PubMed;
(c) M. C. Lea, Br. J. Photogr., 1866, 13, 84 Search PubMed;
(d) M. C. Lea, Am. J. Sci., 1892, 43(3), 527 CrossRef;
(e) M. C. Lea, Am. J. Sci., 1893, 46(3), 413 CrossRef.
- V. Strukil, M. D. Igrc, M. Eckert-Maksic and T. Friscic, Chem. – Eur. J., 2012, 18, 8464–8473 CrossRef CAS PubMed.
- T. Friscic, C. Mottillo and H. M. Titi, Angew. Chem., Int. Ed., 2020, 59, 1018–1029 CrossRef CAS PubMed.
- M. Perez-Venegas and E. Juaristi, ACS Sustainable Chem. Eng., 2020, 8, 8881–8893 CrossRef CAS.
- A. Delori, T. Friscic and W. Jones, CrystEngComm, 2012, 14, 2350–2362 RSC.
- T. Friscic, S. L. Childs, S. A. A. Rizvi and W. Jones, CrystEngComm, 2009, 11, 418–426 RSC.
- G. Kaupp, CrystEngComm, 2009, 11, 388–403 RSC.
- D. Tan and F. Garcia, Chem. Soc. Rev., 2019, 48, 2274–2292 RSC.
- V. Declerck, P. Nun, J. Martinez and F. Lamaty, Angew. Chem., Int. Ed., 2009, 48, 9318–9321 CrossRef CAS PubMed.
- T. Dalidovich, K. A. Mishra, T. Shalima, M. Kudrjasova, D. G. Kananovich and R. Aav, ACS Sustainable Chem. Eng., 2020, 8, 15703–15715 CrossRef CAS.
- J. L. Howard, Y. Sagatov, L. Repusseau, C. Schotten and D. L. Browne, Green Chem., 2017, 19, 2798–2802 RSC.
- M. Lanzillotto, L. Konnert, F. Lamaty, J. Martinez and E. Colacino, ACS Sustainable Chem. Eng., 2015, 3, 2882–2889 CrossRef CAS.
- A. D. Sharapov, R. F. Fatykhov, I. A. Khalymbadzha, V. V. Sharutin, S. Santra, G. V. Zyryanov, O. N. Chupakhin and B. C. Ranu, Green Chem., 2022, 24, 2429–2437 RSC.
- L. Konnert, A. Gauliard, F. Lamaty, J. Martinez and E. Colacino, ACS Sustainable Chem. Eng., 2013, 1, 1186–1191 CrossRef CAS.
- S. Kuntikana, C. Bhat, M. Kongot, S. I. Bhat and A. Kumar, ChemistrySelect, 2016, 1, 1723–1728 CrossRef CAS.
- Z. T. Bhutia, G. Prasannakumar, A. Das, M. Biswas, A. Chatterjee and M. Banerjee, ChemistrySelect, 2017, 2, 1183–1187 CrossRef CAS.
- S. Bagchi, A. Monga, S. Kumar, Deeksha and A. Sharma, ChemistrySelect, 2018, 3, 12830–12835 CrossRef CAS.
- T. X. Metro, J. Bonnamour, T. Reidon, J. Sarpoulet, J. Martinez and F. Lamaty, Chem. Commun., 2012, 48, 11781–11783 RSC.
- L. Konnert, B. Reneaud, R. M. de Figueiredo, J. M. Campagne, F. Lamaty, J. Martinez and E. Colacino, J. Org. Chem., 2014, 79, 10132–10142 CrossRef CAS PubMed.
- L. Konnert, M. Dimassi, L. Gonnet, F. Lamaty, J. Martinez and E. Colacino, RSC Adv., 2016, 6, 36978–36986 RSC.
- E. Colacino, A. Porcheddu, I. Halasz, C. Charnay, F. Delogu, R. Guerra and J. Fullenwarth, Green Chem., 2018, 20, 2973–2977 RSC.
- D. Tan, V. Strukil, C. Mottillo and T. Friscic, Chem. Commun., 2014, 50, 5248–5250 RSC.
- T. Portada, D. Margetic and V. Strukil, Molecules, 2018, 23(12), 3163 CrossRef PubMed.
- Z. J. Jiang, Z. H. Li, J. B. Yu and W. K. Su, J. Org. Chem., 2016, 81, 10049–10055 CrossRef CAS PubMed.
- L. Jiang, L. D. Ye, J. L. Gu, W. K. Su and W. T. Ye, J. Chem. Technol. Biotechnol., 2019, 94, 2555–2560 CrossRef CAS.
- P. Zhang, C. Q. Liu, L. Yu, H. Q. Hou, W. M. Sun and F. Ke, Green Chem. Lett. Rev., 2021, 14, 610–617 CAS.
- F. J. Wang, H. Xu, M. Xin and Z. Zhang, Mol. Diversity, 2016, 20, 659–666 CrossRef CAS PubMed.
- B. M. Sharma, R. S. Atapalkar and A. A. Kulkarni, Green Chem., 2019, 21, 5639–5646 RSC.
- D. E. Crawford, C. K. G. Miskimmin, A. B. Albadarin, G. Walker and S. L. James, Green Chem., 2017, 19, 1507–1518 RSC.
- C. Gomes, C. S. Vinagreiro, L. Damas, G. Aquino, J. Quaresma, C. Chaves, J. Pimenta, J. Campos, M. Pereira and M. Pineiro, ACS Omega, 2020, 5, 10868–10877 CrossRef CAS PubMed.
- Q. Cao, D. E. Crawford, C. C. Shi and S. L. James, Angew. Chem., Int. Ed., 2020, 59, 4478–4483 CrossRef CAS PubMed.
- R. B. Carvalho and S. V. Joshi, Green Chem., 2019, 21, 1921–1924 RSC.
- M. S. Said, N. S. Khonde, M. N. Thorat, R. S. Atapalkar, A. A. Kulkarni, J. Gajbhiye and S. G. Dastager, Asian
J. Org. Chem., 2020, 9, 1022–1026 CrossRef CAS.
- Q. Cao, J. L. Howard, D. E. Crawford, S. L. James and D. L. Browne, Green Chem., 2018, 20, 4443–4447 RSC.
- N. B. Patil, R. S. Atapalkar, S. P. Chavan and A. A. Kulkarni, Chem. – Eur. J., 2021, 27, 17695–17699 CrossRef CAS PubMed.
- Y. Yeboue, B. Gallard, N. Le Moigne, M. Jean, F. Lamaty, J. Martinez and T. X. Metro, ACS Sustainable Chem. Eng., 2018, 6, 16001–16004 CrossRef CAS.
- D. E. Crawford, A. Porcheddu, A. S. McCalmont, F. Delogu, S. L. James and E. Colacino, ACS Sustainable Chem. Eng., 2020, 8, 12230–12238 CrossRef CAS.
- S. S. Tamhankar and L. K. Doraiswamy, AIChE J., 1979, 25(4), 561–582 CrossRef CAS.
- T. Friscic, S. L. James, E. V. Boldyreva, C. Bolm, W. Jones, J. Mack, J. W. Steed and K. S. Suslick, Chem. Commun., 2015, 51, 6248–6256 RSC.
- F. Cuccu, L. De Luca, F. Delogu, E. Colacino, N. Solin, R. Mocci and A. Porcheddu, ChemSusChem, 2022, 15, 362 CrossRef PubMed.
-
N. Fantozzi, J. Volle, A. Porcheddu, D. Virieux, F. Garcia and E. Colacino, ChemRxiv, 2022, preprint, p. 370, DOI:10.26434/chemrxiv-2022-b370p.
- D. Virieux, F. Delogu, A. Porcheddu, F. García and E. Colacino, J. Org. Chem., 2021, 86(20), 13885–13894 CrossRef CAS PubMed.
- E. Colacino, V. Isoni, D. Crawford and F. García, Trends Chem., 2021, 2, 8 Search PubMed.
- K. Yoo, E. Hong, T. Huynh, B. Kim and J. Kim, ACS Sustainable Chem. Eng., 2021, 9(26), 8679–8685 CrossRef CAS.
- C. Martin, AAPS PharmSciTech, 2016, 17, 3–19 CrossRef PubMed.
- C. Patel, E. André-Joyaux, J. A. Leitch, X. M. de Irujo-Labalde, F. Ibba, J. Struijs, M. A. Ellwanger, R. Paton, D. L. Browne, G. Pupo, S. Aldridge, M. A. Hayward and V. Gouverneur, Science, 2023, 381, 302–306 CrossRef CAS PubMed.
- R. Atapalkar and A. A. Kulkarni, Chem. Commun., 2023, 59, 9231 RSC.
- M. Lavayssiere and F. Lamaty, Chem. Commun., 2023, 59, 3439–3442 RSC.
|
This journal is © The Royal Society of Chemistry 2024 |