DOI:
10.1039/D4MA00775A
(Review Article)
Mater. Adv., 2024,
5, 8404-8418
Advancements in MXenes and mechanochemistry: exploring new horizons and future applications
Received
1st August 2024
, Accepted 14th September 2024
First published on 16th September 2024
Abstract
MXenes, a class of two-dimensional (2D) materials derived from transition metal carbides, nitrides, and carbonitrides, have garnered significant attention due to their unique properties and potential applications in various fields, including energy storage, catalysis, and electronics. Mechanochemistry, the study of chemical reactions driven by mechanical forces, offers a novel approach to synthesize and manipulate MXenes, enhancing their properties and expanding their functional applications. This review explores the intersection of MXenes and mechanochemistry, highlighting recent advancements in the mechanochemical synthesis of MXenes and their derivatives. We discuss the mechanisms underlying the mechanochemical processes, including the role of shear forces, ball milling, and other mechanical techniques in facilitating the exfoliation and functionalization of MXenes. Furthermore, we examine the impact of mechanochemical methods on the structural integrity, surface chemistry, and electronic properties of MXenes, which are crucial for their performance in applications such as supercapacitors, batteries, and sensors. This review also addresses the challenges and limitations associated with mechanochemical approaches, including scalability and reproducibility, while proposing future directions for research in this promising field. By integrating mechanochemistry with MXene research, we aim to provide insights into innovative strategies for the development of advanced materials that can meet the demands of next-generation technologies. This synthesis of knowledge not only underscores the versatility of MXenes but also emphasizes the transformative potential of mechanochemistry in materials science.
1. Introduction
MXenes are a family of two-dimensional (2D) transition metal carbides, nitrides, or carbonitrides that are endowed with excellent electrical conductivity, high mechanical strength, hydrophilicity, large specific surface area, and intriguing surface chemistry.1,2 MXenes have a general formula of Mn+1XnTx (n = 1–4), where M represents transition metals, X is carbon and/or nitrogen, and T stands for surface termination groups (–OH, –F, –O or −Cl). They are synthesized by selectively etching the A element from the MAX (M = metal, A = A-group element, X = C and/or N) phases, which are layered ternary compounds (Fig. 1).3,4 The ensuing MXene structure features a layered morphology with abundant surface functional groups, making it highly attractive for a wide variety of applications, including energy conversion/storage,1,5–7 catalysis,8–11 imaging/sensing,12–14 electromagnetic shielding,15,16 optoelectronics,17 wireless communication,18 triboelectrics,19 and water treatment/purification.20 MXenes have been incorporated into various composite forms by combining them with polymers, metals, ceramics, or other nanomaterials to enhance their properties and expand their potential applications in view of their unique attributes like high electrical conductivity and large surface area.13,21,22 In energy storage, MXenes have shown promise as electrode materials in supercapacitors and batteries due to their high electrical conductivity and large surface area, enabling efficient charge storage.23–26 MXene composites have also been explored for their use in electromagnetic shielding, as their conductivity can effectively dissipate electromagnetic radiation.15,16,27,28 Moreover, MXenes have shown potential as electro- and photocatalysts for various chemical reactions due to their unique surface chemistry and abundant active sites. They have demonstrated effectiveness as photocatalysts and co-catalysts in processes such as water splitting, reduction of CO2, and the elimination of organic pollutants from wastewater.29,30 MXene and semiconductor hybrids have been demonstrated to be powerful photocatalysts due to the distinctive characteristics of their interface, which enables rapid charge separation and reduces the Schottky barrier.9,31 Notably, MXenes possess remarkable electronic, mechanical, chemical, and physical properties, along with controllable surface groups and a large surface area, making them highly promising catalysts for energy conversion reactions. Moreover, the exceptional electrical conductivity and surface properties of MXenes render them suitable for the photocatalytic removal of organic contaminants from wastewater. These outstanding attributes contribute to their potential in diverse catalytic applications.32–34
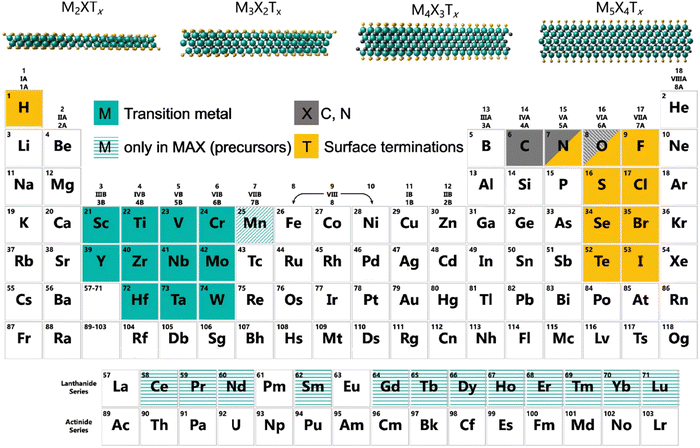 |
| Fig. 1 The elements utilized in the synthesis of MXenes are illustrated in the periodic table. Metals (M elements) are denoted in green, nonmetals (X elements) in gray, and terminating elements (T) in dark yellow. Rare-earth elements, indicated by striped backgrounds, could be part of in-plane ordered MAX structures. Elements with diagonal stripes are exclusively found in MXene precursors (MAX phases). Oxygen is distinctly identified by gray diagonal stripes owing to its role in the newly discovered oxycarbide MXene. Reproduced with permission from ref. 4. Copyright 2022 Springer Nature. | |
MXenes and their composites offer several advantages that make them attractive for various applications because of high electrical conductivity, combined with a large surface area, which enables efficient charge storage and transport. Surface functional groups offer customization possibilities for adjusting their interactions with other materials, improving compatibility and performance in composite integration.35,36 Moreover, MXenes are relatively lightweight and mechanically robust, making them suitable for applications such as electromagnetic interference shielding, where weight and strength are critical factors.37,38 While MXenes and their composites boast numerous advantageous features, addressing several challenges is crucial for their widespread adoption. Further research is imperative to optimize the dispersion of MXenes in composite materials to fully leverage their reinforcing capabilities. Another significant challenge is the scalability of MXene synthesis, as the current methods often involve harsh etching conditions and low yields.39 Additionally, the stability of MXenes in various environments, such as aqueous solutions or at elevated temperatures, needs to be improved to ensure their long-term performance.40 For instance, the environmental instability of an MXene (Ti3C2Tx) was addressed,41 wherein even at low concentrations, the dark green aqueous dispersion of the monolayer optimized MXene demonstrated no signs of oxidation or degradation after being aged under ambient conditions for two months. In contrast, conventional Ti3C2Tx experienced complete oxidative degradation within ∼10 days under the same conditions. Importantly, optimized Ti3C2Tx exhibited enhanced conductivity, improved nonlinear optical properties, excellent thermal stability, and comparable biocompatibility relative to its conventional counterpart.41
Mechanochemistry, the study of chemical reactions driven by mechanical forces, has emerged as a powerful tool in materials science,42–44 especially for the synthesis and processing of MXenes with exceptional properties and applications.45 Mechanochemical methods have proven to be highly effective for the synthesis and exfoliation of MXenes, as exemplified in the case of Pt/MXene (Nb2CTx) composites with improved electrocatalytic hydrogen evolution performance.46 MXenes are commonly synthesized from MAX phases, resulting in layered structures with plentiful surface functional groups, rendering them highly appealing for diverse applications.47–50 Mechanochemistry involves the use of mechanical forces, such as ball milling or grinding, to initiate chemical reactions or induce structural transformations in solid-state materials. Importantly, it eliminates the need for many solvents, offering the possibility that mechanochemistry could help make many industries more environmentally friendly. Mechanochemical synthesis can enhance reactivity, produce metastable compounds, and allow precise control over composition and structure. This is a highly efficient strategy requiring less energy and time and rendering the overall operation a sustainable and environmentally friendly process.51,52 This article highlights the significant advancements in MXenes and mechanochemistry, emphasizing the potential of mechanochemical techniques to tailor the properties of MXenes. By shedding light on the underlying mechanisms and recent research progress, this article opens the door for further exploration and optimization of mechanochemical synthesis strategies, propelling MXene research to new heights of innovation and applications.
2. MXenes and mechanochemistry
2.1. Mechanochemical synthesis
Mechanochemical synthesis has garnered significant attention in recent years due to its ability to produce a wide range of functional materials with unique properties under sustainable conditions. Its simplicity, versatility, enhanced reactivity, and eco-friendly nature make it an attractive option for diverse applications, leading to fewer purification steps and ultimately reducing the overall environmental impact of chemical production.42,52–54 Typically, the protocol relies on the mechanical forces generated by ball milling or other forms of mechanical energy input to initiate chemical reactions in solid-state materials. The process involves the milling of reactant materials together, leading to mechanical deformation, fracturing, and localized heating. These mechanical actions enable the breaking of chemical bonds, diffusion of reactants, and subsequent formation of new compounds. The high-energy collisions between the milling balls and the reactant particles provide the necessary activation energy for chemical reactions to occur.55,56 Despite persistent challenges, such as contamination and scalability, ongoing research and technological advancements aim to overcome these obstacles, further expanding the scope and impact of mechanochemical synthesis in the materials science domain.42,46 Mechanochemical fabrication offers several unique advantages, including its simplicity of operation and ease of scale-up and the ability to create a uniform reaction environment that results in nanoparticles with consistent size and shape. Mechanochemical processing of bulk solids has become a highly efficient and reactive technique for synthesizing and transforming different (nano)materials.52 Unlike traditional approaches, mechanochemical synthesis relies on simple techniques such as milling in comminution devices. However, recent advancements have demonstrated mechanochemical reactivity combined with other energy sources commonly deployed in solution-based chemistry.52 These include controlled temperature milling, light irradiation, sound agitation, and electrical impulses in innovative experimental setups. These novel approaches have enabled reactions that were previously unattainable through conventional mechanochemical processing. The techniques of thermo-, sono-, electro-, and photo-mechanochemical syntheses represent significant progress in modern mechanochemistry, opening up newer possibilities for solid-state reactivity, which have been comprehensively reviewed by Martinez et al.52
Mechanochemical processing is a promising sustainable methodology for producing metal-oxide nanoparticles with minimal environmental impact57 as it curbs the use of organic solvents. This not only reduces the environmental footprint but also offers the potential for more precise control over particle-size distribution. In contrast, conventional liquid-phase synthesis techniques typically involve the use of organic solvents, while vapour-phase synthesis requires organic precursors. Furthermore, water-based liquid-phase synthesis, despite its high reaction rate, often presents challenges in achieving precise control of particle-size distribution without the aid of surfactants.58 Besides, it is important to note that high-energy ball milling can result in significant energy consumption. To accurately assess the environmental advantages of various bottom-up approaches for obtaining metal oxide nanoparticles, a thorough life-cycle evaluation ought to be performed. Some studies have shown that the mechano-synthesis of organometallic species exhibits a low E-factor and low-process mass intensity when compared to conventional solution techniques57,59 while offering a distinct advantage by minimizing agglomeration.57 This is possible due to the presence of a solid by-product matrix that serves as a physical barrier between particles during the particle-growth phase, which is not the case for liquid-phase or vapour-phase fabrication methods often resulting in particle agglomeration. While liquid-phase synthesis methods can employ surfactants or micelles to deter agglomeration, this approach limits the options for surface treatment of nanoparticles during the application stage. Moreover, in the absence of a solid barrier, liquid-phase or vapour-phase synthesis requires highly dilute conditions to avoid particle agglomeration, leading to reduced product yield.57 However, there are some important disadvantages/limitations while deploying mechanochemical over other techniques. The end products from mechanochemical operations can be susceptible to contamination from the by-product phase, milling balls, or milling containers.57 Furthermore, its usage can sometimes lead to lower crystallinity of the final product owing to the presence of residual defects and amorphization caused by the mechanical energy input. Although annealing subsequent to milling operation can help improve the crystallinity of nanomaterials generated by mechanochemical approaches, there is a risk of particle agglomeration or the migration of impurities from the by-product phase into the nanomaterials during the heating process.57
Mechanochemical synthesis of MXenes typically involves the use of high-energy ball milling or other mechanical milling techniques wherein the starting materials, such as MAX phases or their precursors, are combined with a suitable etchant and milled together. The mechanical forces generated during milling facilitate the removal of the A element, leading to the formation of MXenes,60 and offer several advantages for MXene production (Table 1). It provides a scalable and efficient route to synthesize MXenes, eliminating the need for harsh chemical reactions or high-temperature treatments.61 For instance, Ljubek et al.61 introduced a fluorine-free mechanochemical technique for fabrication of Ti3C2Tx from Ti3AlC2. Moreover, mechanochemistry allows for the precise control of the degree of delamination and exfoliation, which directly impacts the properties of the resulting MXenes.62 In one study, an MXene (Ti3C2Tx) was prepared through a combination of ball milling and chemical etching using Ti3AlC2 powder.63 The investigation of the electrochemical performance of the sample, which underwent 6 h of mechanochemical treatment and 12 h of chemical etching, showed an electric double layer capacitance behavior with an enhanced specific capacitance of 146.3 F g−1 compared to samples treated for 24 and 48 h. Furthermore, the MXene sample exhibited increased specific capacitance during a 5000-cycle stability test owing to the termination of the –OH group, intercalation of K+ ions, and transformation into a TiO2/Ti3C2 hybrid structure in KOH electrolyte (3 M). Notably, the ball milling pre-treated MXene displayed excellent performance and stability due to the increased interlayer distance between the MXene sheets and the intercalation and deintercalation of Li+ ions.63
Table 1 Selected examples of mechanochemical synthesis methods of MXene-based materials and their applications
MXene-based materials |
Technique |
Applications |
Advantages/properties |
Ref. |
Pt/Nb2CTx MXene composites |
Simple mechanochemical ball milling and annealing strategy |
Electrocatalytic hydrogen evolution reaction |
• High electrochemical hydrogen evolution reaction activity |
46
|
• High stability with remarkable durability |
Ti3C2Tx MXene |
A combination of ball milling and chemical etching from Ti3AlC2 powder |
High-performance supercapacitors |
• Enhanced specific capacitance of 146.3 F g−1 |
63
|
• Stability over 5000 cycles |
• High energy (138.33 W h kg−1) and power density (1500 W kg−1) |
MXene (Ti3C2Tx)/TiO2 heterostructure |
A solvent mechanochemical technique (in situ fabrication) |
Sodium (Na)-ion storage |
• Improved stability of the electrode structure |
64
|
• Excellent electrode capacity (660 mA h g−1) |
Metal–organic-frameworks/MXene-derived carbonaceous metal selenide/MXene composites |
A solvent-free mechanochemical technique (a ball-milling methodology) |
Electrode materials for lithium (Li)/Na storage |
• Enhanced Li/Na ion storage properties |
65
|
• Low-cost, environmentally friendly and readily scalable synthesis technique |
Few-layered MXene (Ti3C2Tx) nanodot composites |
A red-phosphorus-assisted ball-milling synthesis |
Na-ion battery anode material |
• Good sodiation capacity (≈600 mA h g−1) at 260 mA g−1 |
66
|
• Improved cycle stability for 150 cycles |
Fluorine-free porous MXene (Ti3C2) |
A fluorine-free chemical-combined ball-milling technique |
Li-ion batteries |
• Enhanced specific surface area |
67
|
• Excellent electrochemical performance |
• Excellent reversible capacity (310 mA h g−1) at a current density of 100 mA g−1 |
• A reversible capacity of 97 mA h g−1 could be steadily maintained after cycling for 3000 cycles at a high current density of 1 A g−1 |
An efficient one-step mechanical exfoliation method has been developed for the rapid and high-yielding synthesis of MXenes (Ti3C2) as depicted in Fig. 2,68 which significantly reduced the preparation time compared to conventional methods. The ensuing MXenes exhibited excellent stability as colloidal dispersions in aqueous solutions and could disperse well in seven different organic solvents for extended periods of over 30 days. Moreover, the as-synthesized MXene materials showed the ability to efficiently accommodate diverse nanoparticles at ambient temperature, leading to a 100% reduction of p-nitrophenol in just 10 min.68
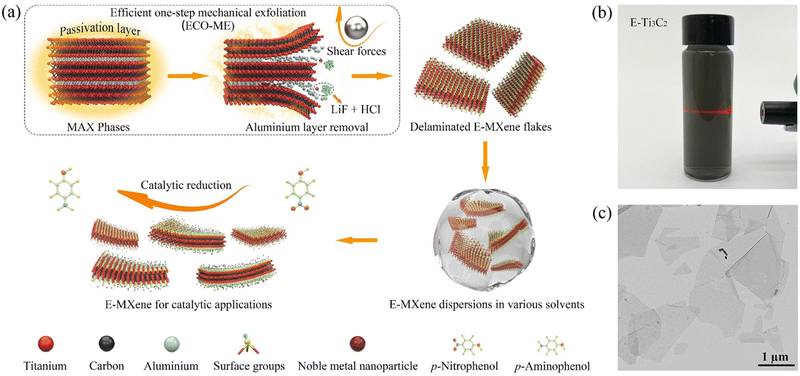 |
| Fig. 2 (a) The preparative process of MXenes utilizing the efficient one-step mechanical exfoliation technique. The diagram illustrates the synthetic pathway to MXenes and their catalytic applications. (b) A photographic image showing the stable colloidal MXene aqueous solution. (c) A transmission electron microscopy (TEM) image displaying the as-prepared MXene nanosheets. Reproduced with permission from ref. 68. Copyright 2023 Elsevier. | |
Mechanochemical synthesis can be employed to develop MXene-based materials for energy storage and conversion applications where ball milling can facilitate the preparation of MXene electrodes with enhanced electrochemical performance in supercapacitors or batteries.65,69 Shi et al.65 introduced a unique technique for synthesizing metal–organic-framework/MXene-derived carbonaceous metal selenide/MXene composites, devoid of any solvent usage. As a result, these composites exhibited distinct nanostructures enriched with mesopores and macropores. Through this approach, electrode materials with superior Li/Na ion storage properties could be attained compared to their solution-based counterparts. The mechanochemically synthesized composites displayed exceptional performance in advanced lithium/sodium storage applications.65 High-energy ball milling of precursors can facilitate the exfoliation and delamination of MAX phases, leading to the production of MXenes as shown for the fabrication of the Ti3AlC2 MAX phase in the Ti–Al–C system.70 The objective was to examine the effects of varying milling times, up to a maximum of 10 h, on mixtures of Ti, Al, and C with stoichiometric ratios. Thorough thermodynamic evaluation revealed highly exothermic reactions between the elemental powders of titanium, aluminum, and carbon, leading to the formation of the desired product phases in a self-sustaining regime. Accordingly, experimental findings demonstrated that, following 10 h of milling, the elemental powders underwent a rapid combustion reaction, resulting in the formation of Ti3AlC2 and TiC. These outcomes conclusively established that the dominant mechanism at play in this process was the mechanically induced self-propagating reaction.70 Mechanochemical methods offer great opportunities for scalable and cost-effective synthesis of MXenes, enabling the large-scale production of these materials. However, it is imperative to conduct further comprehensive studies in order to thoroughly explore and analyze the underlying mechanisms and optimization techniques.
2.2. Recent advancements
The fusion of MXenes and mechanochemistry offers great opportunities for synthesis, functionalization, and applications in the domain of materials science50,71 as MXene-based composites exhibit high electrical conductivity, unique optical/thermal properties, excellent mechanical properties, and good chemical stability.72–75 These attributes make them promising candidates for energy storage and conversion devices, including batteries, supercapacitors, and fuel cells, as MXenes can be engineered to enhance their electrochemical performance, such as increased capacitance and improved cycling stability. In addition, MXenes have shown potential in various other fields, including catalysis, electromagnetic shielding, sensing, and water purification. Mechanochemistry enables the facile integration of MXenes into composites or hybrid materials, further expanding their application prospects.50,76
Mechanochemistry offers an avenue for precisely controlling the synthesis parameters, such as milling time, milling speed, and precursor compositions, to achieve desired MXene properties. By elucidating these mechanisms, researchers can explore new avenues for optimizing mechanochemical synthesis strategies, enabling the development of MXenes with unprecedented properties. Applying mechanochemical strategies to MXene research can lead to the development of novel MXene-based materials with adjusted features and improved performance. Thus, the synergy among MXenes and mechanochemistry tactics holds great potential for advancements in various fields, including energy storage, catalysis, electronics, and beyond.77,78 For instance, strain modulation is a technique that holds great promise in the field of electrochemical materials. By adjusting the characteristics of these materials, strain modulation allows for developing mechano-electrochemical coupling, which in turn enables high-performance energy storage. MXenes due to their exceptional conductivity, mechanical properties, and interlayer spacing for ion intercalation have shown enormous potential for lithium storage applications. However, a persistent issue with MXene-based electrodes is the sluggish kinetics caused by the restacking of MXene nanosheets, a problem that has yet to be effectively addressed.77 In one study, Wang et al.77 developed an innovative solution to tackle the issue of slow kinetics in MXene-based electrodes (Fig. 3) by creating strain in an MXene (Ti3C2Tx) via mechanochemical means. By subjecting the MXene to mechanical forces, they induced out-of-plane tension and in-plane biaxial compression, leading to a comprehensive assessment of the strain states in the MXene. Specifically, the mechanochemically induced strained MXene (Ti3C2Tx) exhibited a notable decrease in the lithium diffusion barrier, resulting in enhanced ion-transfer kinetics where the strained MXene electrode displayed a high discharge capacity of 380.5 mA h g−1 at 0.1 A g−1, outperforming earlier documented unstrained Ti3C2Tx electrodes. Additionally, this electrode exhibited superior rate capability and exceptional lithium storage performance across a wide temperature range, from 40 °C to −20 °C. This work not only addressed the issue of sluggish kinetics in MXene-based electrodes but also established a novel approach for strain engineering of MXenes. By harnessing the power of mechanochemistry, the diffusion kinetics of lithium ions in lithium-ion batteries can be effectively enhanced. These findings have significant implications for developing high-performance energy storage systems and pave the way for further advancements in the field of MXene-based materials.77
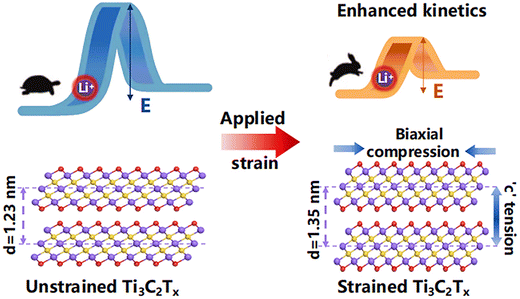 |
| Fig. 3 Biaxial compressive strain engineering for enhancing lithium storage kinetics in MXene electrodes using a mechanochemical method. Reproduced with permission from ref. 77. Copyright 2021 Elsevier. | |
Mechanochemistry can be employed for functionalizing MXenes by introducing desired surface modifications.62,79 By incorporating suitable reagents during ball milling, functional groups can be introduced onto the MXene surface, altering its properties and enabling tailored applications. This surface functionalization can enhance the compatibility of MXenes with other materials deployed in composites or facilitate the targeted interactions in catalytic or sensing applications where mechanochemical protocols are employed to fabricate MXene-based composites. By ball milling MXenes with polymers, metals, or other nanomaterials, the dispersion and interfacial interactions within the composite can be improved. This approach allows for the incorporation of MXenes into various matrices, thus enhancing the electrical, mechanical, or catalytic properties of the resulting composites.46,60,63 In a particular study focused on improving lithium ion storage capacity, researchers reported the development of a stable Ti–O–P bond-infused MXene (Ti3C2Tx)/carbon nanotubes@red phosphorus nanohybrid.79 To produce a conductive network, carbon nanotubes were incorporated into MXenes, which were then combined with red phosphorus through a ball-milling process. During this procedure, the surface oxygen-containing functional groups of MXene interacted with red phosphorus, resulting in the formation of Ti–O–P bonds. The nanohybrid showed exceptional performances due to the significant capacity contribution of red phosphorus, the conductive network and buffering capabilities of MXene/carbon nanotubes, and the impact of the Ti–O–P bond. It exhibited a remarkable reversible capacity of 2598 mA h g−1 at 0.05C (1C = 2600 mA g−1, based on the active material red phosphorus), enhanced cycling stability (2078 mA h g−1 after 500 cycles), and excellent rate capability (454 mA h g−1 at 30C). These findings indicated the captivating potential of the MXene-based nanohybrids for designing lithium-ion batteries with high performances.79 Wang et al.80 developed a plasma-assisted mechanochemical approach to introduce defects in an MXene (Ti3C2Tx), resulting in enhanced lithium ion storage performance when deployed as an anode in lithium-ion batteries (Fig. 4). Following the plasma-assisted mechanochemical treatment, the layer structure of the MXene became distorted, and a plethora of defects served as additional active sites for lithium ion storage. Consequently, the plasma-assisted mechanochemically treated MXene exhibited enhanced cycling stability, improved lithium storage capacity (242 mA h g−1 at a current density of 100 mA g−1), and high rate performance (100 mA h g−1 at 5 A g−1). This strategy can open up newer pathways to enhance the electrochemical energy storage capabilities of MXene-based electrode composites.80
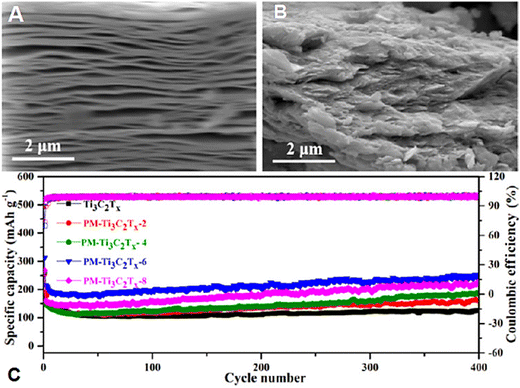 |
| Fig. 4 Scanning electrode microscopy (SEM) images of (A) an MXene (Ti3C2Tx) and (B) a plasma-assisted mechanochemically treated-MXene (PM-Ti3C2Tx). (C) Cycling performance of an MXene (Ti3C2Tx) and a PM-MXene (Ti3C2Tx) at 100 mA g−1. Reproduced with permission from ref. 80. Copyright 2021 American Chemical Society. | |
The poor realistic capacity and low rate performance of hard carbon have limited its practical application as an anode material in sodium-ion batteries.64 However, in one study, Gao et al.64 proposed a solvent mechanochemical technique to address these challenges. By functionalizing MXenes, the electrochemical performance of the batteries could be enhanced through the in situ production of the hard carbon–MXene/TiO2 electrodes (Fig. 5). During the ball milling process, MXenes (Ti3C2Tx) with oxygen-containing functional groups reacted with hard carbon particles, forming Ti–O–C covalent cross-linked hard carbon–MXene composites. Remarkably, the air oxidation of the MXene nanosheets at the edges resulted in the formation of TiO2 nanorods, creating a regular MXene/TiO2 heterojunction structure. This Ti–O–C covalent bonding played a crucial role in protecting the heterojunction structures from pulverization and detachment from the current collector during the charge/discharge cycles, which were caused by sodium ion intercalation/detachment. Consequently, the stability of the electrode structure was significantly improved. Furthermore, the heterojunction facilitated the formation of a three-dimensional (3D) conductive network, providing more active sites for electrochemical reactions. Ultimately, the resulting electrode demonstrated superior electrode capacity, thus positioning it as a promising anode material for sodium-ion batteries.64 This innovative approach of preparing MXene/TiO2 heterojunction-decorated hard carbon offers a fresh perspective on the structural design of MXenes and carbon material composites for sodium-ion batteries.
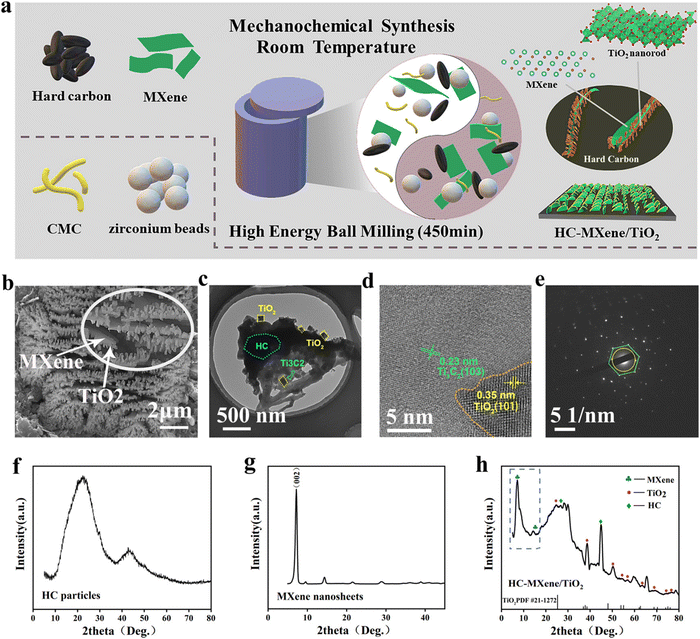 |
| Fig. 5 (a) A mechanochemical approach to synthesize hard carbon (HC)–MXene/TiO2 electrodes utilizing Ti3C2 as a multifunctional conductive agent. (b) SEM was utilized to examine the morphology of the flowerlike MXene/TiO2 heterostructures. (c) Transmission electron microscopy (TEM) provided a detailed view of the heterostructures at the nanoscale. (d) High-resolution transmission electron microscopy (HR-TEM) images were obtained to study the atomic-level structure of the MXene/TiO2 heterostructures. (e) Selected area electron diffraction (SAED) pattern analysis was performed on the heterostructures to gain insights into their crystallographic properties. (f) X-ray diffraction (XRD) patterns were recorded for HC particles to determine their crystalline structure. (g) MXene nanosheets were analyzed using XRD to assess their crystallographic characteristics. (h) XRD analysis was conducted on the electrode to investigate its structural properties. Reproduced with permission from ref. 64. Copyright 2021 American Chemical Society. | |
MXenes and their composites have been extensively studied for their adsorption and degradation properties in the removal of a wide range of contaminants namely toxic metals, inorganic ions, radionuclides, as well as organic pollutants like dyes, pharmaceuticals, aromatic hydrocarbons, and pesticides.81,82 An innovative MXene-based adsorbent was developed using a facile mechanochemical method to effectively remove organic contaminants from wastewater.60 One of the key advantages of this material was its high specific surface area (135.7 m2 g−1), allowing for enhanced adsorption capability, particularly for methylene blue, with an adsorption capacity of 209 mg g−1. This excellent performance could be attributed to the increased interlayer space among the MXene sheets and the presence of free carboxylate groups from the terephthalate. Furthermore, it was uncovered that the spent adsorbent could be effectively recouped by simply adding aluminum to the mechanochemical procedure. This reutilization process facilitated the synthesis of the MAX phase, where both the terephthalate and the pollutant were carbonized into carbides. This exciting finding enabled the resynthesis of MXene-based adsorbents for subsequent use, thereby enhancing the sustainability and cost-effectiveness of the overall process.60 Li et al. evaluated an asymmetric interface derived from the edge-rich defective Ti3C2Tx (ERDT) to expose unsaturated coordinated active titanium atoms through mechanochemical treatment (Fig. 6), which resulted in a stepped cross-section structure that significantly enhanced the degradation rate constant of carbamazepine in the ERDT/peroxymonosulfate system, exhibiting a value of 0.326 min−1. Comparatively, the degradation rate constant for carbamazepine in the bulk MXene was only 0.003 min−1, which was 108.9 times lower. The findings indicated that ERDT played a crucial role in initiating both free radical and non-radical titanium metal oxide species pathways, contributing 51% and 48% to carbamazepine degradation, respectively. Furthermore, in the MXene/peroxymonosulfate system, the Bader charge of the metastable Ti–OH remained nearly constant, while in the ERDT/peroxymonosulfate system, the metastable Ti–O–Ti intermediate decreased by 0.413e−, resulting in the formation of surface-bound oxygen species (Ti(III)–O*–Ti(III)). This suggests that ERDT, without hydroxyl capping, was more inclined to generate HSO4− ions and create a Ti(III)–O*–Ti(III) poor-electron region. Consequently, the system exhibited greater electron transfer from peroxymonosulfate and carbamazepine. Combined with the presence of edge-defective active sites under mechanical shearing force, this led to a stronger adsorption affinity between peroxymonosulfate and ERDT, thereby enhancing the catalytic performance for the degradation of target emerging contaminants.
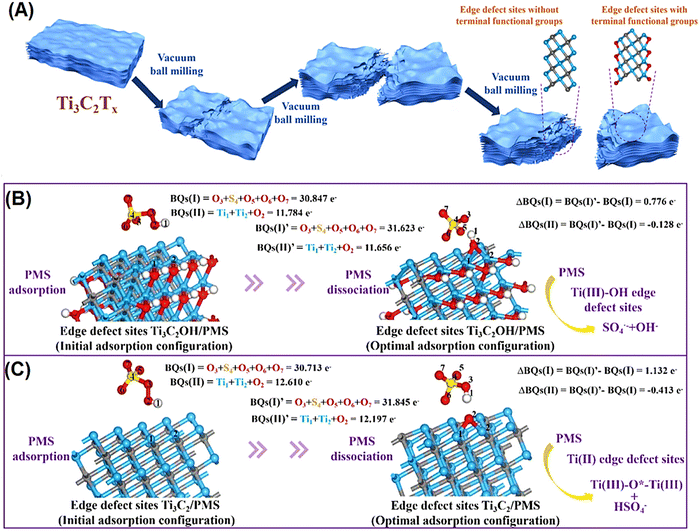 |
| Fig. 6 (A) A visual representation showing the synthetic pathway for a bulk MXene (Ti3C2Tx) and edge-rich defective Ti3C2Tx is presented. (B) The (010) Ti3C2OH/peroxymonosulfate (PMS) system along with its corresponding Bader charge. (C) The (010) Ti3C2/PMS system and its corresponding Bader charge. Reproduced with permission from ref. 83. Copyright 2023 American Chemical Society. | |
3. Challenges
While mechanochemistry has enabled significant advancements in MXene synthesis, there are still challenges that need to be addressed, especially the scalability of mechanochemical processes to produce MXenes in large quantities. In addition, understanding the underlying mechanistic details of formation of MXenes through mechanical forces is essential for further optimization and control of their properties. Looking ahead, the combination of mechanochemistry and MXenes holds great potential for developing advanced materials with controllable properties. Additional explorations in mechanochemical synthesis techniques will pave the way for the exploration of new MXene derivatives and their applications in diverse fields.
While mechanochemical synthesis offers numerous advantages, the repeated impact and grinding between milling balls and the reactant particles can introduce impurities from the milling media, limiting the purity of the synthesized materials. Notably, mechanochemical synthesis can generate heat, leading to increased local temperature, which can induce unwanted side reactions or affect the stability of the created materials. Scaling up mechanochemical synthesis for industrial applications can be challenging due to issues related to equipment design, handling of larger quantities of materials, and ensuring reproducibility. Controlling the selectivity of mechanochemical reactions can be difficult, as multiple competing reactions can occur simultaneously. A thorough optimization of milling parameters is therefore necessary to achieve the desired products with high selectivity.
Overall, the important challenges associated with MXenes and mechanochemistry involve controlling contamination during synthesis and developing methods to enhance the purity and consistency of MXene products, as well as optimizing the mechanochemical processes to ensure efficient and reliable production. Some of the key challenges and limitations that need to be addressed are summarized as follows:
3.1. Scalability
One of the primary challenges with MXenes and their derivatives is achieving large-scale production. The current synthesis methods often involve harsh etching conditions and low yields, limiting their commercial viability.39,72,84 Developing scalable and cost-effective synthesis strategies is crucial for the widespread implementation of MXenes in various applications. For instance, the fabrication of MXenes using hydrofluoric (HF) acid etching has been extensively documented.85,86 However, the high acute toxicity associated with HF acid poses significant challenges for large-scale production of MXenes and their widespread use in energy-related applications.87 Therefore, it is essential to investigate safer methods for synthesizing MXenes, which would facilitate their mass production and broaden their applicability. In one study, a novel approach utilizing thermal-assisted electrochemical etching has been developed to synthesize MXenes such as Ti2CTx, Cr2CTx, and V2CTx. This investigation demonstrated the potential of MXenes in various applications while offering a nontoxic and HF-free synthesis route for these materials.87 Scaling up the mechanochemical synthesis for industrial applications can be challenging. The design of equipment capable of handling large quantities of materials and also the preservation of effective milling conditions are two important factors. Besides, ensuring the scalability and reproducibility of mechanochemical reactions on a larger scale is a complex task that requires careful optimization and consideration of process parameters.
3.2. Stability
MXenes are susceptible to environmental conditions, such as exposure to moisture or high temperatures, and their stability can be influenced by factors such as environmental conditions, chemical interactions, and structural changes. Ensuring their stability over time is essential for their long-term performance and practical applications.88,89 Notably, in comparison to MXenes in a free-standing film configuration, their aqueous dispersion form exhibits a significantly higher oxidation rate. Although the precise mechanism by which MXenes oxidize in their aqueous state is currently a topic of debate, previous research on Ti3C2Tx MXenes suggests that oxygen and water play a complex role in initiating oxidation. This investigation eventually pinpointed water as the primary factor responsible for Ti3C2Tx hydrolysis. Numerous studies have examined the oxidation of MXenes, particularly Ti3C2Tx, under various storage conditions and have identified several parameters that influence the rate of oxidation, which include the pH of the dispersion, as well as the nature, size, and concentration of MXenes.90 Additional explorations are warranted to enhance the stability of MXenes through surface modifications or protective coatings. MXenes are also susceptible to reactions with certain chemicals, such as strong acids or oxidizing agents, which can lead to structural damage and loss of functionality. It is essential to avoid exposure to corrosive substances to maintain the stability of MXenes. Furthermore, structural changes can occur in MXenes under specific conditions, affecting their stability and they may include delamination, restacking, or phase transitions, which can alter their properties and performance. Understanding the underlying mechanisms behind these structural changes is crucial for designing MXene-based materials with improved stability and various strategies have been explored to enhance the stability of MXenes. Surface modifications, such as functionalization with polymers or inorganic materials, can be employed to enhance their chemical and thermal stability. In addition, the manufacturing processes of MXenes/derivatives ought to be optimized to minimize structural changes and ensure long-term stability.89
3.3. Contamination and environmental impact
Controlling and minimizing contamination during MXene synthesis is a significant challenge.91 The milling media and equipment used in the synthesis process can introduce impurities, affecting the purity and consistency of the final MXene products. Developing methods to minimize contamination and improve the purity of MXenes is necessary for their reliable characterization and successful integration into various applications. By implementing stringent protocols and employing high-quality milling media and equipment, researchers can mitigate the risk of contamination and ensure the production of pristine MXene materials. Furthermore, the development of advanced purification techniques and thorough characterization methods can aid in identifying and eliminating any residual impurities. This comprehensive approach is essential for obtaining MXene samples with consistent quality, enabling accurate and reliable experimental results.
3.4. Dispersion and compatibility
Achieving uniform dispersion of MXenes within composite materials can be a formidable task due to their inherently hydrophilic nature. To address this issue, various methods have been explored, such as using polar organic solvents like N,N-dimethylformamide,92 optimizing the pH value of acidic dispersions, and utilizing raw materials that improve the stability and conductive properties of MXenes.93 MXenes tend to agglomerate in certain matrices, affecting the properties and performance of the composites. Therefore, developing effective strategies to improve the dispersion and compatibility of MXenes with different matrices is crucial for realizing their full potential in composite applications. There are several tactics that can be employed to address the limitations associated with MXene dispersion. One approach involves chemically modifying the surface of an MXene to enhance its hydrophobicity, thereby improving its compatibility with the matrix material. Another effective option is the assembly of MXene nanosheets into macrostructures, promoting a more uniform dispersion. Notably, the utilization of polymeric super-dispersants has shown promise in achieving broad dispersibility of MXene nanosheets in various solutions.94,95
3.5. Reproducibility
Achieving reproducibility in mechanochemical synthesis can be demanding. Variations in milling conditions, such as the ball-to-powder ratio, milling time, and rotational speed, can significantly impact the reaction outcomes. Standardizing the synthesis protocols and identifying the critical milling parameters for reproducibility are important for ensuring consistency in the synthesized materials.96 By adjusting parameters such as milling time, milling speed, and precursor compositions, researchers can adjust the properties of MXenes to meet specific application requirements. This level of control enables the production of MXenes and their derivatives with optimized properties for various applications, including energy storage, catalysis, robotics, biomedicine, environmental remediation, and electronics.
Overcoming these hurdles will pave the way for the successful implementation of MXenes and mechanochemical synthesis in various applications, thus unlocking their full potential for advancing materials science. Improved synthesis methods, such as scalable and eco-friendly approaches, will facilitate the large-scale fabrication of MXenes and their derivatives. Furthermore, advancements in MXene functionalization, hybridization, and composite design will lead to enhanced performance and expanded applications. The integration of MXenes with other emerging materials, such as graphene or carbon nanotubes, may develop novel functionalities and synergistic effects. Mechanochemical synthesis offers the opportunity to functionalize the surface of MXenes during the milling process. By introducing functional groups or incorporating dopants, researchers can modify the surface chemistry of MXenes, enhancing their stability, conductivity, or catalytic activity. This opens up avenues for designing MXenes with tailored functionalities, thus expanding their potential applications. Advances in mechanochemical synthesis techniques have led to a better understanding of the underlying mechanisms governing the transformation of precursor materials into MXenes. Through in-depth characterization and analysis, researchers can unravel the complex processes occurring during mechanochemical synthesis, which is crucial for optimizing synthesis strategies and further enhancing the properties and performance of MXenes.
4. Future perspectives
The future of MXenes and mechanochemistry is poised for exciting advancements and innovative applications across various scientific disciplines. As researchers delve deeper into the synergistic relationship between these two fields, several promising prospects emerge for the development of novel materials and technologies:
4.1. Enhanced functionalization and tunability
One of the key future prospects in the field of MXenes and mechanochemistry is the enhanced functionalization and tunability of MXene materials. By leveraging mechanochemical synthesis techniques, researchers can precisely tailor the surface chemistry and properties of MXenes to meet specific application requirements. This level of control opens up new avenues for designing MXene-based materials with advanced functionalities and performance characteristics.
4.2. Expanded applications in biomedical and environmental fields
The integration of MXenes and mechanochemistry holds immense potential for expanding applications in the biomedical and environmental fields. Mechanochemically synthesized MXenes can be engineered to exhibit biocompatibility, antimicrobial properties, and targeted drug delivery capabilities, making them promising candidates for biomedical implants, therapeutics, and biosensors. Additionally, MXenes produced through mechanochemical routes show promise for environmental applications such as water purification, pollutant remediation, and catalytic processes.
4.3. Scalability and industrial transferability
Another future prospect lies in the scalability and industrial transferability of mechanochemically synthesized MXenes. As researchers optimize synthesis protocols and scale-up processes, the production of MXene materials on a commercial scale becomes more feasible. This scalability paves the way for the widespread adoption of MXenes in various industries, ranging from electronics and energy storage to aerospace and automotive sectors.
4.4. Synthesis strategies
One prominent trend in MXenes and mechanochemistry is the exploration of novel synthesis strategies to enhance the efficiency and versatility of MXene materials. From mechanochemical exfoliation methods to bottom-up assembly techniques, researchers are constantly innovating to develop new pathways for synthesizing MXenes with tailored properties and functionalities. These cutting-edge approaches hold the potential to revolutionize the production of MXene-based materials for diverse applications. Notably, a growing focus on sustainability and green chemistry principles is also shaping the future of MXenes and mechanochemistry. Researchers are exploring eco-friendly synthesis routes that minimize waste generation, energy consumption, and environmental impact. By adopting sustainable practices in the production of MXene materials, the field aims to address global challenges while advancing towards a more sustainable and environmentally conscious future. Mechanochemical synthesis offers several advantages over traditional methods, making it an attractive approach in the materials science domain, and it can be applied to a wide range of materials, including metals, alloys, oxides, intermetallic compounds, and organics. This strategy can eliminate the need for complex reaction conditions, like high temperatures and pressures, thus reducing the cost and simplifying the synthesis process. It typically uses minimal or no solvents, leading to reduced chemical waste and diminished environmental impact compared to traditional solution-based synthesis methods. Notably, mechanical forces applied during ball milling can overcome kinetic barriers, enabling the synthesis of metastable compounds and facilitating reactions that are otherwise difficult to achieve. In addition, mechanochemical synthesis allows for precise control over the composition, phase, and crystallinity of the synthesized materials by adjusting milling parameters such as the milling time and speed and ball-to-powder ratio. This approach has been employed in green chemistry processes, such as solvent-free reactions, waste valorization, and the synthesis of biodegradable materials.97,98 It can be applied for the fabrication of nanoparticles, nanocomposites, and nanostructured materials with tailored properties for applications in electronics, catalysis, and sensing.57
4.5. Multifunctional MXene-based composites
Another trend gaining momentum is the development of multifunctional MXene-based composites through mechanochemical synthesis routes. By incorporating MXenes into various matrices and nanomaterials, researchers can create hybrid structures with synergistic properties, such as enhanced mechanical strength, conductivity, and catalytic activity. These composite materials show promise for applications in advanced electronics, energy storage, and environmental remediation.
5. Conclusion
MXenes and their composites have shown great potential in various applications, including energy storage, sensing, biomedical engineering, catalysis, soft robotics, and electronics. Their high surface area, metallic conductivity, and hydrophilicity make them suitable for supercapacitors and batteries, where they can enhance energy storage and charge/discharge rates. In addition, MXene-based composites can serve as efficient catalysts, enabling various chemical reactions with enhanced reaction rates and selectivity. The unique combination of properties offered by MXenes makes them promising candidates for developing advanced materials in the field of nanotechnology. Mechanochemistry is a fascinating part of chemistry that explores the use of mechanical energy to drive chemical reactions by applying mechanical forces, such as grinding, milling, or shearing, to initiate or accelerate chemical transformations. Mechanochemical reactions typically exhibit unique reaction pathways and product distributions compared to traditional solution-based reactions. This field has garnered significant attention in view of its sustainable and green chemistry attributes encompassing several advantages, including higher reaction rates, improved selectivity, and reduced waste generation. Moreover, mechanochemistry has shown promise in various applications, such as organic synthesis, pharmaceutical manufacturing, and materials science. By controlling the power of mechanical energy, mechanochemistry opens up new possibilities for the development of efficient and eco-friendly chemical reactions.
One of the crucial aspects in MXene synthesis is the scalability of the production process. While MXenes have shown exceptional properties in the laboratory, their large-scale synthesis and commercialization remain a complex task. Overcoming this challenge requires the development of efficient and cost-effective production methods that can be readily adopted on an industrial scale. The stability of MXenes in various environments is also an important aspect for their further practical applications; MXenes are prone to oxidation, which can degrade their properties over time. Researchers are actively working on developing strategies to enhance the stability of MXenes and improve their long-term performance. In terms of mechanochemistry, one of the challenges lies in understanding the underlying mechanisms of the reactions induced by mechanical forces. While mechanochemical synthesis has proven effective in producing new materials, the fundamental understanding of how mechanical energy influences chemical reactions is still evolving. Further research is needed to elucidate the intricate details of mechanochemical processes and optimize their efficiency. The advancements in MXene-based devices and technologies, contributing to the development of sustainable energy solutions, environmental remediation, and advanced electronics, are anticipated in the future, thus expanding the scope of mechanochemical reactions to new classes of compounds and leading to exploration of novel reaction pathways and optimization of the efficiency of mechanochemical processes.
Author contributions
Siavash Iravani: supervision, conceptualization, writing – reviewing and editing; Atefeh Zarepour: writing – reviewing and editing; Ehsan Nazarzadeh Zare: writing – reviewing and editing; Pooyan Makvandi: writing – reviewing and editing; Arezoo Khosravi: writing – reviewing and editing; Rajender S. Varma: writing – reviewing and editing; and Ali Zarrabi: supervision, writing – reviewing and editing.
Data availability
No data were used for the research described in this article.
Conflicts of interest
The author(s) declare no competing interest.
References
- Y. Zhang, Z. Feng, X. Wang, H. Hu and M. Wu, MXene/carbon composites for electrochemical energy storage and conversion, Mater. Today Sustainability, 2023, 22, 100350 CrossRef.
- J. Zou, J. Wu, Y. Wang, F. Deng, J. Jiang, Y. Zhang, S. Liu, N. Li, H. Zhang, J. Yu, T. Zhai and H. N. Alshareef, Additive-mediated intercalation and surface modification of MXenes, Chem. Soc. Rev., 2022, 51, 2972–2990 RSC.
- A. Rosenkranz, M. C. Righi, A. V. Sumant, B. Anasori and V. N. Mochalin, Perspectives of 2D MXene Tribology, Adv. Mater., 2023, 35, 2207757 CrossRef CAS PubMed.
- B. Anasori and Y. Gogotsi, MXenes: trends, growth, and future directions, Graphene 2D Mater., 2022, 7, 75–79 CrossRef.
- B. Anasori, M. R. Lukatskaya and Y. Gogotsi, 2D metal carbides and nitrides (MXenes) for energy storage, Nat. Rev. Mater., 2017, 2, 16098 CrossRef CAS.
- Z. Xiao, X. Xiao, L. B. Kong, H. Dong, X. Li, X. Sun, B. He, S. Ruan and J. Zhai, MXenes and MXene-based composites for energy conversion and storage applications, J. Materiomics, 2023, 9(6), 1067–1112 CrossRef.
- S. Iravani and R. S. Varma, MXene-based wearable supercapacitors and their transformative impact on healthcare, Mater. Adv., 2023, 4, 4317–4332 RSC.
- J. D. Gouveia, H. Rocha and J. R. B. Gomes, MXene-supported transition metal single-atom catalysts for nitrogen dissociation, Mol. Catal., 2023, 547, 113373 CrossRef CAS.
- J. K. Im, E. J. Sohn, S. Kim, M. Jang, A. Son, K.-D. Zoh and Y. Yoon, Review of MXene-based nanocomposites for photocatalysis, Chemosphere, 2021, 270, 129478 CrossRef CAS PubMed.
- W. Kong, J. Deng and L. Li, Recent advances in noble metal MXene-based catalysts for electrocatalysis, J. Mater. Chem. A, 2022, 10, 14674–14691 RSC.
- J. Wang, J. Jiang, F. Li, J. Zou, K. Xiang, H. Wang, Y. Li and X. Li, Emerging carbon-based quantum dots for sustainable photocatalysis, Green Chem., 2023, 25, 32–58 RSC.
- N. Arif, S. Gul, M. Sohail, S. Rizwan and M. Iqbal, Synthesis and characterization of layered Nb2C MXene/ZnS nanocomposites for highly selective electrochemical sensing of dopamine, Ceram. Int., 2021, 47, 2388–2396 CrossRef CAS.
- Z. U. D. Babar, B. D. Ventura, R. Velotta and V. Iannotti, Advances and emerging challenges in MXenes and their nanocomposites for biosensing applications, RSC Adv., 2022, 12, 19590–19610 RSC.
- V. Kedambaimoole, K. Harsh, K. Rajanna, P. Sen, M. M. Nayak and S. Kumar, MXene wearables: properties, fabrication strategies, sensing mechanism and applications, Mater. Adv., 2022, 3, 3784–3808 RSC.
- F. Shahzad, M. Alhabeb, C. B. Hatter, B. Anasori, S. M. Hong, C. M. Koo and Y. Gogotsi, Electromagnetic interference shielding with 2D transition metal carbides (MXenes), Science, 2016, 353, 1137–1140 CrossRef CAS PubMed.
- A. Iqbal, J. Kwon, M.-K. Kim and C. M. Koo, MXenes for electromagnetic interference shielding: experimental and theoretical perspectives, Mater. Today Adv., 2021, 9, 100124 CrossRef CAS.
- K. Hantanasirisakul, M.-Q. Zhao, P. Urbankowski, J. Halim, B. Anasori, S. Kota, C. E. Ren, M. W. Barsoum and Y. Gogotsi, Fabrication of Ti3C2Tx MXene Transparent Thin Films with Tunable Optoelectronic Properties, Adv. Electron. Mater., 2016, 2, 1600050 CrossRef.
- A. Sarycheva, A. Polemi, Y. Liu, K. Dandekar, B. Anasori and Y. Gogotsi, 2D titanium carbide (MXene) for wireless communication, Sci. Adv., 2018, 4(9), 1–9 Search PubMed.
- Y. Dong, S. S. K. Mallineni, K. Maleski, H. Behlow, V. N. Mochalin, A. M. Rao, Y. Gogotsi and R. Podila, Metallic MXenes: a new family of materials for flexible triboelectric nanogenerators, Nano Energy, 2018, 44, 103–110 CrossRef CAS.
- M. Khatami and S. Iravani, MXenes and MXene-based Materials for the Removal of Water Pollutants: Challenges and Opportunities, Comments Inorg. Chem., 2021, 41, 213–248 CrossRef CAS.
- X. Zhan, C. Si, J. Zhou and Z. Sun, MXene and MXene-based composites: synthesis, properties and environment-related applications, Nanoscale Horiz., 2020, 5, 235–258 RSC.
- M. G. Rasul, A. Kiziltas, B. Arfaei and R. Shahbazian-Yassar, 2D boron nitride nanosheets for polymer composite materials, npj 2D Mater. Appl., 2021, 5, 56 CrossRef CAS.
- M. Hu, H. Zhang, T. Hu, B. Fan, X. Wang and Z. Li, Emerging 2D MXenes for supercapacitors: status, challenges and prospects, Chem. Soc. Rev., 2020, 49, 6666–6693 RSC.
- A. K. Tareen, K. Khan, M. Iqbal, Y. Zhang, J. Long, A. Mahmood, N. Mahmood, Z. Xie, C. Li and H. Zhang, Recent advance in two-dimensional MXenes: new horizons in flexible batteries and supercapacitors technologies, Energy Storage Mater., 2022, 53, 783–826 CrossRef.
- X. Xu, L. Yang, W. Zheng, H. Zhang, F. Wu, Z. Tian, P. Zhang and Z. M. Sun, MXenes with applications in supercapacitors and secondary batteries: a comprehensive review, Mater. Rep.: Energy, 2022, 2, 100080 CAS.
- J. Jiang, Y. Zou, A. Arramel, F. Li, J. Wang, J. Zou and N. Li, Intercalation engineering of MXenes towards highly efficient photo(electrocatalytic) hydrogen evolution reactions, J. Mater. Chem. A, 2021, 9, 24195–24214 RSC.
- S. Verma, U. Dwivedi, K. Chaturvedi, N. Kumari, M. Dhangar, S. A. R. Hashmi, R. Singhal and A. K. Srivastava, Progress of 2D MXenes based composites for efficient electromagnetic interference shielding applications: a review, Synth. Met., 2022, 287, 117095 CrossRef CAS.
- Z. Wang, Z. Cheng, C. Fang, X. Hou and L. Xie, Recent advances in MXenes composites for electromagnetic interference shielding and microwave absorption, Composites, Part A, 2020, 136, 105956 CrossRef CAS.
- C. C. Leong, Y. Qu, Y. Kawazoe, S. K. Ho and H. Pan, MXenes: novel electrocatalysts for hydrogen production and nitrogen reduction, Catal. Today, 2021, 370, 2–13 CrossRef CAS.
- Y. Sun, X. Meng, Y. Dall'Agnese, C. Dall'Agnese, S. Duan, Y. Gao, G. Chen and X.-F. Wang, 2D MXenes as co-catalysts in photocatalysis: synthetic methods, Nano-Micro Lett., 2019, 11, 1–22 CrossRef CAS PubMed.
- C. Prasad, X. Yang, Q. Liu, H. Tang, A. Rammohan, S. Zulfiqar, G. V. Zyryanov and S. Shah, Recent advances in MXenes supported semiconductors based photocatalysts: properties, synthesis and photocatalytic applications, J. Ind. Eng. Chem., 2020, 85, 1–33 CrossRef CAS.
- V. Shankar, S. P. Dharani, A. Ravi and A. SaravanaVadivu, A concise review: MXene-based photo catalytic and photo electrochemical water splitting reactions for the production of hydrogen, Int. J. Hydrogen Energy, 2023, 48, 21654–21673 CrossRef CAS.
- T. Amrillah, A. R. Supandi, V. Puspasari, A. Hermawan and Z. W. Seh, MXene-Based Photocatalysts and Electrocatalysts for CO2 Conversion to Chemicals, Trans. Tianjin Univ., 2022, 28, 307–322 CrossRef CAS.
- N. Kitchamsetti and A. L. F. de Barros, Recent Advances in MXenes Based Composites as Photocatalysts: Synthesis, Properties and Photocatalytic Removal of Organic Contaminants from Wastewater, ChemCatChem, 2023, 15, e202300690 CrossRef CAS.
- B. C. Wyatt, S. K. Nemani and B. Anasori, 2D transition metal carbides (MXenes) in metal and ceramic matrix composites, Nano Converge., 2021, 8, 16 CrossRef CAS PubMed.
- M. S. Saharudin, A. Ayub, S. Hasbi, F. Muhammad-Sukki, I. Shyha and F. Inam, Recent advances in MXene composites research, applications and opportunities, Mater. Today: Proc., 2023 DOI:10.1016/j.matpr.2023.02.435.
- F. M. Oliveira, J. Azadmanjiri, X. Wang, M. Yu and Z. Sofer, Structure Design and Processing Strategies of MXene-Based Materials for Electromagnetic Interference Shielding, Small Methods, 2023, 7, 2300112 CrossRef CAS PubMed.
- A. B. Talipova, V. V. Buranych, I. S. Savitskaya, O. V. Bondar, A. Turlybekuly and A. D. Pogrebnjak, Synthesis, Properties, and Applications of Nanocomposite Materials Based on Bacterial Cellulose and MXene, Polymers, 2023, 15, 4067 CrossRef CAS PubMed.
- S. Iravani, MXenes and MXene-based (nano)structures: a perspective on greener synthesis and biomedical prospects, Ceram. Int., 2022, 48, 24144–24156 CrossRef CAS.
- A. Iqbal, J. Hong, T. Y. Ko and C. M. Koo, Improving oxidation stability of 2D MXenes: synthesis, storage media, and conditions, Nano Converge., 2021, 8, 1–24 CrossRef PubMed.
- X. Li, X. Ma, H. Zhang, N. Xue, Q. Yao, T. He, Y. Qu, J. Zhang and X. Tao, Ambient-stable MXene with superior performance suitable for widespread applications, Chem. Eng. J., 2023, 455, 140635 CrossRef CAS.
- J.-L. Do and T. Friščić, Mechanochemistry: A Force of Synthesis, ACS Cent. Sci., 2017, 3, 13–19 CrossRef CAS PubMed.
- J. A. Leitch and D. L. Browne, Mechanoredox Chemistry as an Emerging Strategy in Synthesis, Chem. – Eur. J., 2021, 27, 9721–9726 CrossRef CAS PubMed.
- J. L. Howard, Q. Cao and D. L. Browne, Mechanochemistry as an emerging tool for molecular synthesis: what can it offer?, Chem. Sci., 2018, 9, 3080–3094 RSC.
- S. Kumar, N. Kumari and Y. Seo, MXenes: versatile 2D materials with tailored surface chemistry and diverse applications, J. Energy Chem., 2024, 90, 253–293 CrossRef CAS.
- X. Fan, P. Du, X. Ma, R. Wang, J. Ma, Y. Wang, D. Fan, Y. Long, B. Deng, K. Huang and H. Wu, Mechanochemical Synthesis of Pt/Nb2CTx MXene Composites for Enhanced Electrocatalytic Hydrogen Evolution, Materials, 2021, 14, 2426 CrossRef CAS PubMed.
- M. Kandeel, A. Turki Jalil, M. Hadi Lafta, S. Ziyadullaev and Y. F. Mustafa, Recent progress in synthesis and applications of MXene-based nanomaterials (MBNs) for (bio)sensing of microbial toxins, pathogenic bacteria in food matrices, Microchem. J., 2022, 183, 108121 CrossRef CAS.
- J. A. Kumar, P. Prakash, T. Krithiga, D. J. Amarnath, J. Premkumar, N. Rajamohan, Y. Vasseghian, P. Saravanan and M. Rajasimman, Methods of synthesis, characteristics, and environmental applications of MXene: a comprehensive review, Chemosphere, 2022, 286, 131607 CrossRef CAS PubMed.
- I. A. Vasyukova, O. V. Zakharova, D. V. Kuznetsov and A. A. Gusev, Synthesis, toxicity assessment, environmental and biomedical applications of MXenes: a review, Nanomaterials, 2022, 12, 1797 CrossRef CAS PubMed.
- U. Noor, M. F. Mughal, T. Ahmed, M. F. Farid, M. Ammar, U. Kulsum, A. Saleem, M. Naeem, A. Khan, A. Sharif and K. Waqar, Synthesis and applications of MXene-based composites: a review, Nanotechnology, 2023, 34, 262001 CrossRef PubMed.
- R. Dubadi, S. D. Huang and M. Jaroniec, Mechanochemical Synthesis of Nanoparticles for Potential Antimicrobial Applications, Materials, 2023, 16, 1460 CrossRef CAS PubMed.
- V. Martinez, T. Stolar, B. Karadeniz, I. Brekalo and K. Užarević, Advancing mechanochemical synthesis by combining milling with different energy sources, Nat. Rev. Chem., 2023, 7, 51–65 CrossRef CAS PubMed.
- D. Chen, J. Zhao, P. Zhang and S. Dai, Mechanochemical synthesis of metal–organic frameworks, Polyhedron, 2019, 162, 59–64 CrossRef CAS.
- S. Głowniak, B. Szczęśniak, J. Choma and M. Jaroniec, Mechanochemistry: toward green synthesis of metal–organic frameworks, Mater. Today, 2021, 46, 109–124 CrossRef.
- M. Kumar, X. Xiong, Z. Wan, Y. Sun, D. C. W. Tsang, J. Gupta, B. Gao, X. Cao, J. Tang and Y. S. Ok, Ball milling as a mechanochemical technology for fabrication of novel biochar nanomaterials, Bioresour. Technol., 2020, 312, 123613 CrossRef CAS PubMed.
- R. Takahashi, A. Hu, P. Gao, Y. Gao, Y. Pang, T. Seo, J. Jiang, S. Maeda, H. Takaya, K. Kubota and H. Ito, Mechanochemical synthesis of magnesium-based carbon nucleophiles in air and their use in organic synthesis, Nat. Commun., 2021, 12, 6691 CrossRef CAS PubMed.
- T. Tsuzuki, Mechanochemical synthesis of metal oxide nanoparticles, Commun. Chem., 2021, 4, 143 CrossRef CAS PubMed.
- S. L. James, C. J. Adams, C. Bolm, D. Braga, P. Collier, T. Friščić, F. Grepioni, K. D. M. Harris, G. Hyett, W. Jones, A. Krebs, J. Mack, L. Maini, A. G. Orpen, I. P. Parkin, W. C. Shearouse, J. W. Steedk and D. C. Waddelli, Mechanochemistry: opportunities for new and cleaner synthesis, Chem. Soc. Rev., 2012, 41, 413–447 RSC.
- V. K. Singh, A. Chamberlain-Clay, H. C. Ong, F. León, G. Hum, M. Yang Par, P. Daley-Dee and F. García, Multigram Mechanochemical synthesis of a Salophen Complex: A Comparative Analysis, ACS Sustainable Chem. Eng., 2021, 9, 1152–1160 CrossRef CAS.
- M. Vakili, G. Cagnetta, J. Huang, G. Yu and J. Yuan, Synthesis and Regeneration of A MXene-Based Pollutant Adsorbent by Mechanochemical Methods, Molecules, 2019, 24, 2478 CrossRef CAS PubMed.
- G. Ljubek, M. Kralj and M. K. Roković, Fluorine-free mechanochemical synthesis of MXene, Mater. Sci. Technol., 2023, 39, 1645–1649 CrossRef CAS.
- X. Liu, W. Kang, X. Li, L. Zeng, Y. Li, Q. Wang and C. Zhang, Solid-state mechanochemistry advancing two dimensional materials for lithium-ion storage applications: a mini review, Nano Mater. Sci., 2023, 5, 210–227 CrossRef CAS.
- I. Cho, A. R. Selvaraj, J. Bak, H. Kim and K. Prabakar, Mechanochemical Pretreated Mn+1AXn (MAX) Phase to Synthesize 2D-Ti3C2Tx MXene Sheets for High-Performance Supercapacitors, Nanomaterials, 2023, 13, 1741 CrossRef CAS PubMed.
- P. Gao, H. Shi, T. Ma, S. Liang, Y. Xia, Z. Xu, S. Wang, C. Min and L. Liu, MXene/TiO2 Heterostructure-Decorated Hard Carbon with Stable Ti–O–C Bonding for Enhanced Sodium-Ion Storage, ACS Appl. Mater. Interfaces, 2021, 13, 51028–51038 CrossRef CAS PubMed.
- X. Shi, W. Liang, G. Liu, B. Chen, L. Shao, Y. Wu, Z. Sun and F. García, Electrode materials for Li/Na storage from mechanochemically synthesised MOFs/MXene Composites: a Solvent-free approach, Chem. Eng. J., 2023, 462, 142271 CrossRef CAS.
- T. Zhang, X. Jiang, G. Li, Q. Yao, J. Y. Lee and A. Red-Phosphorous-Assisted Ball-Milling Synthesis, of Few-Layered Ti3C2Tx (MXene) Nanodot Composite, ChemNanoMat, 2018, 4, 56–60 CrossRef CAS.
- N. Xue, X. Li, M. Zhang, L. Han, Y. Liu and X. Tao, Chemical-Combined Ball-Milling Synthesis of Fluorine-Free Porous MXene for High-Performance Lithium Ion Batteries, ACS Appl. Energy Mater., 2020, 3, 10234–10241 CrossRef CAS.
- Y. Liu, Q. Tang, M. Xu, J. Ren, C. Guo, C. Chen, W. Geng, W. Lei, X. Zhao and D. Liu, Efficient mechanical exfoliation of MXene nanosheets, Chem. Eng. J., 2023, 468, 143439 CrossRef CAS.
- M. Q. Long, K. K. Tang, J. Xiao, J. Y. Li, J. Chen, H. Gao, W. H. Chen, C. T. Liu and H. Liu, Recent advances on MXene based materials for energy storage applications, Mater. Today Sustainability, 2022, 19, 100163 CrossRef.
- N. Shahin, S. Kazemi and A. Heidarpour, Mechanochemical synthesis mechanism of Ti3AlC2 MAX phase from elemental powders of Ti, Al and C, Adv. Powder Technol., 2016, 27, 1775–1780 CrossRef CAS.
- M. Mozafari and M. Soroush, Surface functionalization of MXenes, Mater. Adv., 2021, 2, 7277–7307 RSC.
- R. Akhter and S. S. Maktedar, MXenes: a comprehensive review of synthesis, properties, and progress in supercapacitor applications, J. Materiomics, 2023, 9, 1196–1241 CrossRef.
- D. Ayodhya, A review of recent progress in 2D MXenes: synthesis, properties, and applications, Diamond Relat. Mater., 2023, 132, 109634 CrossRef CAS.
- S. K. Balu, S. Andra, S. Chitra, R. Ramadoss, Y. R. Kumar, M. Selvaraj, H. A. Ghramh, M. A. Assiri and S. Mohan, Two dimensional (2D) MXenes as an emerging class of materials for antimicrobial applications: properties and mechanisms, J. Environ. Chem. Eng., 2022, 10(6), 108663 CrossRef CAS.
- B. Fu, J. Sun, C. Wang, C. Shang, L. Xu, J. Li and H. Zhang, MXenes: Synthesis, Optical Properties, and Applications in Ultrafast Photonics, Small, 2021, 17, 2006054 CrossRef CAS PubMed.
- R. M. Ronchi, J. T. Arantes and S. F. Santos, Synthesis, structure, properties and applications of MXenes: current statusand perspectives, Ceram. Int., 2019, 45, 18167–18188 CrossRef CAS.
- J. Wang, Y. Hu, B. Yang, X. Wang, J. Qin and M. Cao, Mechanochemistry-induced biaxial compressive strain engineering in MXenes for boosting lithium storage kinetics, Nano Energy, 2021, 87, 106053 CrossRef CAS.
- J. Jyoti, B. P. Singh, M. Sandhu and S. K. Tripathi, New insights on MXene and its advanced hybrid materials for lithium-ion batteries, Sustainable Energy Fuels, 2022, 6, 971–1013 RSC.
- S. Zhang, H. Liu, B. Cao, Q. Zhu, P. Zhang, X. Zhang, R. Chen, F. Wu and B. Xu, An MXene/CNTs@P nanohybrid with stable Ti–O–P bonds for enhanced lithium ion storage, J. Mater. Chem.
A, 2019, 7, 21766–21773 RSC.
- X. Wang, J. Chen, D. Wang and Z. Mao, Defect Engineering to Boost the Lithium-Ion Storage Performance of Ti3C2Tx MXene Induced by Plasma-Assisted Mechanochemistry, ACS Appl. Energy Mater., 2021, 4, 10280–10289 CrossRef CAS.
- N. Khandelwal and G. K. Darbha, A decade of exploring MXenes as aquatic cleaners: covering a broad range of contaminants, current challenges and future trends, Chemosphere, 2021, 279, 130587 CrossRef CAS PubMed.
- S. Iravani and R. S. Varma, MXene-Based Photocatalysts in Degradation of Organic and Pharmaceutical Pollutants, Molecules, 2022, 27, 6939 CrossRef CAS PubMed.
- S. Li, Z. Li, J. Li, J. Tan, Y. Zou, Y. Wang, Y. Lu, X. Zhu and T. Zhang, Tailoring Asymmetric Interface-Triggered Peroxymonosulfate Activation via Staircase-Shaped Edge-Rich-Defective Ti3C2Tx by Mechanochemical Treatment: Hydroxyl “Capping” or “Subtraction” Strategy?, ACS EST Eng., 2023, 3, 544–556 CrossRef CAS.
- T. Amrillah, C. A. Che Abdullah, A. Hermawan, F. N. Indah Sari and V. N. Alviani, Towards Greener and More Sustainable Synthesis of MXenes: A Review, Nanomaterials, 2022, 12, 4280 CrossRef CAS PubMed.
- X. Guan, Z. Yang, M. Zhou, L. Yang, R. Peymanfar, B. Aslibeiki and G. Ji, 2D MXene Nanomaterials: Synthesis, Mechanism, and Multifunctional Applications in Microwave Absorption, Small Stuct., 2022, 3, 2200102 CrossRef CAS.
- K. R. G. Lim, M. Shekhirev, B. C. Wyatt, B. Anasori, Y. Gogotsi and Z. W. Seh, Fundamentals of MXene synthesis, Nat. Synth., 2022, 1, 601–614 CrossRef.
- S.-Y. Pang, Y.-T. Wong, S. Yuan, Y. Liu, M.-K. Tsang, Z. Yang, H. Huang, W.-T. Wong and J. Hao, Universal Strategy for HF-Free Facile and Rapid Synthesis of Twodimensional MXenes as Multifunctional Energy Materials, J. Am. Chem. Soc., 2019, 141, 9610–9616 CrossRef CAS PubMed.
- Z. Zheng, C. Guo, E. Wang, Z. He, T. Liang, T. Yang and X. Hou, The oxidation and thermal stability of two-dimensional transition metal carbides and/or carbonitrides (MXenes) and the improvement based on their surface state, Inorg. Chem. Front., 2021, 8, 2164–2182 RSC.
- F. Cao, Y. Zhang, H. Wang, K. Khan, A. K. Tareen, W. Qian, H. Zhang and H. Ågren, Recent Advances in Oxidation Stable Chemistry of 2D MXenes, Adv. Mater., 2022, 34, 2107554 CrossRef CAS PubMed.
- R. A. Soomro, P. Zhang, B. Fan, Y. Wei and B. Xu, Progression in the Oxidation Stability of MXenes, Nano-Micro Lett., 2023, 15, 108 CrossRef CAS PubMed.
- B. Farasati Far, N. Rabiee and S. Iravani, Environmental implications of metal–organic frameworks and MXenes in biomedical applications: a perspective, RSC Adv., 2023, 13, 34562–34575 RSC.
- K. Maleski, V. N. Mochalin and Y. Gogotsi, Dispersions of Two-Dimensional Titanium Carbide MXene in Organic Solvents, Chem. Mater., 2017, 29, 1632–1640 CrossRef CAS.
- Y. Cui, J. Zhu, H. Tong and R. Zou, Advanced perspectives on MXene composite nanomaterials: types synthetic methods, thermal energy utilization and 3D-printed techniques, iScience, 2023, 26, 105824 CrossRef CAS PubMed.
- X. Liu, J. Yang, J. Wang, Y. Zhang, J. Wu and F. Yang, MXene-modified silicone rubber composites with high thermal stability: preparation and properties, Mater. Lett., 2021, 304, 130639 CrossRef CAS.
- X. Han, X. Qiu, M. Zong and J. Hao, Assembled MXene Macrostructures for Multifunctional Polymer Nanocomposites, Small Stuct., 2023, 4, 2300090 CrossRef CAS.
- A. M. Belenguer, G. I. Lampronti and J. K. M. Sanders, Reliable Mechanochemistry: Protocols for Reproducible Outcomes of Neat and Liquid Assisted Ball-mill Grinding Experiments, J. Vis. Exp., 2018, 131, 56824 Search PubMed.
- C. Len, V. Duhan, W. Ouyang, R. Nguyen and B. Lochab, Mechanochemistry and oleochemistry: a green combination for the production of high-value small chemicals, Front. Chem., 2023, 11, 1306182 CrossRef CAS PubMed.
- C. Espro and D. Rodríguez-Padrón, Re-thinking organic synthesis: mechanochemistry as a greener approach, Curr. Opin. Green Sustainable Chem., 2021, 30, 100478 CrossRef CAS.
|
This journal is © The Royal Society of Chemistry 2024 |
Click here to see how this site uses Cookies. View our privacy policy here.