DOI:
10.1039/D4MA00511B
(Review Article)
Mater. Adv., 2024,
5, 8384-8403
Phosphatidylserine: paving the way for a new era in cancer therapies
Received
18th May 2024
, Accepted 1st August 2024
First published on 30th August 2024
Abstract
Lipid phosphatidylserine (PS) plays a vital role in the growth and proliferation of cancer cells and has been identified as a potential target for cancer treatment. Recent studies have focused on using phosphatidylserine-targeting agents in the treatment of several classes of cancer, such as breast, lung, and prostate. The use of PS-targeting antibodies to target cancer cells while leaving healthy cells unharmed is selective. These antibodies are specifically targeted to phosphatidylserine molecules located on the exterior membrane of cancer cells, triggering a series of events that ultimately destroy the cancer cells. In addition, incorporating phosphatidylserine into the liposome membrane specifically targets cancer cells, thereby enabling more efficient drug delivery and improving cancer treatment outcomes. In general, PS has active ingredients that are currently undergoing clinical trials for potential use in treating various types of cancer. On the role of phosphatidylserine in biophysical and cancer biology, this review summarizes recent studies, as well as related prospective clinical and preclinical trials such as immunotherapy and biomarkers. A new indication of future PS implementation in cancer therapy appears to be a new era.
1. Introduction
Cancer is a multifaceted and complex disease, which has impacted numerous individuals globally. This chronic condition is marked by the uncontrolled proliferation of cells and the infiltration and dissemination of cells from their original sites to other locations within the body.1–3 According to Cancer Research UK, there exists a multitude of over 200 diverse forms of cancer that have the potential to occur in any anatomical region and can exert influence on individuals across various age groups, genders, and ethnic backgrounds.4 This phenomenon has instigated a significant shift in the conventional methodology of medical treatment, transitioning away from a generalized approach and towards a more personalized or “customized therapy” wherein the determination of treatment options is predicated upon the specific mutational configuration of a patient's tumor.5 Tumors can be classified as benign or malignant.6 Benign neoplasms are non-malignant tumors that cannot spread throughout the body. However, some neoplasms can still be dangerous based on their location, such as an intracranial neoplasm, which may present challenges in surgical removal.7 Whereas, malignant tumors do not remain encapsulated, show features of invasion, and metastasize,5,8 which are often more challenging to treat and can be life-threatening.9
Evidence suggests that cancer is a disease of the genome at the cellular level.1–3 Interestingly, most agents responsible for the onset of cancer, acknowledged as carcinogens, induce modifications in the sequence of DNA or mutations, commonly referred to as mutagens. Consequently, akin to all genetic disorders, the development of cancer is a consequence of modifications occurring within the DNA.10 Tobacco use, exposure to radiation or chemicals, virus infections, and specific dietary and lifestyle variables can all result in these alterations, which can either be inherited or acquired.11,12
Despite notable advancements in cancer research and treatment, there is still a great need for new and effective therapies that can improve patient outcomes while reducing toxic side effects. In particular, the lipid composition of the plasma membrane has been associated with the advancement and progression of cancer.12 One promising avenue of research involves targeting PS, a molecule pivotal for proliferation and survival. PS and phosphatidylcholine (PC) are two significant phospholipids that contribute to cellular membrane structural and functional properties.3 PS is a negatively charged phospholipid naturally present on the inner layer of the cell membrane, but during apoptosis or cell activation, the asymmetric arrangement of the phospholipids of the cell membrane is disrupted. With this novelty, PS is flipped to the outer leaflet of the membrane, where it serves as an “eat-me” signal for phagocytic cells.6 Additionally, PC is abundant in the outer leaflet of the cell membrane and contributes to membrane stability and fluidity. PS, which is available in outer plasma membranes and is subjected to a variety of stimuli, also involves the progression of various diseases,13,14 which may be found in other membranes during cell formation.
Basically, PS is dysregulated in different cancer types, including breast, lung, and colon cancer.15 Nowadays, PS is a promising target to focus on for cancer therapy due to its involvement in cancer progression and its potential as a focal point for therapeutic intervention. Understanding the role of PS in cancer cell survival and proliferation could lead to the development of innovative treatment approaches that specifically disrupt cancer cell function while sparing normal cells.16 Recent studies suggest that the inhibition of PS synthesis or the disruption of its interaction with proteins involved in cancer cell function presents an exciting avenue for novel cancer therapy.17 In addition, PS not only contributes to cancer cell survival and proliferation but also regulates tumor progression and metastasis.18,19 This dysregulation of PS has been associated with the ability of cancer cells to evade the immune system and promote angiogenesis, the neo-genesis of blood vessels that is crucial for tumor growth and dissemination.20–22
Furthermore, the relationship between PS and drug resistance in cancer has garnered significant attention. Cancer cells with elevated levels of PS have been found to exhibit increased resistance to chemotherapy and targeted therapies, posing a novelty and a considerable challenge in the clinical management of cancer.23 In addition to targeting PS directly, there is growing interest in utilizing PS as a cancer biomarker for prognosis and diagnosis.24,25 Advancements in imaging technologies and molecular profiling techniques have enabled the detection of elevated PS levels in cancerous tissues, providing valuable insights into disease progression and the potential response to treatment.15,26 Herein, we discuss several strategies that have been explored, including the use of antibodies, small molecules, and nanoparticles. By diminishing the levels of PS within cancer cells, these molecules can hinder their proliferation and viability. Encouraging outcomes have been observed in clinical trials by investigating PS-targeted therapies.26 Furthermore, this review addresses the challenges and future perspectives of PS-targeted therapies. Despite its promising potential, several hurdles remain, including the need for precise delivery systems and a better understanding of the role of PS in different cancer types. By elucidating these aspects, we hope to pave the way for the successful integration of PS-targeted strategies into the broader framework of cancer treatment. As research continues to unravel the complexities of PS and its interactions, it holds the promise of transforming cancer treatment paradigms, making it an essential focus for future oncological research.
2. Structural organization of PS
In a cell, PS and PC are the crucial phospholipids that are essential components of cell membranes. These phospholipids are asymmetrically distributed on the cell membrane, with PS predominantly located on the inner surface of the cell membrane and PC predominantly situated on the outer side.27 Cell-to-cell contact, membrane trafficking, signal transduction, and other cellular activities are all significantly impacted by the structural arrangement of PS and PC on the cell membrane.28 Understanding the organization and function of PS on the cell membrane is essential for developing targeted therapies for various diseases, including cancer.29,30
2.1. Molecular structure of PS with PC
PS is a negatively charged phospholipid composed of a serine molecule, a glycerol backbone, and two fatty acid chains. The serine molecule is attached to the glycerol backbone by a phosphate group and the fatty acid chains that form the hydrophobic tail.31 The hydrophilic head of PS contains a phosphate group, a serine amino acid, and a carboxyl group, which contribute to its negative charge.32–36
The plasma membrane of mammals is composed of nearly 1000s of phospholipid molecules, out of which PS comprises approximately 2–10% of total phospholipids.37 PS is a crucial phospholipid made up of two fatty acids attached by an ester linkage to the first and second carbons of the glycerol and serine linked by a phosphodiester linkage to the third carbon. It is a principal cell membrane component that is specifically present in the inner cytoplasmic leaflet of the membrane.38 PC and phosphatidylethanolamine (PE) are mostly converted enzymatically by mammalian cells via a serine exchange process to produce PS by the action of 2 enzymes, i.e. S-synthase 1 (substrate PC) and PS-synthase 2 (substrate PE), generated from the endoplasmic reticulum.39 PS is essential for apoptosis and cell signalling.40 It is generally accepted that one of the primary mechanisms for eliminating apoptotic cells is PS exposure to the outside of the cell membrane, which sends a signal called “eat me signal” and marks the cell for destruction.41 The chemical structure of PS confers upon it a crucial function as an essential constituent of cell membranes. It comprises a glycerol backbone, fatty acid chains, phosphate group, and amino acid serine. The glycerol backbone is a three-carbon structure that provides a rigid framework for the careful arrangement of the other PS constituents, and 2 fatty acid chains are present in PS that provide fluidity and stability to the cell membrane.42 The glycerol backbone of the PS molecule is attached to a phosphate group, which is further linked to a serine amino acid, hence giving the molecule the name “phosphatidylserine.” This linkage delivers hydrophobic properties to the molecule, as shown in Fig. 1.42
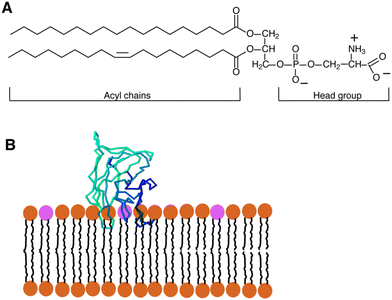 |
| Fig. 1 (A) Diagram showing the structure of a typical glycerophospholipid, or PS, which has both unsaturated and saturated fatty acyl chains. The head group has one net negative charge at a physiological pH. The interaction between PS and the C2 domain of lactadherin (LactC2; blue-green-barrel structure) in a membrane bilayer is depicted in (B). The PS head groups are displayed in fuchsia, and the remaining lipids are brown. Three “fingers” of the LactC2 structure are observed, which are believed to reach the hydrophobic area of the membrane bilayer and contain hydrophobic amino acids. Reprinted from, ref. 41 Copyright CC BY 2011 MDPI. | |
However, PC is a neutral phospholipid composed of a choline molecule, a glycerol backbone, and two fatty acid chains.5 Fatty acid chains produce the hydrophobic tail of the choline molecule, which is joined to the glycerol backbone by a phosphate group. The hydrophilic head of PC contains a phosphate group, a choline molecule, and a glycerol group.32,43 All the phospholipids are present in eukaryotic cell membranes, in which PC is the most prevalent, making up 40–50% of the total.37 It is one of the main components of lung surfactants and cell membranes and is abundantly present on the outer surface of the cell membrane. It is believed that the PC transfer protein (PCTP), which belongs to the steroidogenic acute regulatory protein-related transfer (START) superfamily, moves between membranes inside the cell and plays a crucial role in cell signalling.44 A range of fatty acids make up the glycerophosphoric acid and choline head groups that make up this phospholipid. A significant amount of dipalmitoylphosphatidylcholine can be found in animal lung PC.45
Two methods allow mammalian species to generate PC, out of which the CDP-choline pathway is the most important. This pathway is also known as the ‘Kennedy pathway’, as described by Eugene and Kennedy.46 Three enzymatic steps comprise the CDP pathway, which requires choline. First, choline kinase phosphorylates the choline into PC at the expense of ATP. Second, PC using CTP in the presence of cytidylyltransferase (CT) is converted into CDP-choline. Third, the conversion of CMP to diacylglycerol to create PC is catalyzed by 1,2-diacylglycerol choline phosphotransferase (Fig. 2). Through three consecutive methylations of PE by phosphatidylethanolamine N-methyltransferase (PEMT), PC can also be produced endogenously in a second pathway.47 The relevance of the endoplasmic reticulum (ER) in PC production was highlighted by subcellular fractionation, which showed that PEMT and the last enzyme in the CDP-choline pathway are both found there.48,49
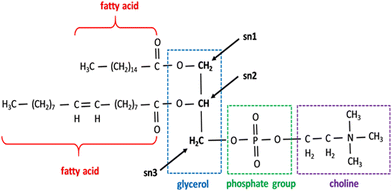 |
| Fig. 2 Phospholipids showing the structure of the PC. All phospholipids share the same fundamental structure but different fatty acids and head groups in place of choline. Sn 1, 2, and 3 positions are marked. Reprinted from, ref. 41 Copyright CC BY 2019 MDPI. | |
2.1.1. Distribution of PS and PC in the membrane.
PS and PC are both found in the membrane of all cells, but their distributions are different in different cell types and the specific membrane domain. In general, PS is more abundant in the inner side of the cell membrane, where it constitutes up to 10–20% of the total phospholipids.50 This asymmetric distribution is maintained by a class of enzymes called flippases,51 which selectively transport PS from the outer to the inner leaflet. In healthy cells, exposure to PS on the outer leaflet is minimal.52 In apoptotic cells, PS is translocated to the outer surface, where it serves as a signal for phagocytosis by macrophages and other immune cells.53 Moreover, PC is more abundant on the outer side of the cell membrane, constituting up to 50–60% of the total phospholipids.54 This distribution is also maintained by flippases, which selectively transport PC from the inner to the outer surface. Furthermore, PC is present in lipid rafts,55 which are specialized membrane regions essential for cell signalling and signal transduction and rich in signalling molecules and membrane receptors.56
In mammalian cells, PS is produced inside a particular area known as mitochondria-associated-membrane (MAM),57 which is present in the ER and is synthesized from PC or PE by phosphatidylserine synthase-1 (PSS-1) or phosphatidylserine synthase-2 (PSS-2) through a base-exchange process with serine, as shown in Fig. 3. After blending due to the interaction of MAM and mitochondrial exterior layers, a part of the PS moves into the mitochondria.24,58 The PS present inside the mitochondria releases carbon dioxide. PE is regulated by the PS decarboxylase (PSD) found in the prokaryotes and mitochondria of eukaryotes (Fig. 3). Further, it was found that insufficiency of PSD quality resulted in underdevelopment and severe health issues in mice.24,58 Thus, for proper cell growth and mitochondrial functionality, the process of PE production through PS becomes crucial. The remaining PS is sent to various organelles, such as plasma film and Golgi, as shown in Fig. 3. Most transportation occurs through non-spontaneous processes, such as solvent vehicle proteins or vesicles.58 However, the number of integrated PS molecules that enter mitochondria vs. other organelles is still a mystery.
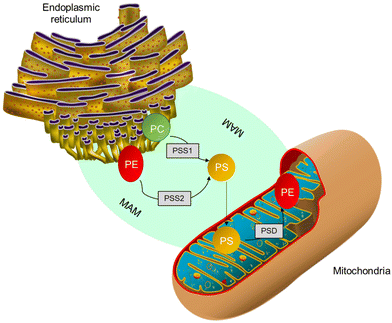 |
| Fig. 3 PS production inside MAM from PC either by PSS1 or PSS2. Further, a few PSs are transmitted to mitochondria and decarboxylated to form phosphatidylethanolamine by PS decarboxylase.58 | |
2.1.2. Interactions of PS and PC with other lipids and proteins.
The presence of PS and PC in the membrane has important implications for their interactions with other lipids and proteins. PS has a high affinity for calcium ions, and its binding to calcium is known to regulate various cellular processes, such as blood clotting, synaptic transmission, and apoptosis.59 In addition, PS can interact with other lipids, such as cholesterol and sphingolipids,51 to form specialized membrane domains known as lipid rafts. These domains are enriched in signalling molecules and membrane receptors and are essential for signal transduction and cell signalling.
PC also interacts with various lipids and proteins, including sphingomyelin,60 which is another abundant phospholipid in the membrane. The interaction between PC and sphingomyelin is known to form a specialized type of lipid raft, known as the liquid-ordered phase or the raft phase, which has distinct biophysical properties compared to the surrounding membrane.61 These domains are essential for the organization of signalling molecules and membrane receptors, as well as for the regulation of membrane fluidity and permeability.
In addition to their interactions with lipids, PS and PC also interact with proteins in the membrane. For example, PS has been shown to interact with several proteins involved in blood clotting, including coagulation factor Va, prothrombin, and protein C.62 These interactions are essential for the regulation of blood clotting and thrombosis. PC, however, interacts with several membrane proteins, such as ion channels, transporters, and receptors. These interactions are essential for the regulation of cell signalling, ion homeostasis, and nutrient uptake.
2.2. Functional roles of PS and PC in cells
The distribution and interactions of PS and PC in the membrane play critical functional roles in the cell. For example, the asymmetric distribution of PS in the cell membrane is vital for the recognition and clearance of apoptotic cells by phagocytic cells.63 The exposure of PS on the outer surface of the membrane serves as a signal for recognition and phagocytosis, which is an essential process for the removal of damaged or infected cells.5
The distribution of PC in the outer surface of the cell membrane is vital for the formation and stability of lipid rafts, which are critical for cell signalling and membrane organization. The interaction between PC and sphingomyelin in the lipid raft phase is vital for the organization of signalling molecules and membrane receptors, which is critical for signal transduction and cell signalling.64 In addition, the fluidity and permeability of the membrane are regulated by the distribution and interactions of PS and PC with other lipids and proteins, which are essential for the regulation of cell function and adaptation to changing environmental conditions.50
In many biological processes, PS or PC is essential for function. It is a component of the cell membrane and plays a role in endocytic internalization, intracellular vesicle movement, and the establishment of cell polarity. Furthermore, PS plays a vital role in preserving the structural and functional integrity of cell membranes.65 Additionally, PS exposure is connected to phospholipid externalization during biological processes, such as apoptosis.33,66 In terms of brain function, PS and DHA are essential for the structure and operation of the brain.67 Furthermore, the synthesis of PS by PS synthase is pivotal for the growth and maintenance of neurons.68 PS plays a function in neurotoxicity and Alzheimer's disease because it is widely distributed in neuronal membranes and is exposed on the cell surface when oxidative damage occurs69
Moreover, PS modifies the secondary structure of protein aggregates and impacts the rates at which proteins form, especially when amyloidogenic proteins are present. Furthermore, PS plays a role in insulin production, insulin signaling transduction, and the development of problems related to diabetes. For instance, PS supplementation has demonstrated promise in preventing unfavorable ventricular remodeling and decreasing the size of myocardial infarcts,70 indicating a possible role in cardiac health. In tumor microenvironments, PS has also been linked to the control of T cell responses, suggesting that PS may be a valuable target for cancer immunotherapy. Knowing the functioning properties of PS is critical to comprehending different disorders and creating effective treatment plans.22,33,71,72 PS is crucial for more than just cell activities; it may also have therapeutic uses.
Regardless of the evident potential of PS-targeted therapies, notable challenges and constraints exist that necessitate attention. For instance, certain tumors may exhibit low expression levels of PS, rendering them less responsive to these treatments, as shown in Table 2.15 Furthermore, these therapies may exert off-target effects that have the potential to induce undesired adverse reactions. Nevertheless, there is a basis for optimism regarding the future of PS-targeted therapies in the field of cancer treatment. An intriguing prospect involves the combination of PS-targeted therapies with immunotherapy.15 PS and immune cell exposure in tumor microenvironments yield immunosuppression and the advancement of tumor development. Therefore, for the survival of cell proliferation, growth and symptoms linked to cancer, the PS position on the membrane is vital.38,73
Understanding the intricate interaction of PS within the field of cancer biology and its repercussions for resistance to treatment is of utmost importance for the advancement of more efficient therapeutic approaches. Through the specific targeting of PS and the pathways associated with it, innovative treatment methods have the potential to enhance the effectiveness of current therapies and surmount drug resistance in cancer.
3. PS in cancer treatment
3.1. Exposure of PS to immune suppression
Immune suppression and inflammation provide favourable conditions for tumor development by impairing the immune system's capacity to identify and eliminate cancer cells.74–76 Tumor cells can escape immune system detection by interfering with the stage of T-cell activation.77 In the tumor microenvironment, PS is abnormally exposed and is essential for producing immunosuppressive signals that obstruct the development of both systemic and local antitumor immune responses.29,76,78 PS is exposed to the outer membrane within the tumor microenvironment due to various metabolic processes linked to apoptosis. Activation of caspases, production of reactive oxygen species, and cell activation-induced calcium ion inflow are some of these processes.79 PS exposure significantly impairs both innate and adaptive immune responses, enabling tumor cells to avoid immune detection.80 Interestingly, PS exposure is known to be a point of control that may be addressed pharmacologically and functions as an early indicator or precursor of the several immunosuppressive signals that follow.81 The primary causes of PS exposure in the tumor microenvironment are necrotic tissue and apoptotic cells introduced by pathological states or treatment. Nevertheless, it has also been seen in living endothelial cells and extracellular vesicles derived from leukocytes, stroma, and tumors. Tyro, Axl, and Mertk (TAM receptor tyrosine kinases) are among the several PS receptors that are particularly crucial for the detection of PS and the induction of immune suppression in the tumor microenvironment.82 T cell/transmembrane, immunoglobulin, and mucin (TIM) are additional PS receptors.83 Tim-1, Tim-3, and Tim-4 are associated with receptor-mediated immunosuppression of Th1 cells, activation of Th2 cells—a subset of T helper cells important in humoral immunity—and the uptake of apoptotic cells by macrophages and dendritic cells. The receptor tyrosine kinases (RTKs) that make up the TAM gene family are expressed on leukocytes and different kinds of tumors. Protein S or Gas6 are bridge proteins that have γ-carboxylated domains that allow TAM RTKs to connect to PS. As the TAM receptor is engaged in interaction with the receptor-binding domain, the exposed PS is directly bound by the γ-carboxylated GLA domain of Gas6 and Protein S. Therefore, when TAMRTKs on macrophages are activated, target cells exposed to PS are phagocytosed, resulting in macrophage polarization, which acts as a pro-inflammatory signal, changing the phenotype of the macrophages from “M1” to “M2,” which has pro-tumor activity, and allowing for the production of interleukin-10 and TGF-β.84 TAM RTK activation on tumor cells is associated with resistance to chemotherapy and the ability of tumor cells to undergo epithelial plasticity. Preclinical studies have shown decreased tumor growth and metastasis when the vitamin K-dependent γ-carboxylation of Gas6 is inhibited, preventing Axl from being activated on tumor cells.84 Additionally, PS-TAM RTK binding to tumor cells escalates the expression of programmed death-ligand 1 (PD-L1). Therefore, blocking the activation of the TIM and TAM-RTK pathways by PS can enhance immune responses against cancer, as shown in Fig. 4.85 Furthermore, a study revealed that radiation therapy elevates PS expressions in B16 melanoma in mice bodies. The extrinsic plasma membrane surface of tumor cells, PS, hinders the immunological response to receptors found in T cells and others.85,86 Inhibition of dendritic cell antigen representation and induction of regulatory T-cell limits T-cell activation by producing immunosuppressive cytokines, such as IL 10 or TGF-β.
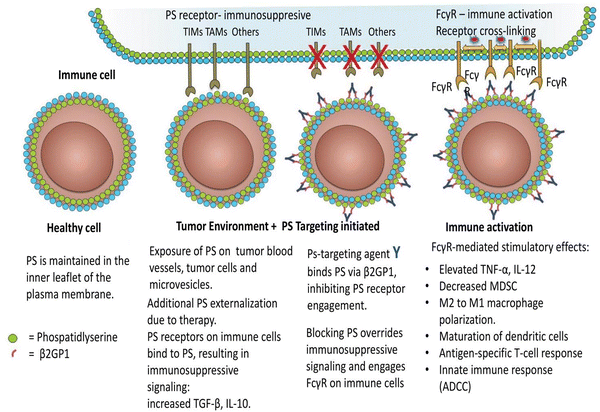 |
| Fig. 4 Immune cells are activated in the tumor microenvironment by PS-targeting antibodies interacting with exposed PS. As the article explains, oxidative stress and an immature tumor vasculature work together to cause PS to show marked dysregulation in the tumor microenvironment. This imbalance is further exacerbated by the release of PS-positive tumor exosomes and the increased apoptotic index of tumours that are actively growing. Antibodies specifically targeting PS are thought to bind to externally exposed PS molecules with selectivity and interfere with PS's ability to regulate tumor microenvironmental functions by preventing PS from connecting to PS receptors and by blocking Fcγ-mediated ADCC (antibody-dependent cell-mediated cytotoxicity). Consequently, immunogenic signals are triggered in the tumor microenvironment. Reprinted from, ref. 85 Copyright CC BY 2018 Taylor and Francis Online. | |
Moreover, the binding of PS to T cell receptors blocks T cells that are activated by GPR174 (G-protein coupled receptor 174 is a protein encoded by the GPR174 gene in humans). This G-protein coupling receptor shortage of T-reg cells can induce macrophage polarization and upsurge IL-10 expression.24 When PS encounters macro-vesicles by PS, phagocytes clear apoptotic cells, preventing undesired inflammatory reactions and equilibrating an anti-inflammatory state in the tumor microenvironment, as shown in Fig. 5. Exposure of PS from tumor samples in patients has been demonstrated to suppress the activation of T-cell reactions24 in micro-vesicles (exosomes). However, PS exposure in tumor cells also promotes anti-tumor effects through the mediation of long-term inflammation.
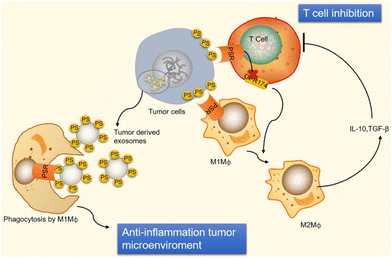 |
| Fig. 5 Immunological suppression occurs in tumour cells and vesicles because of PS exposure. Immunological suppression occurs when PS is given to cancer cells because it ligates to receptors on T cells and macrophages. When PS binds to PSR on macrophages, M2-like macrophages develop and start to release TGF- and IL-10, two anti-inflammatory cytokines. TGF- and IL-10 are cytokines that inhibit the immune system and stop T cells from being activated. Reprinted from, ref. 24 Copyright 2020 Theragnostic. | |
As per a recent study, it has been observed that PS exposure in the body, under conditions with a large number of cells, is essential to bind IFNγ and IL-12 for the conversion of transient cytokine stimuli towards a long-lasting inflammation as a result of reducing immunosuppressive functions.87 In conclusion, the immune response of PS and its immunosuppressive consequences is an intricate process in the tumor microenvironment. This mechanism seems to be a conserved evolutionary strategy among higher metazoans to safeguard against autoimmune complications that may arise during the regular disposal of dying host cells.87 Although the development of agents that target PS receptors is progressing through various stages of pre-clinical and clinical investigation, advanced-stage clinical trials are currently underway to evaluate the efficacy of the PS-targeting antibody bavituximab in multiple oncology indications.
3.2. PS in biomarkers for imaging tool
The discovery and characterization of biomarkers are essential to the advancement of cancer research because they enhance the effectiveness of therapies, diagnosis, and screening. PS has demonstrated potential as a biomarker for the identification and imaging of several forms of cancer. Studies have shown that PS is present on the interface of cancer cells. This process is controlled by calcium-dependent flippases and scramblases, which are enzymes that catalyze the transfer of lipid molecules. The former moves lipids towards the cytoplasm, while the latter moves phospholipids ATP-independently between the inner and outer leaflets. For this reason, PS labelling techniques are useful for enabling tumor visualization.27 Cancer cells exhibiting a lower presence of PS on their surface seem to display a heightened susceptibility to both irradiation and chemotherapeutic agents, such as gemcitabine (Gemzar)/nab-paclitaxel.88 However, cancer cells with higher PS levels on their surfaces are more susceptible to PS-targeting anticancer therapies, such as saposin C encapsulated in a dioleoyl PS nanovesicle (SapC-DOPS).89 Using different strategies, it has been demonstrated that cancer cells targeting PS have been imaged.90,91 In a previous study, researchers used a compound called Annexin V-Cy to image gliosarcoma tumors in mice. When pro-apoptotic drugs were administered to the tumors, they observed that the fluorescent signal in the tumors increased three times. Zhao et al. used PGN635, a new monoclonal antibody that binds exclusively to PS, to perform in vivo optical imaging of exposed PS. The F(ab′) (2) fragment of PGN635 was linked to the near-infrared (NIR) dye IRDye800CW. After injecting 800CW-PGN635 into mice with subcutaneous or orthotopic U87 glioma xenografts, either treated with or without radiation, in vivo dynamic NIR imaging was performed. Following the injection of 800CW-PGN635 into non-irradiated subcutaneous U87 gliomas, the resulting NIR optical imaging showed a clear contrast in the tumors.92 Another imaging technique called positron emission tomography (PET) is employed for radioactive tracers to visualize PS. In another investigation, researchers utilized PGN635 – 89Zr (Zirconium-89) to visualize tumors in both mice and humans. They discovered that the tumors exhibited elevated levels of radioactive tracers, thereby enabling their visualization through PET. Another method for visualizing PS is to use radio waves and magnetic fields, called magnetic resonance imaging (MRI). In a subsequent study, PGN635, along with superparamagnetic iron oxide nanoparticles (SPIO), was used to visualize tumors (breast cancer) in mice, wherein the researchers noticed that the tumors displayed a reduction in signal intensity on MRI, thereby facilitating their detection. A nano-probe known as PGN-IOL/DiR, which is made up of liposomal that binds to PS and enters cells, has a good effect on mice breast cancers of MDA-MB 231.88 Similarly, the use of green indocyanine bonded to PS antibodies in three negative breast cancer cells enabled the tracking and mapping of apoptosis89 and facilitated an effective therapy strategy. PS species may also be biomarkers for the diagnosis of malignancy. The 36 most frequent lipid types were evaluated in one clinical investigation of 15 patients with prostatic cancer and 13 healthy controls. The results demonstrated that a specific species, “PS (18
:
1/18
:
1)”, appeared to have significant implications for normal patients and those with prostate cancer; the two categories, % of sensitivity, differed in the combinations PS (18
:
1/18; 1), lactosylceramide (18
:
1/16
:
0) and PS (18
:
1/18
:
2).90 A new study in patients with lung cancer evaluated lipids in aerosols between cancer and normal cells. In cancerous aerosols, an overexpose of PS was detected with declining PCs,91 which showed that PS is a viable biomarker for lung cancer in combination with other indices. PS species can also be related to the growth and progression of cancer. A method that combines PS and SapC, an endogenous sphingolipid activator protein, can be used to track several cancer cell lines. At an acidic pH, SapC has a considerable binding affinity for PS and is essential for the activation of lysosomal enzymes and the production of sphingosine and ceramide from the breakdown of sphingolipids. Because of the release of lactate from anaerobic glycolysis, tumors are known to have high levels of PS on their cell surface and to have a lower extracellular pH in comparison to normal tissues.91 Consequently, the combination of SapC and PS provides a beneficial and selective technique for targeted tumor imaging and treatment. An illustration of the various SapC-imaging modalities is presented in Fig. 6. The PS-targeting SapC-DOPS nanocarrier technology has been effectively applied to single-photon emission computed tomography (SPECT), MRI, and optical cancer cell imaging.93 Chu et al.94 used CellVue Maroon (CVM)-tagged SapC-DOPS nanovesicles, which contain the far-red fluorophore CVM, to show how to identify brain tumors and arthritic joints in mice. MRI containing iron oxide particles enclosed in SapC-DOPS nanocarriers was utilized to image neuroblastoma selectively. Winter et al. used paramagnetic gadolinium chelates, namely gadolinium-DTPA-bis(stearylamide) (Gd-DTPA-BSA)-loaded SapC-DOPS vesicles, as a targeted contrast agent for glioblastoma multiforme tumor imaging.95Fig. 7 presents the inferred findings from the Winter et al. experiment, which shows that the T1 of tumor cells maps before and after a 10-hour injection of Gd–DTPA–BSA/SapC-DOPS vehicles. In summary, the distinctive characteristics of polystyrene offer significant benefits in the field of imaging applications, as PS-targeting techniques allow for the specific visualization of tumors. Undoubtedly, these techniques will have a significant impact on upcoming diagnostic applications.
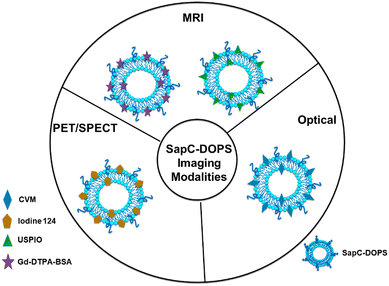 |
| Fig. 6 Schematic diagram showing that many imaging modalities use saposin C-dioleoyl phosphatidylserine (SapC-DOPS)-based modalities to visualize tumors. The far-red fluorophore CVM can be attached to the SapC-DOPS nanovesicles to provide optical imaging for both in vivo and in vitro studies. The use of gadolinium chelates, such as ultrasmall superparamagnetic iron oxide (USPIO) or gadolinium-DTPA-bis (stearyl amide) (Gd-DTAPBSA), as MRI contrast agents is necessary to enable in vivo MRI. Moreover, SapC-DOPS combined with an iodine-124 contrast agent is used for in vivo PET/SPECT imaging. Reprinted from, ref. 18 Copyright CC BY 2022 MDPI. | |
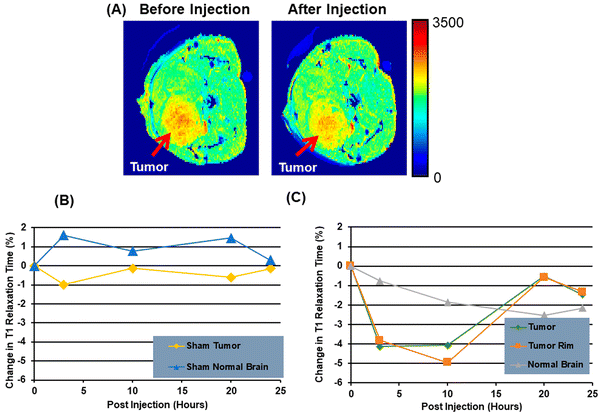 |
| Fig. 7 A mouse model of brain cancer is used to investigate the use of saposin C-dioleoyl phosphatidylserine (SapC-DOPS) as a carrier for contrast agents in MRI. First, a mouse with a glioma undergoes a high-resolution MRI (A). Finally, after injecting Gd-DTPA-BSA/SapC-DOPS vesicles into the tumor rim cells, and normal brain, the percentage change in T1 is evaluated (B) and (C). Reprinted from, ref. 18 Copyright CC BY 2022 MDPI. | |
With the finding of anxA5, a PS-binding protein96 results in the invention of the anxA5 affinity test for evaluating apoptosis by focusing on PS that is exposed to the surface.97,98 With the exception of the myoblast and the megakaryoblast, anxA5 interacts with dying cells rather than surviving ones throughout the body's diverse environment according to the study. In animal vitro models, some researchers have expanded the range of in vivo applications of the anxA5 affinity method.99–101 Because AnxA5 is associated with apoptosis, macrophage infiltration, and the release of red blood cells through intraplate bleeding, it has recently been found to be a helpful marker for differentiating between stable and unstable atherosclerotic plaques in patients.102 Recent studies have suggested that a PS-binding antibody can be used for the molecular imaging of vascular endothelial cells in solid tumors.103
3.3. PS in liposomal carriers
PS is a crucial anti-tumor mechanism in several liposomal carriers. These liposomal transporters stick with PS in the tumor microenvironment and release anti-tumor sequels through metabolism or synergistic drug effects. SapC-DOPS interacts primarily with cancer cells that contain PS and causes apoptosis by inducing ceramide accumulation and caspase activation. Research organizations have used SapC-DOPS to treat several cancers, such as brain cell tumors104 and cancer cell lines from the skin,105 as well as cancer cells from the pancreas.106 SapC-DOPS was found to be beneficial for various metastatic tumors according to the findings. The same research discovered that cells with high top PS levels have more survival probability under radiation therapy and that this was inversely related to themselves. In response to SapC-DOPS therapies, this may have been employed in combination treatment with significant exposure to tumor cells during radiation therapies, as shown in Fig. 8.107
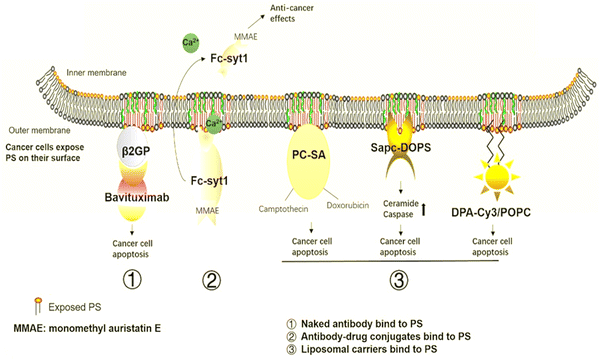 |
| Fig. 8 Cancer therapies involving PS. The medications aim to target PS in cancer treatment. (1) Naked antibodies bind to PS. (2) Antibody–drug conjugates bind to the PS. (3) Liposomal carriers adhere to PS. Reprinted from, ref. 24 Copyright CC BY 2020 Theragnostic. | |
Furthermore, in cancer cells and tumors, PC-SA is a liposomal carrier with a cationic property. PC-SA was found to be more effective when combined with typical tumor treatments in a preclinical study, and it also had anti-cancer benefits when used alone. The cancer medicines, which were camptothecin with doxorubicin encapsulated in PC-SA type liposomes, were used in this study to decrease tumor growth as PC-SA increased the half-life of anti-tumour drugs to carry a long-range in cancer treatment.107
3.4. PS in targeted drug delivery
PS is expressed on the surface of dead and alive cancer cells,108,109 as well as on the endothelial cells of blood vessels.110 This has led to recent speculations that PS is a viable drug-supply target. PS as a medication delivery target is further supported by clinical experience with molecular oncological imaging of PS (TDD). PS selection using 3G4 monoclonal anticorps has recently been shown to improve cytostatic treatment efficacy in mouse cancer models.111,112 Surprisingly, 3G4 was designed to identify the plasma PS-binding protein beta2-glycoprotein 1 rather than PS. This demonstrates that when PS is delivered to the membrane in vivo, it is immediately opsonized by various endogenous PS-binding proteins, such as beta2 glycoprotein 1. In addition to AnxA5, it belongs to the same PS-binding protein family. AnxA5 competes effectively with other PS-binding proteins for PS-bound locations in vivo in humans according to the molecular imaging experience with the protein. This has been confirmed in an in vitro system using isolated proteins and PS binding assays.113 Consequently, AnxA5 is a potential therapeutic option for treating PS in vivo.
3.5. PS in molecular imaging target
With the finding of anxA5, a PS-binding protein96 results in the invention of the anxA5 affinity test for evaluating apoptosis by focusing on PS exposed to the surface.97,98 Apart from the myoblast and the megakaryoblast, anxA5 interacts with dying cells rather than surviving ones throughout the body's diverse environment according to the study. In animal vitro models, some researchers have expanded the range of in vivo applications of the anxA5 affinity method.99–101 Because AnxA5 is associated with apoptosis, macrophage infiltration, and the release of red blood cells through intraplate bleeding, it has recently been found to be a helpful marker for differentiating between stable and unstable atherosclerotic plaques in patients.102 Recent research suggests that a PS-binding antibody can be used for molecular imaging of vascular endothelial cells in solid tumors, as shown in Table 1.103
Table 1 PS-targeting imaging strategies and therapeutics, along with their findings
Strategy/approach |
Description |
Findings and applications |
PS-targeting imaging strategies |
Annexin A5 (anxA5) affinity test |
Utilizes the high affinity of Annexin A5 for PS to measure apoptosis |
Effective in identifying apoptotic cells in various cancer types. Widely used in molecular imaging for cancer diagnosis |
Radiolabeled PS Probes |
PS-binding proteins tagged with radioactive isotopes |
Enables non-invasive imaging of tumor cells. Provides clear visualization of apoptotic cells in preclinical and clinical settings |
PS-targeting liposomes |
Liposomes designed to bind to PS-exposed cancer cells |
Enhances the delivery of imaging agents to tumor sites, improving the accuracy of cancer detection |
Optical imaging with PS-binding dyes |
Dyes that specifically bind to PS and emit fluorescence |
Allows real-time imaging of tumor progression and response to therapy. Useful in intraoperative imaging to guide surgical resection of tumors |
PS-targeting therapeutics |
PS-targeting antibodies |
Antibodies designed to bind specifically to PS-exposed cells |
Effective in delivering cytotoxic agents directly to tumor cells, reducing off-target effects. An example is bavituximab |
Liposomal drug delivery systems |
Liposomes encapsulating chemotherapeutic drugs that target PS |
Improves the pharmacokinetics and biodistribution of drugs, enhancing their therapeutic efficacy |
Antibody–drug conjugates (ADCs) |
Antibodies linked to cytotoxic drugs targeting PS-exposed cells |
Shows promising tumor-killing effects in preclinical models. Potential for reduced side effects compared to conventional chemotherapy |
Natural plant extracts |
Extracts from plants that exhibit anti-cancer properties targeting PS |
Demonstrates potential in inducing apoptosis in cancer cells. Offers a natural and potentially less toxic alternative to synthetic drugs |
PS-targeting nanoparticles |
Nanoparticles functionalized to bind PS on tumor cells |
Facilitates targeted drug delivery and reduces systemic toxicity. Shows potential in improving the efficacy of cancer treatments |
Table 2 PS is in clinical use playing an appropriate clinical significance role
Characteristics |
Clinical significance of PS |
Diseases |
Ref. |
Biomarker |
Increased PS expressions |
B16 melanoma |
114
|
Biomarker |
Exosomal phosphatidylserine (PS) 18 : 1/18 : 1 |
Prostate cancer |
90
|
Biomarker |
Presence of IgM anti-PS-prothrombin complex |
Cancer-associated vasculitis |
27
|
Biomarker |
Presence of IgA anti-PS-prothrombin complex |
Henoch-Schonlein purpura |
115
|
Imaging tool |
Liposomal PGN-IOL/DiR binds to PS |
Breast cancer |
88
|
Imaging tool |
Indocyanine green, tagged PS antibodies |
Triple-negative breast cancer |
89
|
Immunosuppression |
phosphatidylserine-micro vesicles induce TGF-β expression and suppression of macrophages |
Melanoma |
29
|
Tumoricidal target |
SapC-DOPS nano vesicles- phosphatidylserine-targeting agent |
Neuroblastoma |
30,104
|
Tumoricidal target |
SapC-DOPS Nano vesicles- phosphatidylserine-targeting agent |
Skin cancer |
105
|
Tumoricidal target |
SapC-DOPS Nano vesicles- phosphatidylserine-targeting agent |
Pancreatic cancer |
93
|
Antitumor effect |
3G4 monoclonal against PS |
Breast cancer |
111
|
Antitumor effect |
|
|
|
|
PS externalizations and DNA fragmentation by plant products (Chalepin) |
Breast cancer |
32,116
|
Antibody-based killing |
Rituximab mediated killing of CD 20 positive cells |
CD20-positive tumour cells |
43,117
|
3.6. PS in antibody–drug conjugates
Many conjugate antibody drugs are part of the development of targeting medicines that use PS. These drugs are associated with PS-targeting immunizer antibodies, which are cytotoxic medicines that produce anti-tumor effects, as shown in Fig. 9. Some immunizer antibody–drug conjugate medication forms have been proven in trials to have good tumor-suppressive activity to “bare” antibodies available on it, for example, a PS-focusing agent made using PS binding particles, which is a human Fc component that is C2A identified by IgG1. In the breast and prostate cancer models of mice, the noted usage of an Fc-Syt1 synthesized to monomethyl auristatin E, which acted as a cytotoxic chemical, had significant anticancer effects.118 According to some experts, the PS peptide is transferred to pH-sensitive micelles (PEGPDLLA) and then used as a chemotherapeutic drug paclitaxel (PTX) in those micelles. These pH-sensitive micelles are designed to address a corrosive set-off drug discharge framework that is appropriate for an acidic environment with a tumor. Moreover, vivo research concluded that the produced agents generated cytotoxicity and tumor cell uptake, as well as aggregation of tumors.119 In addition, fusion proteins containing L-methionase linked to human Annexin-V have been discovered. In comparison to L-methionine with no fusion protein present, the antibody PS has the most significant effect on tumor cell killing.120 Furthermore, the fusion protein does not affect normal cells, indicating that technology has the potential to develop novel drugs.
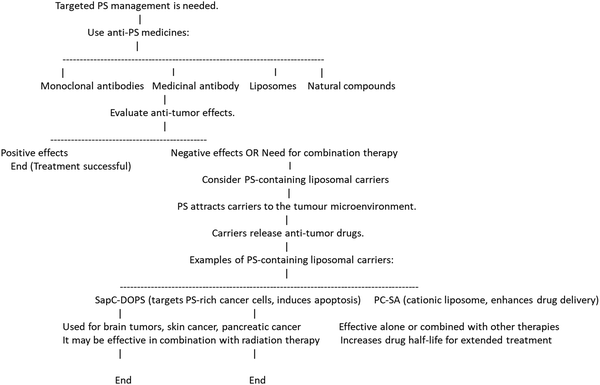 |
| Fig. 9 PS management in detail for end-to-end using manners. | |
3.7. PS with CD47 as a therapy for cancer
PS exposure to the phospholipid bilayer has the potential to induce phagocytic signs, which can be distinguished by immune cells, such as macrophages. In contrast, CD47 articulation on the cells can simultaneously repress the process of phagocytosis. The CD47 is a vital immune suppressive signal used by different tumor cells.121 The CD47 can act as a ligand for a category of glycoprotein known as signal regulatory protein-α or SIRPα. Upon binding of the CD47, the SIRP signaling cascade can be activated, which in turn inhibits phagocytosis81 and allows tumor cells to escape from surveillance of immune cells, such as macrophages and T cells.122 However, in contrast, CD47 ligation induces the articulation of PS in erythrocytes as part of a demise pathway, and CD47 has been suggested to impact PS exposure.123 Again, knocking down the CDC50A, which is a subunit of ATP11C involved in the flipping of PS, can cause the tumor-associated macrophages to improve the anti-CD47 bar's activity to limit tumor growth.124 As mentioned previously, the knockdown of CDC50A consequently increases the exposure of PS in the immortalized category T cells known as Jurkat cells. Hence, it is supposed to impact T cell function. Consequently, the CD47 barrier prevents phagocytosis; nonetheless, PS openness is used to target tumor cells associated with macrophage clearance work. When rituximab, an anti-CD20 antibody, is used with anti-CD47 medications, an anti-CD47 inhibitor, clinical trials have demonstrated some anti-cancer activities with negligible adverse effects in patients with aggressive and indolent lymphoma.117
4. Functions of PS in cancer
In the discipline of immunology, the goal of treatment is to pinpoint the signals that distinguish cancerous cells from non-cancerous ones: ‘eat me’ and ‘don't eat me’. The uneven distribution in eukaryotic cells results in the presence of unfavourable lipids, such as PS and PE, on the cytoplasmic side of the cell membrane and positive phospholipids, such as PC and sphingomyelin, on the outer side, which is sustainably mediated by the controlled activity of ATP-dependent and -independent enzymes referred to as scramblases, floppases, and flippases. In a tumor environment, due to various pathways, the PS is exposed to external surfaces, which results in an ‘eat me ‘signal, leading the cell towards apoptosis.43,117 Due to PS exposure's immunosuppressive qualities, tumor cells can better elude immune surveillance by weakening both innate and adaptive immune responses. The TAM gene families, as well as T cell/transmembrane, immunoglobulin, and mucin (TIM)44, are PS receptors in the tumour microenvironment.82
4.1. Apoptotic function of PS
Every day, millions of cells constantly die and regenerate in our bodies. The dead cells are then eliminated by the action of PS, which initiates a process called apoptosis that causes the dead cells to be phagocytosed.98,125 The apoptosis pathway takes place in 2 ways: the intrinsic pathway, which takes place inside the mitochondria, and the extrinsic pathway, which takes place via numerous apoptotic factors. The intrinsic pathway is initiated by the activation of cytochrome C, which further activates caspase 3/7 liberated from mitochondria. In contrast, in the extrinsic pathway, apoptotic factors, such as TNF and Fas ligand (FasL), activate caspase 3 and promote cell apoptosis. Exposure to the cell membrane PS serves as a clear signal for the recruitment of macrophages and the engulfment of cells, indicating the initiation of apoptosis. Studies on mice and humans have shown that another protein called Xk-related protein 8 (Xkr8), also known as scramblase, becomes incompetent to expose PS during apoptosis if hypermethylated because its expression is suppressed. Moreover, other proteins, such as basigin (BSG) and neuroplastin (NPTN), belonging to the Ig superfamily, and ATPase Phospholipid Transporting 11C(ATP11C), a significant flippase protein-producing gene, are necessary for flipping of PS to the outer leaflet of the membrane. Any mutation can lead to no apoptosis and is not taken up by macrophages. Thus, both flippase and scramblase activities are necessary for PS exposure and performing programmed cell death.23,100,126 Further, the non-apoptotic functions of PS include blood coagulation, myoblast fusion and T lymphocyte activation.
4.2. PS with TIM and TAM receptor function
Two receptor families mediate the phagocytic clearance of apoptotic cells, the TIM and TAM proteins, which are dependent on PS (PtdSer). Tim receptors are a type of membrane receptor that recognizes PS signals and exerts immune suppression.127–129 Three receptors are encoded by the TIM gene on the human chromosome, i.e. TIM1, TIM3 and TIM4, out of which TIM 3 is highly studied in the immune-cancer biology area. Tim 3 is expressed on dendritic cells, CD8+ T cells, antigen-presenting cells, and other immune cells, such as monocytes and natural killer cells. It promotes the phagocytosis of apoptotic bodies, preserves immunological tolerance, and triggers inflammatory reactions. It is well-established that inhibiting TIM-3 can stop tumor development.130 Furthermore, the mast cells, b cells and cd4+ t cells express the TIM-1 receptor, which also acts as a binding site for the Ebola virus. Dendritic cells and macrophages have significant levels of TIM-4 expression. Tim-4 is involved in the inflammatory reactions of the body.131,132
According to recent research, TAM functions as an oncogene that expresses itself inappropriately in malignant cells and is essential for the migration of apoptotic bodies as well as the growth and survival of cells. The TAM encodes three tam receptors: Tyro3, AXL, and MerTK are proteins known to bind specifically to vitamin K-dependent protein S, with Tyro3 and MerTK showing an exclusive affinity for it, which triggers in T cells upon exposure to interleukin-4, while growth arrest protein 6 (Gas6) is another ligand that interacts to tam receptors. By binding to ligands, the team receptors are activated via the RTK pathway, which in turn causes the kinetic domains to become auto-phosphorylated, triggering signaling cascades and gene regulation.133
4.3. Molecular role of PS in cancer therapy
Fracturing PS and its receptors is one possible avenue for cancer treatment. Clinical efforts are conducted using two methods to inactivate PS and increase antitumor activity: interrupting PS in tumor cells and aiming at PS receptors to interfere with receptor signaling. PS and its anti-cancer effects can be bound by various agents, such as liposomal vesicles (SapC-DOPS), monoclonal antibodies (bavituximab), and antibodies conjugated with medicines (monomethyl auristatin E coupled with Fc-Syt1 and Annexin-V coupled with L-methionase).30,88 These agents work by forcing cancer cells to undergo apoptosis and controlling the body's immune system. These innovative treatments are revolutionizing the way cancer is treated and offering hope for the creation of new drugs. The PS receptors can be the target of a wide variety of compounds. Some of the compounds that can bind to and block all three of the TAM receptors are sitravatinib, LDC1267, and RXDX-106. R428 (BGB324), TP0903, KIT, FLT3, VEGFR, PDGFR, BMS777607, NPS-1034, and DP3975 are a few receptors for Axl. The MerTK inhibitors are UNC2025, UNC3133, ONO747, and MRX-2843. In addition, Pfizer-11 and 12, KRCT-7j, GL21.T, 20G7-D9, and AXL-107-MMAE are among the Tyro3 prohibitors. These are strong protein- or c small molecule inhibitors that block TAM receptors, limiting the spread and invasion of metastatic cells in the cancer microenvironment and boosting the activity of the innate and adaptive immune cells.105,117,134 Due to their anti-tumor properties, these compounds have created new therapeutic opportunities. They can be utilized to treat malignancies of the breast, oral, cervix, lung, and pancreas in addition to lymphocyte leukemia (Tables 3 and 4).
Table 3 Small chemical inhibitors of several kinases to target TAM30,88,105,117
Agents |
Primary target (IC50 value) |
TYRO3 IC50 |
MER IC50 |
AXL IC50 |
Development stage |
Primary indication |
Bosutinib |
BCR/ABL |
|
|
0.6 nM |
Approved |
Chronic myelogenous leukemia |
Cabozantinib |
MET VEGFR2 |
|
|
294 |
Approved |
Medullary thyroid cancer renal cell carcinoma hepatocellular carcinoma |
Crizotinib |
c-MET (8 nM) ALK (20 nM) |
>10 000 |
|
7 nM |
Approved |
Non small cell lung cancer |
LDC1267 |
AXL, MER, TYRO3 |
8 nM |
<5 nM |
29 nM |
Preclinical |
Boosting NK cell activity in tumor microenvironment |
RXDX-106 |
AXL, MER, TYRO3, MET |
19 nM |
29 nM |
7 nM |
Termination of Phase 1 |
Immune activation in tumor microenvironment |
Sitravatinib (MGCD516) |
VEGF, c-MET, AXL, MER, TYRO3 |
|
2 nM |
1.5 nM |
Phase 2 |
A potent multi-kinase inhibitor in different models of sarcoma |
Table 4 TAM-targeting selective small molecule inhibitors
Agents |
Primary target (IC50 value) |
TYRO3 IC50 |
MER IC50 |
AXL IC50 |
Development stage |
Primary indication |
Small molecule inhibitors for targeting MER |
MRX-2843 |
MER, FLT3 |
17 nM |
1.3 nM |
15 nM |
To overcome resistance conferring FLT3 mutations in AML |
Phase 1 |
UNC-2025 |
MER, FLT3(0.80 nM) |
17 nM |
0.74 nM |
14 nM |
Leukemia |
Preclinical |
UNC-3133 |
MER |
31 nM |
3.0 nM |
17 nM |
Cancer (FLT IC50 = 6.8 nM), virus infection |
Preclinical |
ONO-7475 |
AXL, MER, FLT3 |
|
0.4 nM |
2.2 nM |
AML |
Phase 1 |
|
Small molecule inhibitors for targeting AXL |
R428 (BGB324) |
AXL |
|
|
14 nM |
Cancer |
Phase 2 |
TP-0903 |
AXL |
|
|
19 nM |
Chronic lymphocytic leukemia |
Phase ½ |
BMS-777607 |
AXL, MET(3.9 nM) |
4.3 nM |
14 nM |
1.1 nM |
Met-dependent gastric carcinoma |
Phase 2 completed |
NPS1034 |
AXL, MET(0.80 nM) |
|
|
48 nM |
EGFR-resistant lung cancer cells due to MET or AXL activity |
Preclinical |
|
Small molecule inhibitors for targeting TYRO3 |
Pfizer compound 11 |
TYRO3 |
0.52 |
<10 fold than TYRO3 |
|
Thrombosis |
Preclinical |
Pfizer compound 12 |
TYRO3 |
0.27 |
<10 fold than TYRO3 |
10–100 fold than TYRO3 |
Thrombosis |
Preclinical |
Pfizer compound 19 |
TYRO3 |
0.010 |
>100 fold than TYRO3 |
>100 fold than TYRO3 |
Thrombosis |
Preclinical |
Pfizer compound 21 |
TYRO3 |
0.0007 |
10–100 fold than TYRO3 |
>100 fold than TYRO3 |
Thrombosis |
Preclinical |
KRCT-6j |
TYRO3 |
0.028 |
12 |
>10 |
Cancer |
Preclinical |
Recent research indicates that PS plays a role in the advancement of tumors. PS is externalized to the outer plasma membrane in different cancer cells; this process is controlled by calcium-dependent membrane proteins, such as flippases and scramblases. The development of tumors and resistance to chemotherapy and radiation therapy have been connected to the externalization of PS. However, PS's possible use in cancer treatment has also been investigated. According to a preclinical investigation, PS-targeting medications exhibit anticancer properties both on their own and in combination with conventional antitumor medications, where they exhibit greater potency.132,134,135 The mechanisms of PS in cancer therapy are complex and multifaceted. PS-targeting agents have been shown to be enhanced. Furthermore, it has been discovered that administering an antibody that targets PS improves overall survival and amplifies the anti-tumor efficacy of tumor-directed radiation therapy. PS-targeting agents have also been explored as a potential cancer immunotherapy strategy, with one study showing that blocking PS externalization can restore pathogen and tumor immunity.33,34,136,137 Furthermore, PS synthase has been recognized as a promising therapeutic target for regulating cellular homeostasis in cancer cells.
Numerous clinical investigations have been carried out to explore the possibilities of PS-targeting medications in the management of cancer. Patients with HER2-negative breast cancer were treated with the PS-targeting combination of paclitaxel and bavituximab in a phase I clinical study.18,108 It has been demonstrated that SapC-DOPS can efficiently target and eradicate several cancer types, including skin, lung, brain, and pancreatic cancer. Clinical trials have also shown that treatment with an antibody that targets PS improves overall survival and increases the effectiveness of tumor-directed radiation therapy. These findings imply that PS-targeting drugs may represent a fresh and exciting development in cancer treatment.
4.4. PS and PC in host immunity
PS and PC are pivotal to host immunity. A significant part of the cell membrane that separates the intracellular and extracellular environments is made up of phospholipids. In addition to their structural role, phospholipids also have signalling functions, including the modulation of immune responses.126,138,139
PS is vital in the identification and elimination of apoptotic cells to preserve immunological tolerance and to avert autoimmunity. PS is also involved in activating immune cells, including natural killer cells and macrophages, to protect the body against bacterial and viral infections.140,141 Exposure to PS causes pro-inflammatory cytokines to be downregulated and anti-inflammatory cytokines to be released, which resolve inflammation. In addition to its role in apoptosis, PS also plays a role in the activation of immune cells. PS can activate natural killer (NK) cells, which are a type of lymphocyte that can kill virus-infected or tumor cells without prior sensitization.142–144 PS also enhances the phagocytic activity of macrophages and stimulates the production of reactive oxygen species (ROS), which can kill bacteria and other pathogens.
Research has shown that PS supplementation can enhance the immune response in individuals with weakened immune systems.105,131 In a study involving elderly individuals, PS supplementation was found to improve the function of immune cells and reduce the incidence of infection. Other studies have shown that PS supplementation can boost the immune response to vaccinations and increase antibody production, which is essential for long-term protection against infectious diseases.145–147
However, PC is the most abundant phospholipid in cell membranes and is also a significant component of lipoproteins, which transport lipids in the bloodstream. PC has been shown to modulate immune responses in several ways.42 For instance, PC can prevent the synthesis of pro-inflammatory cytokines, including interleukin-1 beta (IL-1β) and tumor necrosis factor-alpha (TNF-α).42 Additionally, PC can increase the synthesis of cytokines that reduce inflammation, such as transforming growth factor beta (TGF-β) and interleukin-10 (IL-10).148 PC also affects lymphocytes through immunomodulatory means. PC can inhibit the activation of T cells, which are involved in cell-mediated immunity. PC can also enhance the proliferation of B cells, which produce antibodies in the body.149 PC has also been shown to improve the activity of the complement system, which is a group of proteins that plays a crucial role in the immune response. The complement system helps to identify and eliminate pathogens, and deficiencies in this system can lead to increased susceptibility to infections. Furthermore, PC can modulate the function of dendritic cells, which are specialized antigen-presenting cells that initiate adaptive immune responses.148,150
Research has shown that PC supplementation can enhance the function of system immune cells: T cells and natural killer cells. PC can also reduce inflammation, which is a crucial aspect of the immune response.93,104 In a study involving patients with ulcerative colitis, PC supplementation was found to reduce inflammation and improve symptoms. Further research is required to determine the optimal doses and duration of supplementation for different populations. PS and PC also play crucial roles in cancer immunity. Cancer cells can evade the immune system by expressing surface molecules that suppress immune cell activation and induce the apoptosis of immune cells.105 PS and PC can modulate the immune responses to cancer cells by regulating the activation and proliferation of immune cells and by promoting the phagocytosis of cancer cells.
PS is also flipped out on the surface of cancer cells, acting as an “eat-me” signal that is recognized by immune system cells such as phagocytic cells, such as macrophages and dendritic cells.140,141 PS activates NK cells, which recognize and kill cancer cells with low amounts of major histocompatibility complex (MHC) class I molecules, which are necessary for antigen presentation to T cells. PS also has immunomodulatory effects on dendritic cells, which are specialized antigen-presenting (sAPC) cells that are used to initiate adaptive immune responses.151 PS can enhance the antigen-presenting capacity of dendritic cells and promote the differentiation of r Th cells towards a Th1 phenotype. Th1 cells produce cytokines, such as IFN-γ, which activate macrophages and improve their ability to kill cancer cells.
PC can also modulate the function of T cells, which are involved in cell-mediated immunity. PC can inhibit the activation and proliferation of T cells, reducing the immune response to cancer cells. However, PC can also enhance the differentiation of regulatory T cells (Tregs), which can impair the activation and proliferation of the effector T cells. Tregs are essential for maintaining immune tolerance, but they can also nurture cancer growth by suppressing the immune response to regulate cancer cell proliferation.152 However, the role of PC in the case of cancer immunity is complex and context-dependent, and further research is needed to understand the mechanisms of the action of PC in cancer immunity.
4.5. Signaling cascade influenced by PS and PC
PS and PC play critical roles in regulating cell signalling pathways in cancer. Aberrant signalling cascades are a hallmark of cancer, and targeting these pathways is a promising approach for cancer therapy. PS and PC have been shown to influence various dysregulated signalling cascades in cancer cells, making them attractive targets for targeted cancer therapy.
PS and PC influence the PI3K/AKT/mTOR cell signaling pathway. These cell signalings, which regulate cell growth, survival, and metabolism, are frequently dysregulated in cancer cells.153 PS can initiate the PI3K/AKT pathway by promoting the recruitment of AKT to the plasma membrane. The AKT activation in turn phosphorylates and activates the downstream targets, including mTOR, which promotes cell growth and proliferation. PC has also been shown to influence the PI3K/AKT/mTOR pathway.153 Studies have shown that PC can activate AKT by promoting the activation of phosphoinositide-dependent kinase-1 (PDK1), which is an upstream regulator of AKT. PC has also been shown to regulate mTOR activity by modulating the expression of mTOR regulatory proteins.
Targeting the PI3K/AKT/mTOR signaling pathway has been the focus of many cancer drug development efforts.153 Several medications targeting this system, including PI3K inhibitors, AKT inhibitors, and mTOR inhibitors, are now under clinical studies. However, the efficiency of these drug molecules has limitations, such as off-target effects and drug resistance.154 Targeting PS and PC may provide a novel approach for modulating this pathway in cancer cells.
Another signaling cascade that PS and PC influence is the JAK/STAT pathway.155 This pathway plays a role in controlling cell growth, differentiation, and immune function, and it is often dysregulated in cancer cells.156 PS can activate the JAK/STAT signalling pathway by inducing Janus kinase (JAK), followed by the phosphorylation and activation of signal transducer and activator of transcription (STAT) proteins. PC also modulates the JAK/STAT pathway by activating STAT proteins and promoting their phosphorylation and nuclear translocation.156 PC has also been shown to regulate the expression of JAK proteins, which may impact the activation of this pathway.
Targeting the signalling pathways of JAK/STAT has been the focus of many cancer drug development efforts. Several drugs targeting this pathway, including inhibitors of both JAK and STAT, have recently been used in clinical trials.155 However, the efficacy of these inhibitors is often limited by off-target effects and possibly shows drug resistance. Targeting PS and PC may provide a novel approach for modulating this pathway in cancer cells.
In addition to both the JAK/STAT and PI3K/AKT/mTOR signaling pathways, PS and PC have been shown to influence other signaling cascades that are dysregulated in cancer cells.155 For example, PS has been shown to turn on the MAPK/ERK signalling pathway, which is the key regulator pathway for both cell growth and cell differentiation. Again, PC has been reported to regulate developmental pathways, such as the Wnt/β-catenin pathway, which plays an important role and is also involved in the regulation of cell differentiation and cell proliferation.
Targeting PS and PC for cancer therapy has several advantages over traditional approaches that target specific proteins or pathways.134,157,158 First, PS and PC are ubiquitous components of cell membranes, making them essential for cell survival. Therefore, targeting these phospholipids may have fewer off-target effects than drugs that target specific proteins or pathways. Second, PS and PC are highly expressed in cancer cells, making them attractive targets for cancer-specific therapies.134,144,159 Targeting PS and PC may overcome drug resistance mechanisms that often arise with targeted therapies, as cancer cells may develop resistance to a specific protein or pathway but are unlikely to develop resistance to fundamental components of cell membranes.
Several strategies for targeting PS and PC for cancer therapy have been developed. One approach is to use agents that specifically bind to these phospholipids and initiate apoptotic pathways in cancer cells.111,112 For example, some monoclonal antibodies, such as bavituximab, bind to PS and have been shown to prompt apoptosis in the case of cancer cells by activating the immune system. Another approach is to use lipid-based delivery of drugs to cancer cells through nanoparticles.33,134,160 These engineered small particles are used to target PS and PC on the surface of cancer cells, allowing for the selective delivery of drugs. The current era of anti-cancer drugs with liposomal formulations, such as doxorubicin and paclitaxel, has developed target PS on the surface of cancer cells and has depicted promising results in the case of preclinical studies.32,159,161 Finally, targeting the enzymes that synthesize PS and PC may be a promising approach for cancer therapy. For example, the enzyme PSS is responsible for the synthesis of PS, and inhibitors of PS have been reported to instigate apoptotic events in cancer cells. Similarly, the enzyme choline kinase (CK), which is responsible for the synthesis of PC, is often overexpressed in cancer cells, and inhibitors of CK have demonstrated potential results in preclinical studies. These approaches have been shown to have promising results in the case of preclinical studies, and they can offer a new avenue for developing cancer therapies.
6. Future perspective and challenges
PS has emerged as a pivotal molecule with transformative potential in the landscape of cancer therapies. As we delve deeper into understanding its intricate molecular functions and dynamic role in cancer progression, several emerging trends and future directions come to light. They are as follows:
Role of PS in cancer progression
One of the critical areas of focus in the future will be unravelling the precise role of PS in driving cancer progression. It is similarly involved in crucial cellular processes, such as proliferation, apoptosis evasion, and metastasis formation. Understanding these mechanisms provides valuable insights into designing targeted therapies that specifically disrupt PS-mediated pathways.
Dynamic interplay within the cancer microenvironment
The cancer microenvironment plays a vital role in tumor development and response to treatment. Investigating how PS actively contributes to the complex interplay within this microenvironment is a crucial area of research. Its interactions with immune cells, stromal cells, and signaling molecules offer new avenues for innovative therapeutic strategies that leverage these interactions.
Harnessing PS for targeted cancer therapies
Future research will focus on developing novel methodologies and approaches that harness the potential of PS for targeted cancer therapies. Exploring PS-targeted drug delivery systems, immunotherapies targeting PS-expressing cells, and combination therapies that synergize with PS-mediated pathways can intensify treatment efficacy and impair side effects.
Age-dependent implications of novel PS in cancer
Understanding the age-dependent implications of PS in cancer is crucial for personalized treatment strategies. Senescence-related changes in PS expression and function may impact the treatment response and toxicity profiles. Tailoring therapies based on age-specific considerations will be an essential aspect of future cancer care.
Interactions with mast cells and microglia
The intricate crosstalk between PS, mast cells, and microglia presents a promising avenue for therapeutic intervention. Investigating how PS influences immune cell behavior within the tumor microenvironment can lead to the development of immunomodulatory strategies that enhance anti-tumor immune responses.
Future horizons for next-generation cancer therapeutics
PS has tremendous potential as a cornerstone for next-generation cancer therapeutics. Continued research efforts aimed at elucidating its multifaceted roles, exploring innovative targeting approaches, and integrating age-specific and microenvironmental considerations will pave a new direction for more effective and personalized treatments for cancer.
Despite promising advancements, several challenges remain. The precise delivery of PS-targeted therapies, the heterogeneity of PS expression across different cancer types, and the need for a deeper understanding of PS's role in various cellular contexts are critical areas that require further investigation. Additionally, integrating PS-targeted therapies with existing treatment modalities to maximize therapeutic efficacy and minimize adverse effects presents a complex yet rewarding challenge.
Looking forward, the future of PS in cancer therapy is bright. With ongoing research and technological advancements, the potential for PS-targeted therapies to revolutionize cancer treatment is immense. By continuing to unravel the complexities of PS and its interactions within the tumor microenvironment, researchers can develop more effective, targeted, and personalized cancer treatments. Overall, phosphatidylserine stands at the cusp of ushering in a new paradigm in cancer therapy. Its unique properties and significant role in cancer biology offer a promising avenue for developing novel therapeutic strategies. As our understanding of PS deepens, so too does the potential for groundbreaking advancements in the fight against cancer, paving the way for a future in which cancer therapies are more effective, less invasive, and tailored to the individual needs of patients.
7. Conclusion
In tumor microenvironments, PS represents a transformative frontier in the field of cancer therapies, offering unprecedented insights and opportunities for innovative treatments. As this review has illustrated, PS is not just a structural component of cell membranes but a pivotal player in the intricate communication networks within and between cells, particularly in the context of cancer. The externalization of PS on cancer cells and apoptotic cells reveals its crucial role in immune evasion and tumor progression, making it an attractive target for therapeutic intervention. The diverse biological functions of PS, from modulating immune responses to influencing cellular signaling pathways, underscore its potential as a multifaceted therapeutic target. Current research efforts, including the development of PS-targeting antibodies and PS-binding proteins, have shown promise in disrupting these processes, thereby enhancing the ability of the immune system to recognize and eliminate cancer cells. Beyond these applications, PS is also used in antibody–drug conjugates, where it acts as a cytotoxic agent in PS-targeting antibodies to exert tumor-killing effects. These strategies, while still in the experimental stages, herald a new era of precision medicine in which treatments can be tailored to exploit the unique characteristics of cancer cells. Additional research is needed on cells and agents that detect PS exposure levels, together with other cancer medicines.
Consent for publication
All authors have read and approved the final manuscript.
Data availability
Data will be available upon request.
Conflicts of interest
The authors declare that there are no potential competing interests.
Acknowledgements
The authors acknowledge respective departments and institutions that provide facilities and support. The authors would also like to express their appreciation for the Biorender.com software.
References
- L. A. Garraway and E. S. Lander, Lessons from the cancer genome, Cell, 2013, 153(1), 17–37 CrossRef CAS PubMed.
- K. L. Britt, J. Cuzick and K.-A. Phillips, Key steps for effective breast cancer prevention, Nat. Rev. Cancer, 2020, 20(8), 417–436 CrossRef CAS.
- H. K. Matthews, C. Bertoli and R. A. de Bruin, Cell cycle control in cancer, Nat. Rev. Mol. Cell Biol., 2022, 23(1), 74–88 CrossRef CAS PubMed.
- D. Patel,
et al., Equality, diversity, and inclusion in oncology clinical trials: an audit of essential documents and data collection against INCLUDE under-served groups in a UK academic trial setting, BMC Med. Ethics, 2023, 24(1), 105 CrossRef PubMed.
- R. Birge,
et al., Phosphatidylserine is a global immunosuppressive signal in efferocytosis, infectious disease, and cancer, Cell Death Differ., 2016, 23(6), 962–978 CrossRef CAS PubMed.
- F. Bray,
et al., Global cancer statistics 2018: GLOBOCAN estimates of incidence and mortality worldwide for 36 cancers in 185 countries, Ca-Cancer J. Clin., 2018, 68(6), 394–424 CrossRef PubMed.
- C. I. Ene and S. D. Ferguson, Surgical management of brain metastasis: challenges and nuances, Front. Oncol., 2022, 12, 847110 CrossRef.
- L. Gandhi,
et al., Pembrolizumab plus chemotherapy in metastatic non–small-cell lung cancer, N. Engl. J. Med., 2018, 378(22), 2078–2092 CrossRef CAS PubMed.
- H. Takeyama,
et al., Impact of surgical treatment after sorafenib therapy for advanced hepatocellular carcinoma, Surg. Today, 2018, 48, 431–438 CrossRef.
- A. K. Basu, DNA Damage, Mutagenesis and Cancer, Int. J. Mol. Sci., 2018, 19(4), 970 CrossRef PubMed.
- N. M. Novikov,
et al., Mutational drivers of cancer cell migration and invasion, Br. J. Cancer, 2021, 124(1), 102–114 CrossRef.
- E. Laconi, F. Marongiu and J. DeGregori, Cancer as a disease of old age: changing mutational and microenvironmental landscapes, Br. J. Cancer, 2020, 122(7), 943–952 CrossRef PubMed.
- A. Ale,
et al., Cardioprotective C-kit+ bone marrow cells attenuate apoptosis after acute myocardial infarction in mice-in-vivo assessment with fluorescence molecular imaging, Theranostics, 2013, 3(11), 903 CrossRef PubMed.
- J. Chen,
et al., Dual-targeting theranostic system with mimicking apoptosis to promote myocardial infarction repair via modulation of macrophages, Theranostics, 2017, 7(17), 4149 CrossRef PubMed.
- W. Chang,
et al., Targeting phosphatidylserine for Cancer therapy: prospects and challenges, Theranostics, 2020, 10(20), 9214–9229 CrossRef PubMed.
- D. T. Debela,
et al., New approaches and procedures for cancer treatment: Current perspectives, SAGE Open Med., 2021, 9, 20503121211034366 Search PubMed.
- N. Thapa,
et al., Discovery of a phosphatidylserine-recognizing peptide and its utility in molecular imaging of tumour apoptosis, J. Cell. Mol. Med., 2008, 12(5a), 1649–1660 CrossRef CAS PubMed.
- A. Kaynak,
et al., Phosphatidylserine: The unique dual-role biomarker for cancer imaging and therapy, Cancers, 2022, 14(10), 2536 CrossRef CAS.
- H. Jing,
et al., Microparticle phosphatidylserine mediates coagulation: involvement in tumor progression and metastasis, Cancers, 2023, 15(7), 1957 CrossRef CAS PubMed.
- S. D. Vallabhapurapu,
et al., Variation in human cancer cell external phosphatidylserine is regulated by flippase activity and intracellular calcium, Oncotarget, 2015, 6(33), 34375–34388 CrossRef.
-
M. Mahapatra, et al., Role of Biosurfactants in Heavy Metal Removal and Mineral Flotation, in Biotechnological Innovations in the Mineral-Metal Industry, Springer, 2024, pp. 141–150 Search PubMed.
- S. S. Naser,
et al., Posterity of nanoscience as lipid nanosystems for Alzheimer's disease regression, Mater. Today Bio, 2023, 100701 CrossRef CAS.
- S. Riedl,
et al., In search of a novel target - phosphatidylserine exposed by non-apoptotic tumor cells and metastases of malignancies with poor treatment efficacy, Biochim. Biophys. Acta, 2011, 1808(11), 2638–2645 CrossRef CAS PubMed.
- W. Chang,
et al., Targeting phosphatidylserine for Cancer therapy: prospects and challenges, Theranostics, 2020, 10(20), 9214 CrossRef CAS.
- G. I. Perez,
et al., Phosphatidylserine-Exposing Annexin A1-Positive Extracellular Vesicles: Potential Cancer Biomarkers, Vaccines, 2023, 11(3), 639 CrossRef CAS.
- A. Kaynak,
et al., Phosphatidylserine: The Unique Dual-Role
Biomarker for Cancer Imaging and Therapy, Cancers, 2022, 14(10), 2536 CrossRef CAS.
- T. Kawakami, S. Takeuchi and Y. Soma, Elevated levels of serum IgM anti-phosphatidylserine-prothrombin complex antibodies in patients with cancer-associated vasculitis, Int. J. Dermatol., 2017, 56(10), e203–e204 CrossRef CAS.
- T. Sakuragi and S. Nagata, Regulation of phospholipid distribution in the lipid bilayer by flippases and scramblases, Nat. Rev. Mol. Cell Biol., 2023, 24(8), 576–596 CrossRef CAS PubMed.
- L. G. Lima,
et al., Tumor-derived microvesicles modulate the establishment of metastatic melanoma in a phosphatidylserine-dependent manner, Cancer Lett., 2009, 283(2), 168–175 CrossRef CAS.
- X. Qi,
et al., Cancer-selective targeting and cytotoxicity by liposomal-coupled lysosomal saposin C protein, Clin. Cancer Res., 2009, 15(18), 5840–5851 CrossRef CAS.
-
A. T. Da Poian, et al., The Families of Biological Molecules, Integrative Human Biochemistry: A Textbook for Medical Biochemistry, 2021, pp. 89–185 Search PubMed.
- M. I. Fakai, S. N. Abd Malek and S. A. Karsani, Induction of apoptosis by chalepin through phosphatidylserine externalisations and DNA fragmentation in breast cancer cells (MCF7), Life Sci., 2019, 220, 186–193 CrossRef CAS PubMed.
- A. Bandyopadhyay,
et al., Ligand-based active targeting strategies for cancer theranostics, Naunyn-Schmiedeberg’s Arch. Pharmacol., 2023, 396, 3417–3441 CrossRef PubMed.
- S. Preetam, Nano Revolution: Pioneering the Future of Water Reclamation with Micro/Nanorobots, Nanoscale Adv., 2024, 6, 2569–2581 RSC.
- S. Preetam,
et al., Therapeutic potential of Lipid Nanosystems for the treatment of Parkinson's disease: an updated review, Ageing Res. Rev., 2023, 101965 CrossRef PubMed.
-
S. Preetam, et al., Functionalized exosomes for cancer therapy, in Functionalized Nanomaterials for Cancer Research, Elsevier, 2024, pp. 167–180 Search PubMed.
- J. E. Vance and R. Steenbergen, Metabolism and functions of phosphatidylserine, Prog. Lipid Res., 2005, 44(4), 207–234 CrossRef PubMed.
- J. E. Vance and G. Tasseva, Formation and function of phosphatidylserine and phosphatidylethanolamine in mammalian cells, Biochim. Biophys. Acta, Mol. Cell Biol. Lipids, 2013, 1831(3), 543–554 CrossRef PubMed.
- K. Schutters and C. Reutelingsperger, Phosphatidylserine targeting for diagnosis and treatment of human diseases, Apoptosis, 2010, 15, 1072–1082 CrossRef PubMed.
- M. B. Naeini,
et al., The role of phosphatidylserine recognition receptors in multiple biological functions, Cell. Mol. Biol. Lett., 2020, 25, 1–17 CrossRef.
- H. Ribeiro,
et al., Apoptosis and (in) pain—potential clinical implications, Biomedicines, 2022, 10(6), 1255 CrossRef PubMed.
- J. G. Kay and S. Grinstein, Sensing phosphatidylserine in cellular membranes, Sensors, 2011, 11(2), 1744–1755 CrossRef PubMed.
-
D. G. Maloney, B. Smith and A. Rose, Rituximab: mechanism of action and resistance, Seminars in oncology, Elsevier, 2002 Search PubMed.
- K. Wirtz, Phospholipid transfer proteins, Annu. Rev. Biochem., 1991, 60(1), 73–99 CrossRef PubMed.
-
W. Christie, Phosphatidylcholine and related lipids: structure, occurrence, biochemistry and analysis, Lipidlibrary, AOCS, 2010 Search PubMed.
- E. P. Kennedy and S. B. Weiss, The function of cytidine coenzymes in the biosynthesis of phospholipides, J. Biol. Chem., 1956, 222(1), 193–214 CrossRef.
-
D. E. Vance and J. E. Vance, Biochemistry of lipids, lipoproteins and membranes, Elsevier, 2002, vol. 36 Search PubMed.
- J. E. Vance, Phospholipid synthesis in a membrane fraction associated with mitochondria, J. Biol. Chem., 1990, 265(13), 7248–7256 CrossRef.
- D. J. Shields,
et al., Membrane topography of human phosphatidylethanolamine N-methyltransferase, J. Biol. Chem., 2003, 278(5), 2956–2962 CrossRef PubMed.
- W. Szlasa,
et al., Lipid composition of the cancer cell membrane, J. Bioenerg. Biomembr., 2020, 52, 321–342 CrossRef.
- G. Lenoir,
et al., Transport pathways that contribute to the cellular distribution of phosphatidylserine, Front. cell dev. biol., 2021, 9, 737907 CrossRef.
- H.-W. Shin and H. Takatsu, Phosphatidylserine exposure in living cells, Crit. Rev. Biochem. Mol. Biol., 2020, 55(2), 166–178 CrossRef PubMed.
- W. Wang,
et al., Mobilizing phospholipids on tumor plasma membrane implicates phosphatidylserine externalization blockade for cancer immunotherapy, Cell Rep., 2022, 41(5), 111582 CrossRef.
- R. Ventura, I. Martínez-Ruiz and M. I. Hernández-Alvarez, Phospholipid membrane transport and associated diseases, Biomedicines, 2022, 10(5), 1201 CrossRef PubMed.
- T. Skotland, S. Kavaliauskiene and K. Sandvig, The role of lipid species in membranes and cancer-related changes, Cancer Metastasis Rev., 2020, 39(2), 343–360 CrossRef PubMed.
- B. Li,
et al., Lipid raft involvement in signal transduction in cancer cell survival, cell death and metastasis, Cell Proliferation, 2022, 55(1), e13167 CrossRef PubMed.
- S. Cockcroft, Mammalian lipids: structure, synthesis and function, Essays Biochem., 2021, 65(5), 813–845 CrossRef PubMed.
- D. Xiao and W. Chang, Phosphatidylserine in diabetes research, Mol. Pharmaceutics, 2022, 20(1), 82–89 CrossRef PubMed.
- J. Wang,
et al., The role of phosphatidylserine on the membrane in immunity and blood coagulation, Biomark. Res., 2022, 10(1), 4 CrossRef PubMed.
- J. Rodencal and S. J. Dixon, A tale of two lipids: Lipid unsaturation commands ferroptosis sensitivity, Proteomics, 2023, 23(6), 2100308 CrossRef.
- M. Murata,
et al., Molecular substructure of the liquid-ordered phase formed by sphingomyelin and cholesterol: Sphingomyelin clusters forming nano-subdomains are a characteristic feature, Biophys. Rev., 2022, 14(3), 655–678 CrossRef PubMed.
- S. M. Medfisch,
et al., Phosphatidylethanolamine-phosphatidylserine binding synergy of seven coagulation factors revealed using Nanodisc arrays on silicon photonic sensors, Sci. Rep., 2020, 10(1), 17407 CrossRef PubMed.
- H. L. Scott,
et al., Model membrane systems used to study plasma membrane lipid asymmetry, Symmetry, 2021, 13(8), 1356 CrossRef PubMed.
- O. Engberg,
et al., Sphingomyelin acyl chains influence the formation of sphingomyelin-and cholesterol-enriched domains, Biophys. J., 2020, 119(5), 913–923 CrossRef CAS.
- C. Dias and J. Nylandsted, Plasma membrane integrity in health and disease: significance and therapeutic potential, Cell Discovery, 2021, 7(1), 4 CrossRef CAS.
- T. Bhattacharya,
et al., Advancement in biopolymer assisted cancer theranostics, ACS Appl. Bio Mater., 2023, 6(10), 3959–3983 CrossRef CAS PubMed.
- C. von Schacky, Importance of EPA and DHA blood levels in brain structure and function, Nutrients, 2021, 13(4), 1074 CrossRef PubMed.
- Y.-J. Park,
et al., Phosphatidylserine synthase plays an essential role in glia and affects development, as well as the maintenance of neuronal function, iScience, 2021, 24(8), 102899 CrossRef CAS.
- D. Sokolova, T. Childs and S. Hong, Insight into the role of phosphatidylserine in complement-mediated synapse loss in Alzheimer’s disease, Fac. Rev., 2021, 10(19) DOI:10.12703/r/10-19.
- D. Schumacher,
et al., Phosphatidylserine supplementation as a novel strategy for reducing myocardial infarct size and preventing adverse left ventricular remodeling, Int. J. Mol. Sci., 2021, 22(9), 4401 CrossRef CAS.
-
S. Preetam, et al., in Application of Nanobiosensor in Health Care Sector, in Bio-Nano Interface: Applications in Food, Healthcare and Sustainability, ed. M. Arakha, A. K. Pradhan and S. Jha, 2022, Springer Singapore, Singapore, pp. 251–270 Search PubMed.
- S. Preetam,
et al., Revolutionizing Cancer Treatment: The Promising Horizon of Zein Nanosystems, ACS Biomater. Sci. Eng., 2024, 10(4), 1946–1965 CrossRef CAS.
- S. Zargarian,
et al., Phosphatidylserine externalization,“necroptotic bodies” release, and phagocytosis during necroptosis, PLoS Biol., 2017, 15(6), e2002711 CrossRef PubMed.
- F. Wang,
et al., Effects on tumor growth and immunosuppression of a modified Tα1 peptide along with its circular dichroism spectroscopy data, Data Brief, 2018, 20, 126–131 CrossRef PubMed.
- M. Farhad, A. S. Rolig and W. L. Redmond, The role of Galectin-3 in modulating tumor growth and immunosuppression within the tumor microenvironment, Oncoimmunology, 2018, 7(6), e1434467 CrossRef.
- R. Rajan,
et al., Liposome-induced immunosuppression and tumor growth is mediated by macrophages and mitigated by liposome-encapsulated alendronate, J. Controlled Release, 2018, 271, 139–148 CrossRef CAS PubMed.
- G. L. Beatty and W. L. Gladney, Immune escape mechanisms as a guide for cancer immunotherapy, Clin. Cancer Res., 2015, 21(4), 687–692 CrossRef CAS PubMed.
-
B. Sharma and S. S. Kanwar. Phosphatidylserine: A cancer cell targeting biomarker, in Seminars in cancer biology, Elsevier, 2018 Search PubMed.
- P. Orning and E. Lien, Multiple roles of caspase-8 in cell death, inflammation, and innate immunity, J. Leukoc. Biol., 2021, 109(1), 121–141 CrossRef CAS PubMed.
- I.-M. Kur and A. Weigert, Phosphatidylserine externalization as immune checkpoint in cancer, Pflug. Arch. Eur. J. Physiol., 2024, 1–14 Search PubMed.
- C.-F. Lo,
et al., Targeting the phosphatidylserine-immune checkpoint with a small-molecule maytansinoid conjugate, J. Med. Chem., 2022, 65(19), 12802–12824 CrossRef CAS PubMed.
- P. Aehnlich,
et al., Tam Receptor inhibition–implications for cancer and the immune system, Cancers, 2021, 13(6), 1195 CrossRef CAS.
- C. Min,
et al., Tim-4 functions as a scavenger receptor for phagocytosis of exogenous particles, Cell Death Dis., 2020, 11(7), 561 CrossRef CAS.
- N. Luque-Campos,
et al., The macrophage response is driven by mesenchymal stem cell-mediated metabolic reprogramming, Front. Immunol., 2021, 12, 624746 CrossRef CAS PubMed.
- O. Belzile,
et al., Antibody targeting of phosphatidylserine for the detection and immunotherapy of cancer, ImmunoTargets Ther., 2018, 7, 1–14 CrossRef CAS.
- E.-B. Byun,
et al., Hesperidin structurally modified by gamma irradiation induces apoptosis in murine melanoma B16BL6 cells and inhibits both subcutaneous tumor growth and metastasis in C57BL/6 mice, Food Chem. Toxicol., 2019, 127, 19–30 CrossRef CAS.
- J. Oyler-Yaniv,
et al., Catch and release of cytokines mediated by tumor phosphatidylserine converts transient exposure into long-lived inflammation, Mol. Cell, 2017, 66(5), 635–647 CrossRef CAS PubMed.
- L. Zhang,
et al., Phosphatidylserine-targeted bimodal liposomal nanoparticles for in vivo imaging of breast cancer in mice, J. Controlled Release, 2014, 183, 114–123 CrossRef CAS PubMed.
- R. K. Kannadorai, S. K. Udumala and Y. W. K. Sidney, Noninvasive in vivo multispectral optoacoustic imaging of apoptosis in triple negative breast cancer using indocyanine green conjugated phosphatidylserine monoclonal antibody, J. Biomed. Opt., 2016, 21(12), 126002 CrossRef PubMed.
- T. Skotland,
et al., Molecular lipid species in urinary exosomes as potential prostate cancer biomarkers, Eur. J. Cancer, 2017, 70, 122–132 CrossRef CAS PubMed.
- J. Zhang,
et al., Lipids in surgical aerosol as diagnosis biomarkers for discrimination of lung cancer, Cancer Manage. Res., 2019, 5537–5543 CrossRef CAS.
- D. Zhao,
et al., Near-infrared optical imaging of exposed phosphatidylserine in a mouse glioma model, Transl. Oncol., 2011, 4(6), 355–364 CrossRef PubMed.
- V. M. Blanco, R. Curry and X. Qi, SapC-DOPS nanovesicles: a novel targeted agent for the imaging and treatment of glioblastoma, Oncoscience, 2015, 2(2), 102 CrossRef.
- Z. Chu,
et al., In vivo optical imaging of brain tumors and arthritis using fluorescent SapC-DOPS nanovesicles, J. Visualized Exp., 2014, 87, e51187 Search PubMed.
- P. M. Winter,
et al., Imaging of brain tumors with paramagnetic vesicles targeted to phosphatidylserine, J. Magn. Reson. Imaging, 2015, 41(4), 1079–1087 CrossRef PubMed.
- V. Ntziachristos,
et al., Visualization of antitumor treatment by means of fluorescence molecular tomography with an annexin V–Cy5. 5 conjugate, Proc. Natl. Acad. Sci. U. S. A., 2004, 101(33), 12294–12299 CrossRef CAS.
- F. G. Blankenberg,
et al., In vivo detection and imaging of phosphatidylserine expression during programmed cell death, Proc. Natl. Acad. Sci. U. S. A., 1998, 95(11), 6349–6354 CrossRef CAS.
-
E. Dumont, et al., Real-time imaging of apoptotic cell-membrane changes at the single-cell level in the beating murine heart, Nature Publishing Group, US New York, 2001 Search PubMed.
-
G. Koopman, et al., Annexin V for flow cytometric detection of phosphatidylserine expression on B cells undergoing apoptosis. 1994.
- I. Vermes,
et al., A novel assay for apoptosis flow cytometric detection of phosphatidylserine expression on early apoptotic cells using fluorescein labelled annexin V, J. Immunol. Methods, 1995, 184(1), 39–51 CrossRef CAS.
- C. P. Reutelingsperger, G. Hornstra and H. C. Hemker, Isolation and partial purification of a novel anticoagulant from arteries of human umbilical cord, Eur.
J. Biochem., 1985, 151(3), 625–629 CrossRef CAS.
- B. L. Kietselaer,
et al., Noninvasive detection of plaque instability with use of radiolabeled annexin A5 in patients with carotid-artery atherosclerosis, N. Engl. J. Med., 2004, 350(14), 1472–1473 CrossRef CAS.
- M. Jennewein,
et al., Vascular imaging of solid tumors in rats with a radioactive arsenic-labeled antibody that binds exposed phosphatidylserine, Clin. Cancer Res., 2008, 14(5), 1377–1385 CrossRef CAS.
- J. Wojton,
et al., Systemic delivery of SapC-DOPS has antiangiogenic and antitumor effects against glioblastoma, Mol. Ther., 2013, 21(8), 1517–1525 CrossRef CAS.
- S. Abu-Baker,
et al., Cytotoxicity and selectivity in skin cancer by SapC-DOPS nanovesicles, J. Cancer Ther., 2012, 3(4), 321 CrossRef PubMed.
- H. W. Davis,
et al., Enhanced phosphatidylserine-selective cancer therapy with irradiation and SapC-DOPS nanovesicles, Oncotarget, 2019, 10(8), 856 CrossRef PubMed.
- M. De,
et al., A novel therapeutic strategy for cancer using phosphatidylserine targeting stearylamine-bearing cationic liposomes, Mol. Ther.--Nucleic Acids, 2018, 10, 9–27 CrossRef CAS PubMed.
- H. Kenis, L. Hofstra and C. Reutelingsperger, Annexin A5: shifting from a diagnostic towards a therapeutic realm, Cell. Mol. Life Sci., 2007, 64, 2859–2862 CrossRef CAS.
- H. Kenis,
et al., Cell surface-expressed phosphatidylserine and annexin A5 open a novel portal of cell entry, J. Biol. Chem., 2004, 279(50), 52623–52629 CrossRef CAS PubMed.
- S. Ran and P. E. Thorpe, Phosphatidylserine is a marker of tumor vasculature and a potential target for cancer imaging and therapy, Int. J. Radiat. Oncol., Biol., Phys., 2002, 54(5), 1479–1484 CrossRef CAS.
- X. Huang, M. Bennett and P. E. Thorpe, A monoclonal antibody that binds anionic phospholipids on tumor blood vessels enhances the antitumor effect of docetaxel on human breast tumors in mice, Cancer Res., 2005, 65(10), 4408–4416 CrossRef CAS PubMed.
- T. A. Luster,
et al., Plasma protein β-2-glycoprotein 1 mediates interaction between the anti-tumor monoclonal antibody 3G4 and anionic phospholipids on endothelial cells, J. Biol. Chem., 2006, 281(40), 29863–29871 CrossRef CAS PubMed.
- G. M. Willems,
et al., Competition of annexin V and anticardiolipin antibodies for binding to phosphatidylserine containing membranes, Biochemistry, 2000, 39(8), 1982–1989 CrossRef CAS.
- S. Budhu,
et al., Targeting phosphatidylserine enhances the anti-tumor response to tumor-directed radiation therapy in a preclinical model of melanoma, Cell Rep., 2021, 34(2), 108620 CrossRef CAS.
- T. Kawakami,
et al., High titer of serum antiphospholipid antibody levels in adult Henoch-Schönlein purpura and cutaneous leukocytoclastic angiitis, Arthritis Care Res., 2008, 59(4), 561–567 CrossRef.
- C. Schuck-Paim,
et al., Effect of pneumococcal conjugate vaccine introduction on childhood pneumonia mortality in Brazil: a retrospective observational study, Lancet Global Health, 2019, 7(2), e249–e256 CrossRef.
- R. Advani,
et al., CD47 blockade by Hu5F9-G4 and rituximab in non-Hodgkin's lymphoma, N. Engl. J. Med., 2018, 379(18), 1711–1721 CrossRef CAS.
- R. Li,
et al., Targeting Phosphatidylserine with Calcium-Dependent Protein–Drug Conjugates for the Treatment of Cancer, Mol. Cancer Ther., 2018, 17(1), 169–182 CrossRef CAS PubMed.
- S. Guan,
et al., Phosphatidylserine targeting peptide-functionalized pH sensitive mixed micelles for enhanced anti-tumor drug delivery, Eur. J. Pharm. Biopharm., 2020, 147, 87–101 CrossRef CAS.
- B. D. Van Rite,
et al., Enzyme prodrug therapy designed to target L-methioninase to the tumor vasculature, Cancer Lett., 2011, 301(2), 177–184 CrossRef CAS PubMed.
- P.-A. Oldenborg, CD47: a cell surface glycoprotein which regulates multiple functions of hematopoietic cells in health and disease, Int. Scholarly Res. Not., 2013, 1, 614619 Search PubMed.
- E. R. Unanue, Perspectives on anti-CD47 antibody treatment for experimental cancer, Proc. Natl. Acad. Sci. U. S. A., 2013, 110(27), 10886–10887 CrossRef CAS.
- H. Haubelt,
et al., Variables influencing Platelet Function Analyzer-100TM closure times in healthy individuals, Br. J. Haematol., 2005, 130(5), 759–767 CrossRef PubMed.
- D. Ennishi,
et al., TMEM30A loss-of-function mutations drive lymphomagenesis and confer therapeutically exploitable vulnerability in B-cell lymphoma, Nat. Med., 2020, 26(4), 577–588 CrossRef CAS.
- S. Nallanthighal,
et al., Inhibition of collagen XI alpha 1-induced fatty acid oxidation triggers apoptotic cell death in cisplatin-resistant ovarian cancer, Cell Death Dis., 2020, 11(4), 258 CrossRef CAS PubMed.
- S. Qian,
et al., The role of BCL-2 family proteins in regulating apoptosis and cancer therapy, Front. Oncol., 2022, 12, 985363 CrossRef CAS PubMed.
- M. Ahmadi-Kashani, H. Dehghani and A. Zarrabi, A biocompatible nanoplatform formed by MgAl-layered double hydroxide modified Mn3O4/N-graphene quantum dot conjugated-polyaniline for pH-triggered release of doxorubicin, Mater. Sci. Eng., C, 2020, 114, 111055 CrossRef CAS PubMed.
- R. Bhattacharjee,
et al., Synergy of nanocarriers with CRISPR-Cas9 in an emerging technology platform for biomedical appliances: Current insights and perspectives, Mater. Des., 2022, 224, 111415 CrossRef CAS.
- R. Bhattacharjee,
et al., Theragnostic application of nanoparticle and CRISPR against food-borne multi-drug resistant pathogens, Mater. Today Bio, 2022, 15, 100291 CrossRef CAS PubMed.
- X. Qin,
et al., Artificial Nucleotide-containing Aptamers Used in Tumor Therapy, Chem. Res. Chin. Univ., 2020, 36(2), 164–170 CrossRef CAS.
- E. M. Ahmed, Hydrogel: Preparation, characterization, and applications: A review, J. Adv. Res., 2015, 6(2), 105–121 CrossRef CAS PubMed.
- T. Bansal,
et al., Novel formulation approaches for optimising delivery of anticancer drugs based on P-glycoprotein modulation, Drug Discovery Today, 2009, 14(21–22), 1067–1074 CrossRef CAS PubMed.
- K. V. Myers, S. R. Amend and K. J. Pienta, Targeting Tyro3, Axl and MerTK (TAM receptors): implications for macrophages in the tumor microenvironment, Mol. Cancer, 2019, 18(1), 1–14 CrossRef.
- M. Aggarwal and S. Kumar, The Use of Nanorobotics in the Treatment Therapy of Cancer and Its Future Aspects: A Review, Cureus, 2022, 14(9), e29366 Search PubMed.
- I. F. Tannock,
et al., Limited Penetration of Anticancer Drugs through Tumor Tissue, Clin. Cancer Res., 2002, 8, 878–884 CAS.
- P. MIttal,
et al., Unlocking the power of nanomedicine: the future of nutraceuticals in oncology treatment, Front. Nutr., 2023, 10, 1258516 CrossRef.
- S. Mukherjee,
et al., Reaction kinetics involved in esterification between the fatty acids in castor oil and furfuryl alcohol, Ind. Crops Prod., 2024, 213, 118393 CrossRef CAS.
- K. Taniguchi and M. Karin, NF-κB, inflammation, immunity and cancer: coming of age, Nat. Rev. Immunol., 2018, 18(5), 309–324 CrossRef CAS PubMed.
- T. C. Theoharides, A. Twahir and D. Kempuraj, Mast cells in the autonomic nervous system and potential role in disorders with dysautonomia and neuroinflammation, Ann. Allergy, Asthma, Immunol., 2023, 132(4), 440–454 CrossRef PubMed.
- D. W. Doo,
et al., Inhibition of the Wnt/β-catenin pathway enhances antitumor immunity in ovarian cancer, Ther. Adv. Med. Oncol., 2020, 12, 1758835920913798 CAS.
- T. Mamuladze and J. Kipnis, Type 2 immunity in the brain and brain borders, Cell. Mol. Immunol., 2023, 20(11), 1290–1299 CrossRef CAS PubMed.
- H. Ledford, US cancer institute to overhaul tumour cell lines, Nat. News, 2016, 530(7591), 391 CrossRef.
- A. I. Minchinton and I. F. Tannock, Drug penetration in solid tumours, Nat. Rev. Cancer, 2006, 6(8), 583–592 CrossRef CAS.
- T. P. Padera,
et al., Cancer cells compress intratumour vessels, Nature, 2004, 427(6976), 695 CrossRef CAS.
- J. Denekamp and B. Hobson, Endothelial-cell proliferation in experimental tumours, Br. J. Cancer, 1982, 46(5), 711–720 CrossRef CAS.
- O. Felfoul,
et al., Magneto-aerotactic bacteria deliver drug-containing nanoliposomes to tumour hypoxic regions, Nat. Nanotechnol., 2016, 11(11), 941–947 CrossRef CAS PubMed.
- J. Sinclair,
et al., Profiling signatures of ovarian cancer tumour suppression using 2D-DIGE and 2D-LC-MS/MS with tandem mass tagging, J. Proteomics, 2011, 74(4), 451–465 CrossRef CAS PubMed.
- C. Li,
et al., Effective control of DBPs formation and membrane fouling in catalytic ozonation membrane reactor for municipal wastewater reclamation, Sep. Purif. Technol., 2024, 330, 125492 CrossRef CAS.
- M. K. Jones, A. Nair and M. Gupta, Mast Cells in Neurodegenerative Disease, Front. Cell. Neurosci., 2019, 13, 171 CrossRef CAS.
- J. Niu,
et al., Construction of micro-nano robots: living cells and functionalized biological cell membranes, Front. Bioeng. Biotechnol., 2023, 11, 1277964 CrossRef.
- L. E. Pedersen,
et al., Porcine major histocompatibility complex (MHC) class I molecules and analysis of their peptide-binding specificities, Immunogenetics, 2011, 63, 821–834 CrossRef CAS.
- A. Schmidt, N. Oberle and P. H. Krammer, Molecular mechanisms of treg-mediated T cell suppression, Front. Immunol., 2012, 3, 51 CAS.
- G. Hoxhaj and B. D. Manning, The PI3K–AKT network at the interface of oncogenic signalling and cancer metabolism, Nat. Rev. Cancer, 2020, 20(2), 74–88 CrossRef CAS PubMed.
- D. Miricescu,
et al., PI3K/AKT/mTOR signalling pathway involvement in renal cell carcinoma pathogenesis, Exp. Ther. Med., 2021, 21(5), 1–7 Search PubMed.
- Q. Hu,
et al., JAK/STAT pathway: Extracellular signals, diseases, immunity, and therapeutic regimens, Front. Bioeng. Biotechnol., 2023, 11, 1110765 CrossRef PubMed.
- K. L. Owen, N. K. Brockwell and B. S. Parker, JAK-STAT signaling: a double-edged sword of immune regulation and cancer progression, Cancers, 2019, 11(12), 2002 CrossRef CAS PubMed.
- I. F. Tannock and S. Hayashi, The proliferation of capillary endothelial cells, Cancer Res., 1972, 32(1), 77–82 CAS.
- L. Tesfay,
et al., Stearoyl-CoA desaturase 1 protects ovarian cancer cells from ferroptotic cell death, Cancer Res., 2019, 79(20), 5355–5366 CrossRef CAS.
- M. Hoop,
et al., A smart multifunctional drug delivery nanoplatform for targeting cancer cells, Nanoscale, 2016, 8(25), 12723–12728 RSC.
- S. Aust,
et al., Absence of PD-L1 on tumor cells is associated with reduced MHC I expression and PD-L1 expression increases in recurrent serous ovarian cancer, Sci. Rep., 2017, 7(1), 42929 CrossRef.
- H. Fleury,
et al., Exploiting interconnected synthetic lethal interactions between PARP inhibition and cancer cell reversible senescence, Nat. Commun., 2019, 10(1), 2556 CrossRef PubMed.
|
This journal is © The Royal Society of Chemistry 2024 |
Click here to see how this site uses Cookies. View our privacy policy here.