DOI:
10.1039/D2RA05832D
(Review Article)
RSC Adv., 2022,
12, 32197-32209
Recent advances of magnetite nanomaterials to remove arsenic from water
Received
15th September 2022
, Accepted 27th October 2022
First published on 9th November 2022
Abstract
Pure water is one of the major requirements for living beings but water bodies are contaminated with toxic pollutants and heavy metals. Around 225–500 million people on the earth depend on groundwater, which is highly contaminated by arsenic. Arsenic impurities are present in water as arsenite As(III) and arsenate As(V). Arsenic is a highly toxic metalloid ranking one in toxicity. Researchers have been exploring new techniques and methods to purify water. Magnetic nanoparticles have high absorption and reaction capabilities due to their high surface-to-volume ratio and quantum size effects. Due to their high magnetization, adsorption behaviour, and biodegradability, magnetite nanomaterials are considered excellent materials to purify water. These nanomaterials and their composites are cost-effective as well as they can be easily separated, regenerated, and reused. This review gives a recent overview of the potential of magnetite nanoparticles and their composites to treat contaminated water and remove unwanted arsenic impurities.
1 Introduction
1.1 Contamination of water
Despite more than 70% of the earth being covered with water, available safe drinking water is only 0.3%. Growth in population, rise of industries, and deforestation are the major reasons for clean water scarcity and water contamination. There is a water crisis in the world, and people in many parts of the world do not even get clean water for drinking. This situation would become more complicated in the future due to the increase in the population if the necessary measures are not taken.1,2 This contaminated water is dangerous for humans, living organisms, and sea creatures. Heavy metals, pharmaceuticals, dyes, pesticides, fertilizers, radioactive elements, etc., could contaminate water to a large extent.3 Metalloids and heavy metals such as As, Hg, Cd, Cr, and Pb are very dangerous due to their toxicity and non-biodegradability and can cause serious effects on the cardiovascular, gastrointestinal, renal, and nervous systems.4–6 Out of these metal ions, arsenic has the maximum unfavourable effects on the health of humans because of being more accessible in groundwater than any other metal.7,8
1.2 Arsenic contamination and its origins
Around 40% of the population of the earth depends on groundwater but groundwater is highly contaminated by arsenic. Arsenic is a highly toxic metal ranking one in toxicity and the 12th most common element that exists on the earth. It is present at low levels in rocks and sediments around the world.9 Its presence in water depends on biological activity, weathering reactions, volcanic emissions, and anthropogenic sources. Arsenic originates artificially from oil refineries, chemicals from agriculture, ceramic industries, mineral waste, etc., whereas naturally, it originates from the formation of geological minerals and deposits as depicted in Fig. 1.10 Arsenic impurities are present in water as arsenite As(III) and arsenate As(V), whereas As(III) is exceedingly toxic compared to As(V).11
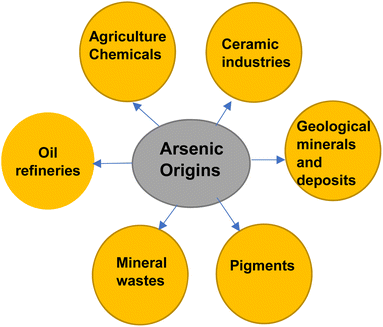 |
| Fig. 1 Origin of arsenic contamination in water. | |
1.3 Effects of arsenic on plants, humans, and animals
Arsenic can contaminate water to a great extent. Excess arsenic concentration in drinking water can lead to skin, cardiovascular, neurological, and respiratory diseases. Its exposure can also cause skin, lung, liver, bladder, and kidney cancer.12–14 Not only humans, arsenic contamination in water affects animals and plants to a great extent. The main effects of arsenic on plants, humans, and animals are depicted in Fig. 2.15 Arsenic is mainly found as As(V) and As(III). Surface water has As(V), whereas groundwater has As(III) in the major form; the United States Environmental Protection Agency and the World Health Organization (WHO) have adopted a maximum contamination level of arsenic of 10 μg L−1 in drinking water.16
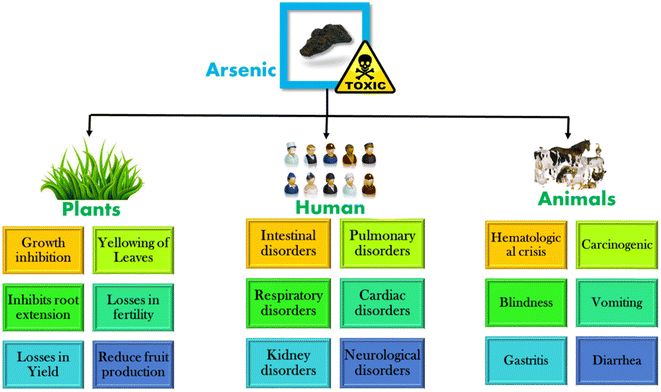 |
| Fig. 2 Effects of arsenic on plants, humans, and animals. Reproduced from ref. 15 with permission from Elsevier, copyright 2021. | |
1.4 Techniques to remove arsenic from wastewater
People have used various traditional methods to clean water from ancient times. These treatment methods consist of boiling, cloth filtration, storing water in copper vessels, storage for an extended time, solar radiation, etc. However, these methods are unsuccessful in the treatment of dissolved pollutants.17 Different technologies such as ion exchange,18,19 co-precipitation,20 reverse osmosis,21 coal combustion,22 green methods,23,24 electrocoagulation,25 electrodeionization,26 and membrane filtration27 are used to remove arsenic from water but these techniques have lower removal efficiencies, produce large amounts of waste, are expensive and produce iron residues.28 Adsorption is an effective technique to clean water and remove unwanted impurities from water.29 Adsorption processes are broadly used to clean groundwater and wastewater for drinking and industrial purposes. This method has many advantages for water purification, such as cost-effectiveness, ease of use, flexibility, adaptable design, less energy consumption, etc.30 However, the viability of adsorption processes highly depends on the type of adsorbent, liquid, pollutants, working conditions, process, regeneration, and disposal of waste.31 The removal efficiency of the adsorbent highly depends on contact time, pH, adsorbent dosage, and concentration of impurities.32
1.5 Nanomaterials for removing arsenic from wastewater
Recently, nanoscale materials are feasible and chemically and ecologically stable having enormously high removal efficiencies and faster kinetics.33 The properties of the nanoparticles strongly depend on the size of the nanoparticles. It has been reported that nanoparticles having sizes less than 30 nm have a large surface-to-volume ratio and superparamagnetic properties.34 These nanoparticles lose their magnetization after the removal of the magnetic field, therefore ideal materials for novel separation processes.35 Nanoparticles have high absorption and reaction capabilities due to their high surface-to-volume ratio and quantum size effects.36 When the surface area is larger, the reaction would be faster as a large surface area makes more sites available for the reaction for a similar volume, leading to more chemical reactivity.37 The large surface area of nanoparticles and quantum size effects enhance the reactivity of nanoparticles with contaminants and give a high reaction rate for absorption and separation of pollutants from water.38
1.6 Magnetite nanomaterials for water treatment
Magnetic nanomaterials are widely used in water treatment due to the ease of separation and reusability of these materials.39 Magnetite nanoparticles are extensively used in many applications in the fields of magnetics, electronics, biomedical sciences, and as sensors.40–43 Magnetite nanoparticles are used as adsorbents in water treatment as they can be separated easily due to high magnetism. These particles could separate and remove the contaminants with the application of external magnetic fields or by centrifugation and filtration.44 Surface modification of these nanoparticles should be required as bare magnetite nanoparticles oxidize easily and aggregate. Also, ferrite nanoparticles show poor stability; therefore, functionalized magnetite composites are widely used for the separation of metals from water and water purification.45 Therefore, magnetite silica nanocomposites,46 magnetite–graphene nanocomposites,47 chitosan–magnetite nanocomposites,48 magnetite nanoparticle coated sand,49 etc. are widely explored for water purification with enhanced results and cost-effectiveness. In composite nanoparticles, the requirement of large doses gets reduced and the efficiency of functionalized particles enhances.
Many researchers address this worldwide problem of arsenic contamination in various review articles. In these reviews, research focuses on the removal of arsenic contamination using chemically modified natural materials,50 pressure-driven and thermally driven membranes,51 a green process using guava leaf biomass, mango bark, and bagasse52, electrocoagulation treatment,53 nano-composites54 and nanomaterials,55 layered double hydroxide,56 hydrotalcite,57 coal fly ash-based adsorbents,58 etc. In recent years, magnetite nanoparticles and their composites have been widely used for arsenic removal. However, the review of magnetite nanoparticles for the removal of arsenic from water remains unexplored. This review gives a recent overview of the potential of magnetite nanoparticles and their composites to treat contaminated water and remove arsenic from wastewater.
2 Adsorption mechanism
2.1 Adsorption process using magnetite nanomaterials
Adsorption is a type of interaction between the adsorbent and adsorbate. The solute which is to be removed in the adsorption process is called an adsorbate, while the solid on which it is retained is known as an adsorbent.59 Several advantages have been cited regarding the adsorption operation. The adsorption process has many advantages; such as, it requires less energy and is cost-effective as many adsorbents can be restored and reused many times. Adsorption is efficient since it can remove or recover all the adsorbate from the solution, providing a perfect separation.60 Adsorption occurs when the adsorbate in a liquid binds itself to a solid substance's surface. Adsorbents should have a very high internal surface area to ease the adsorption. Arsenic contamination could be removed using magnetite nanocomposites. These nanocomposites are added to contaminated water and arsenic gets diffused on the nanocomposites. With a suitable magnet's help, these nanocomposites and arsenic could be removed. It was found that arsenic can be bounded by magnetite nanoparticles. A suitable magnetic field could remove this arsenic-magnetite waste effectively, thereby purifying water. For practical use as an adsorbent, the number of reuse cycles and the material's regeneration are vital for enhancing the adsorption process and reducing operational costs. This process could be repeated many times to get clean water as shown in Fig. 3.
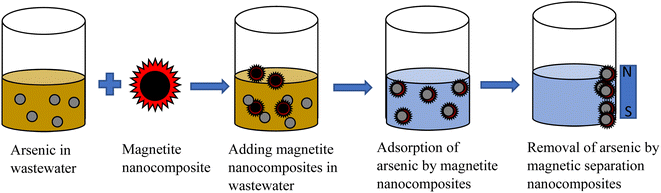 |
| Fig. 3 Process of arsenic removal from wastewater using magnetite nanocomposites. | |
Magnetite can be synthesized either by merging atoms/molecules/clusters or breaking down the bulk material into smaller dimensions. These methods are known as the ‘bottom up’ and ‘top down’ approaches, respectively,61–64 as shown in Fig. 4.
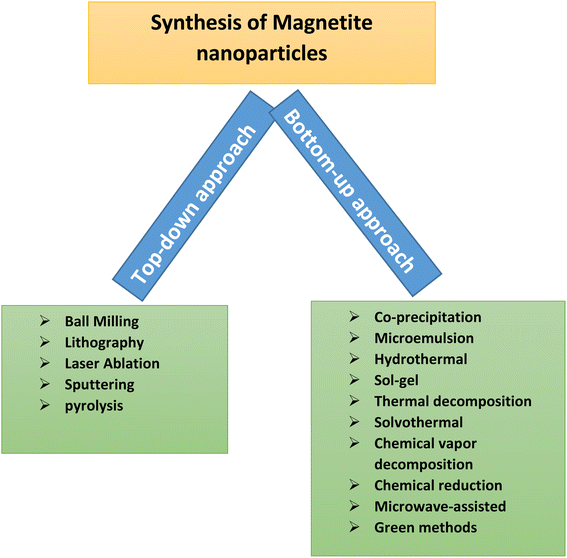 |
| Fig. 4 Techniques to synthesize magnetite nanoparticles. | |
Functionalized magnetite composites having a high surface area have been used for such applications. Then their structure, microstructure and magnetic properties were studied performing different techniques including XRD, SEM/EDX, IR, TGA/DSC, TEM, and SQUID magnetometry.
2.2 Adsorption models
The amount of material adsorbed can be calculated using the relation65 |
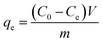 | (1) |
where C0 and Ce are the initial and final concentrations of arsenic in solution (mg L−1), V is the volume of solution (L) and m is the mass of the adsorbent (magnetite composite) (g).
Pseudo-first-order and pseudo-second-order kinetics models are used for estimation of adsorption of arsenic for these experimental data and rate controlling steps are fitted in these models (eqn (2) and (3)).66
|
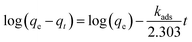 | (2) |
|
 | (3) |
and
where
qe and
qt are the amounts of absorbed arsenic per unit mass of adsorbent at equilibrium and time
t, respectively, in mg g
−1. The
kads and
k are pseudo-first-order and pseudo-second-order adsorption rate constants, and
h is the initial adsorption rate (mg g
−1 min
−1).
Freundlich and Langmuir isotherm models can be used to compare experimental adsorption isotherm data. Eqn (5) represents the linear form of the Freundlich isotherm model (Freundlich 1906):67
|
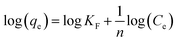 | (5) |
Ce is the equilibrium concentration of As(V) in mg L−1, KF is the adsorption capacity indicator, and n (1 < n < 10) is the adsorption intensity. Adsorption is considered good if n = 1–2, better if n = 2–10 and unfavourable if n < 1.
The Langmuir isotherm model is given by eqn (6) in the linear form as68
|
 | (6) |
where
qm is the maximum adsorption capacity, and
b (L mg
−1) is the Langmuir adsorption constant which depends on the force between the solute and adsorbent.
The adsorption efficiency of the process also depends on ‘r’ which is a dimensionless quantity calculated by using the relation68
|
 | (7) |
where
C0 is the initial concentration of As(
V) and
b is the Langmuir constant.
r < 1.0 indicates favorable adsorption, while
r > 1.0 shows unfavourable adsorption.
3 Arsenic removal using magnetite nano adsorbents
In recent years, iron oxide nanoparticles have exhibited excellent properties in sorption activities due to their high specific surface area, porosity structure, and strong magnetic response, resulting in an exceptional sorption capacity.69,70
Magnetic nanomaterials are promising adsorbents because of the directional movement of these nanomaterials in the applied magnetic field. These nanomaterials are widely used to treat wastewater by removing heavy metals due to their exceptional physical and chemical properties such as strong adsorption, superparamagnetic nature, high surface area, biodegradability, non-toxicity, etc.71,72
3.1 Magnetite nanomaterials to remove heavy metals and dyes from wastewater
Today's Fe3O4 nanomaterials are the most preferred magnetic materials for various applications due to their high magnetization, superparamagnetic nature, low Curie temperature, non-toxicity, etc. These nanomaterials have shown excellent suitability for water treatment because of their stability, low cost, and regeneration after use.73,74
Carboxymethyl cellulose immobilized Fe3O4 nanoparticles were able to adsorb Pb(II) from wastewater.75 The L-arginine modified magnetic bio adsorbent (Fe3O4-CS-L) adsorbs Zn2+, Cd2+, and Pb2+ from an aqueous solution by the adsorption method with good recyclability.76 Surfactant modified magnetite nanoparticles effectively adsorb Cd2+, Pb2+ and Zn2+ from wastewater.77 Iron oxide magnetic nanoparticles (MNPs) grafted on a hyperbranched polyglycerol (HPG) polymer were able to adsorb Ni, Cu, and Al successfully from industrial wastewater.78 Magnetite nanoparticles loaded on activated carbon (Fe3O4-MNPs-AC) have shown a high adsorption capacity for dyes from wastewater in a short time.79 Magnetite nanoparticles exhibited remarkable adsorption for Pb2+ and Cr6+. Mechanisms for Pb2+ adsorption by magnetite nanoparticles are shown in Fig. 5.80
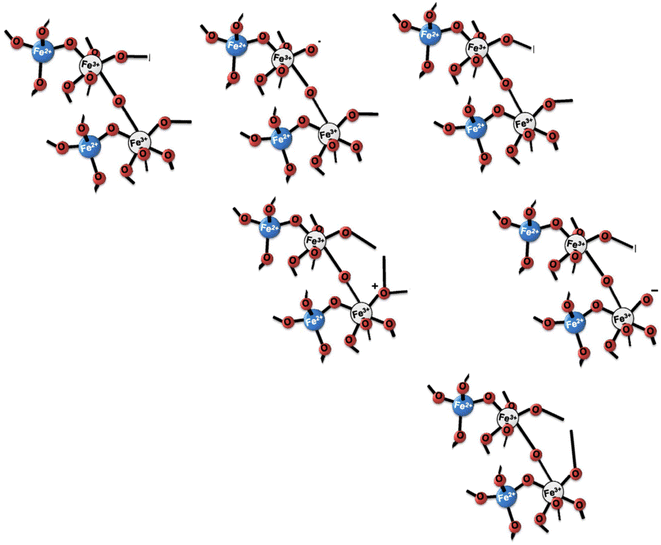 |
| Fig. 5 Lead(II) adsorption on magnetite surfaces. Reproduced from ref. 80 with permission from Elsevier, copyright 2016. | |
L-Cysteine functionalized magnetite nanoparticles have successfully adsorbed Pb2+ and Cr6+ from wastewater and shown good stability and reusability of the adsorbent.81 Fe3O4@SiO2 core/shell magnetic nanoparticles functionalized with hydrous lanthanum oxide easily adsorb phosphate from water.82 Magnetite/carbon composites are synthesized using waste fruit peels. These composites could adsorb methyl blue, Congo red, rhodamine B, and Cr6+ ions for the treatment of wastewater.83 Composites of magnetite nanoparticles, chitosan nanoparticles, and polythiophene are very efficient in adsorbing Hg from wastewater.84 Magnetite graphene oxide encapsulated in alginate beads has shown improved performance to adsorb As(V) and Cr(VI) from aqueous solutions.85 Spent tea-supported magnetite (ST/Mag) nanoparticles successfully removed Cr(VI) from saline wastewater.86
3.2 Magnetite nanocomposites for arsenic removal from wastewater
3.2.1 Magnetite–silica nanocomposite. Porous silica has been used as a carrier vehicle due to the high surface area of these particles which could have applications such as drug delivery agents, catalysis, clean water, etc. The high surface area led to the adsorption of unwanted ions rather than bare particles. The adsorption of arsenic increases with increasing its concentrations for both magnetite and magnetite–silica nanocomposite but the adsorption capacity of the magnetite–silica nanocomposite is higher than that of plain magnetite nanoparticles.46The removal process of arsenic is divided into fast and slow adsorption process rates. It took 30 initial minutes to adsorb 87% of As(V) using magnetite nanoparticle coated sand as the adsorbent, while in the next 330 min, the removal efficiency increased to 99.84% and As(V) concentration was reduced below 5 μg L−1 from 6700 μg L−1. It was observed that magnetite nanoparticle coated sand could adsorb As(V) more effectively in the acidic pH range (2–7) rather than the basic pH range (7–12). As(V) removal efficiency decreased from 99.99 to 39.33% with increasing pH from 7 to 12.87
3.2.2 Magnetite–graphene nanocomposites. Graphene-based materials were observed to be good adsorbents.88 Due to the cost-ineffectiveness of these particles, these materials could not be used in the treatment of wastewater. However, magnetite–graphene nanocomposites have shown outstanding adsorbing properties and have the potential to be used as a better absorbent to clean water effectively.89 pH values play an important role in adsorption. The change in pH could alter the surface charge which subsequently changes the active sites present on the adsorbent material. Researchers found that arsenic removal greatly depends on the pH, ions employed, the size and surface area of nanoparticles, etc.90Non-oxidative graphene (M-nOG) synthesized using a physical exfoliation method showed higher efficiency for arsenic removal. The adsorption of As(V) was maximum at a pH of 4 and decreased sharply with increasing the pH, whereas for As(III), the adsorption capacity was maximum at a pH of 7 as shown in Fig. 6. The adsorption of As(V) on MnGO decreases with increasing the pH due to an increase of the negatively charged surface sites (OH−) on the adsorbent. These negative charges on the surface of the adsorbent increase, resulting in an increase in repulsion between M-nOG and arsenic.91 As(III) and As(V) removal is maximum at a temperature of 35 °C as shown in Fig. 6. The decrease in arsenic removal efficiency indicates the exothermic nature of the adsorption process for temperatures above 35 °C. Maximum adsorption capacities observed for As(III) and As(V) were 32.7 mg g−1 and 13.1 mg g−1, respectively. It was observed that the M-nOG could be regenerated even after being reused for five cycles.16
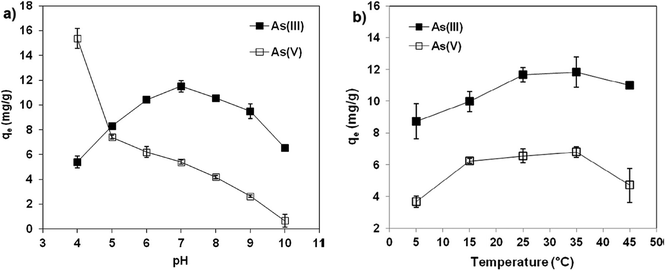 |
| Fig. 6 Effect of (a) pH and (b) temperature on As(III) and As(V) adsorption by M-nOG. Reproduced from ref. 16 with permission from Elsevier, copyright 2017. | |
It was observed that the adsorption capacity of the magnetite–graphene oxide composite for As(III) and As(V) was 85 mg g−1 and 38 mg g−1, whereas that for the magnetite-reduced graphene oxide composite was 57 mg g−1 and 12 mg g−1 for As(III) and As(V). These results showed that an increased amount of magnetite caused significantly higher absorption of arsenic more effectively.92
3.2.3 Magnetic Fe3O4/douglas fir biochar composites. Biochar is a carbonous stable solid resulting from the thermal disintegration of biomass under a low-oxygen atmosphere and could be used as an adsorbent due to its high porosity, surface area, and functionality.93 Magnetic biochar can separate heavy metals from contaminated water easily.94 Magnetic Fe3O4/douglas fir biochar composites (MBC) were used to convert As(III) into less toxic As(V) via redox reactions. Maximum removal percentages depend on the initial concentrations of As(III). A decrease in removal percentage was found with an increase in initial concentrations of As. Experimental data were fitted in accordance with the Sips, Langmuir, and Freundlich models. For low arsenic concentrations, the Sips model reduces to the Freundlich isotherm, whereas for high concentrations, it follows the character of the Langmuir isotherm. Adsorption vs. time for initial As(III) concentrations and Sips, Langmuir, and Freundlich curves are shown in Fig. 7.95
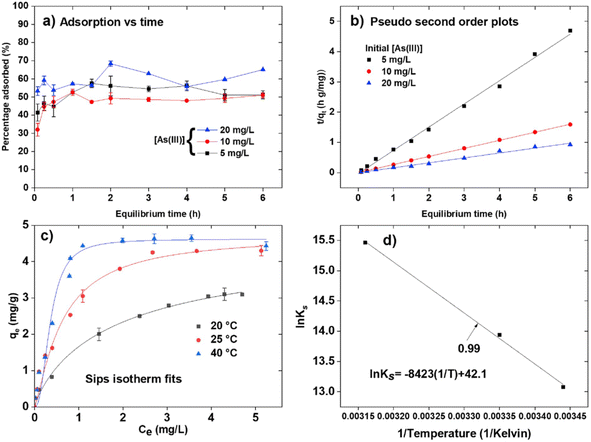 |
| Fig. 7 (a) Adsorption vs. time; (b) pseudo 2nd order model; plots of initial As(III) concentrations of 5, 10, and 20 mg L−1; (c) the Sips isotherm fits of As(III) adsorption; and (d) van't Hoff plot of ln Ks vs. 1/T for the As(III) adsorption. Reproduced from ref. 95 with permission from Elsevier, copyright 2019. | |
3.2.4 Magnetite nanocomposites using organic materials. Superparamagnetic iron oxide nanoparticles were synthesized using tea residue (MION-tea). The adsorption data obeyed the Langmuir equation with a high adsorption capacity of 188.69 mg g−1 for arsenic(III), and 153.8 mg g−1 for arsenic(V). The maximum removal (56.4% to 98.4%) of As(III) occurs with an increase in adsorbent dose from 0.01 to 0.25 g L−1, followed by no change in removal percentage due to the starting equilibrium condition. The removal percentage of arsenic highly depends on the initial amount, the concentration of As(III) and the pH of the solution as shown in Fig. 8. The number of active sites on the adsorbent surface is not sufficient to adsorb As(III) ions at higher concentrations but at low concentration, the ratio of surface active sites to total As(II) is large, and hence As(III) ions can interact with the active sites on the adsorbent surface adequately (Table 1).96
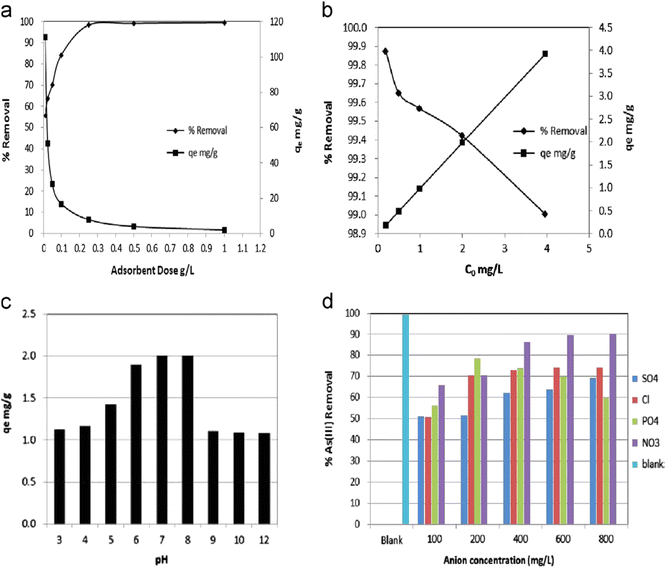 |
| Fig. 8 (a) Effect of adsorbent dose, (b) effect of initial As(III) concentration on As(III) adsorption, (c) effect of pH, and (d) effect of interfering anions on As(III) adsorption, using MION-tea. Reproduced from ref. 96 with permission from Elsevier, copyright 2014. | |
Table 1 Arsenic adsorption behavior of magnetite nanomaterials and their composites
Adsorbent |
Adsorption capacity (Qmax in mg g−1) |
Ref. |
Magnetite/non-oxidative graphene (M-nOG) composites |
32.7 mg g−1 for As(III) |
16 |
13.1 mg g−1 for As(V) |
Magnetite–silica |
170 μmol g−1 |
46 |
Magnetite coated sand |
6695 μg L−1 |
87 |
Magnetite–graphene oxide composites (M-GO) |
85 mg g−1 for As(III) |
92 |
38 mg g−1 for As(V) |
Magnetite-reduced graphene oxide composite (M-rGO) |
57 mg g−1 for As(III) |
12 mg g−1 for As(V) |
MION-tea |
188.69 mg g−1 for arsenic(III) |
96 |
153.8 mg g−1 for arsenic(V) |
Humic acid coated graphene-Fe3O4 nanocomposites |
8.67 mg g−1 for As(III) |
97 |
61.73 mg g−1 for As(V) |
Pine cone magnetite nanoparticles composite |
17 mg g−1 for arsenic(V) |
98 |
Chitosan-coated magnetite nanoparticle |
10.81 mg g−1 for As(V) |
100 |
Magnetite-modified water hyacinth biochar |
7.4 mg g−1 for As(V) |
101 |
Magnetized PSAC |
227.6 mg g−1 for arsenate |
102 |
Magnetite/Cu2+ |
10.33 mg g−1 |
103 |
Yttrium-doped magnetite and goethite |
170.48 mg-As per g for As(V) |
104 |
84.22 mg-As per g for As(III) |
|
Fe3O4-functionalized BNNS (BNNS-Fe3O4) nanocomposite |
26.31 mg g−1 for As(V) |
105 |
Boron nitride nanosheets (BNNSs) |
5.30 mg g−1 |
Fly ash by magnetite |
65.78 mg g−1 |
106 |
MNp-OP |
1.86 mg g−1 for As(III) |
107 |
MNp-CS |
2.79 mg g−1 for As(III) |
The humic acid-coated graphene–magnetite nano-composite has shown absorption capacities of 8.67 and 61.73 mg g−1 for As(III) and As(V) respectively.97 A novel magnetic composite consisting of Fenton treated pine cone, agricultural waste, and magnetite nanoparticles was prepared and applied to achieve simultaneous arsenite oxidation and adsorptive removal.98
Magnetite nanoparticles were synthesized by an eco-friendly green method using onion peel (MNp-OP) and corn silk extract (MNp-CS). Onion peel and corn silk extract are good phenolic compounds to reduce Fe3+. The maximal adsorption capacities for MNp-OP and MNp-S were 1.86 mg g−1 and 2.79 mg g−1, respectively.99 The chitosan/magnetite nanocomposite decreased the amount of As(V) from 0.6 mg L−1 to less than 0.01 mg L−1 and could remove As(V) even after several cycle runs effectively.100 Magnetite-modified water hyacinth biochar has a sorption capacity of 7.4 mg g−1 for As(V) and the used sorbent could be separated using a suitable magnet.101 Palm shell waste-based activated carbon (PSAC) magnetized by hydrothermal impregnation of nano-magnetite and then coated by lanthanum effectively removed arsenate with an adsorption capacity of 227.6 mg g−1.102
3.2.5 Other magnetite nanocomposites. Magnetite nanoparticles synthesized using iron ore tailings were used to adsorb arsenate in the presence of Cu2+, Zn2+, and Mn2+ at pH = 5.5. It was observed that the adsorption of arsenate was more noticeably affected by the copper, followed by zinc and manganese ions. The maximum amount of adsorbed arsenate was 10.33 mg g−1.103 Yttrium-doped iron oxide (magnetite and goethite) adsorbed arsenic(III and V) via the linkages of Y–O–As and Fe–O–As. The adsorption capacities are 170.48 mg-As per g and 84.22 mg-As per g for As(V) and As(III) respectively.104 Boron nitride nanosheets (BNNSs) and Fe3O4-functionalized BNNS (BNNS-Fe3O4) nanocomposite were used for the removal of As(V) ions. The highest adsorption capacity (26.31 mg g−1) was found for the BNNS-Fe3O4 nanocomposite, whereas the bare BNNS adsorbent had 5.30 mg g−1 at pH = 2.105 The fly ash (FA) modified by magnetite (M) showed an adsorption capacity of 19.14 mg g−1 for As(V). After five consecutive adsorption processes, the adsorption capacity increased to 65.78 mg g−1 by FAM.106 Magnetite-rich particles (MEP) were isolated from mill scale (wustite, hematite, magnetite, elemental iron, small amount of oil, and grease) and showed a maximum adsorption quantity of 12.69 mg g−1 of arsenate on 1 g of MEP.107
4 Mechanism of arsenic removal by magnetite
The mechanism of arsenic removal by magnetite was explained by many researchers.108,109 An inner-sphere ligand exchange mechanism is employed to adsorb As(III) and As(V) on magnetite in the form of arsenic oxyanion which exchanges with surface OH or OH2 groups with Fe3+ at the iron oxide surface.110 There are two types of H atoms, i.e., in the inner-sphere complexes and directly attached to the surface which could form the inner-sphere arsenate complexes and FeOH⋯H hydrogen bond.111 The absorbed As(V) anions are generally coordinated to two adjacent Fe3+ cations on the magnetite surface and form a surface complex known as a bidentate binuclear-bridging complex [Fig. 9(a)]. However, iron oxide adsorbs As(III) via both bidentate binuclear-bridging complexes and monodentate complexes. In the monodentate complex, a single oxygen atom from the As(III) oxyanion is coordinated to Fe3+ cations on the magnetite surface [Fig. 9(b)]. The main bonding mechanism of As(III) could include either a monodentate bond or the formation of an outer-sphere complex. In the outer sphere complex, the ligand is bound to the surface OH or OH2, possibly by a hydrogen bond [Fig. 9(c)].92
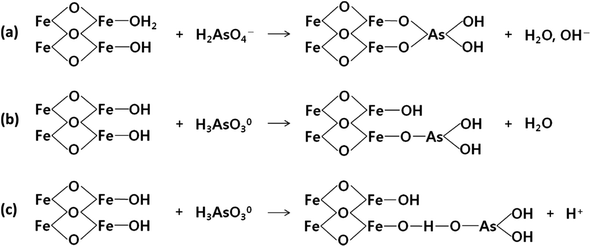 |
| Fig. 9 The mechanisms of arsenic adsorption (a) As(V) and (b), (c) As(III) by magnetite nanocomposites. Reproduced from ref. 92 with permission from Elsevier, copyright 2016. | |
5 Future outlook and challenges
Clean water is an essential requirement of all living beings. There is an urgent need of the hour to find economically feasible, recyclable, and eco-friendly adsorbents for water treatment. Arsenic is the most hazardous contaminant in water. Iron-based nanomaterials could be the future of water purification due to their low cost and convenient technologies. This review reveals the importance of magnetite nanomaterials and their composites to treat water efficiently by removing arsenic impurities from the contaminated water. These materials are ideal due to their high surface-to-volume ratio, control of morphology, easy magnetic separation, reusability, and high efficiency. Magnetite nanomaterials could separate out the contaminated ions or particles via magnetic separation methods which can reduce the cost as compared to traditional methods such as centrifugation, filtration, sedimentation, etc.
Adsorption is considered the most effective method to remove arsenic contamination. Practically, there could be a challenge with agglomeration in magnetite nanomaterials due to their high magnetization. However, these challenges could be tackled by using suitable composites of these materials. There should be a focus on research to find the toxicity and likely health effects. These materials should be studied properly for any hazards to the health and environment. Future research should be based on removing arsenic as well as other toxic impurities from wastewater. It should be kept in mind that these removal techniques keep good and essential minerals in the water intact.
Magnetite and its nanocomposites should be explored for removing different heavy metals such as Hg, Cd, Cr, Pb, etc., and other pollutants from wastewater. These water cleaning techniques should be commercialized in near future. It could be done as a multidisciplinary study for chemists, engineers, material scientists, and biologists worldwide. These materials have shown reusability which could be cost-effective as well. It is our duty to save clean water for our future generations.
6 Conclusion
Arsenic is a highly toxic metal ranking one in toxicity. Efforts have been made to remove this harmful metal from water but could not be successful due to various pros and cons. Researchers have been exploring new techniques and methods to purify water. Magnetite nanomaterials and their composites are considered excellent materials to purify water because of their high magnetization, adsorption behaviour, and biodegradability. These nanomaterials and their composites are cost-effective as well as they can be easily separated, regenerated, and reused. This review gives an overview of the potential of magnetite nanoparticles and their composites to treat contaminated water and remove unwanted arsenic impurities. These materials could be explored to clean water from harmful contamination.
Conflicts of interest
The authors declared that they have no conflicts of interest related to this work. I declare that I do not have any commercial or associative interest that represents a conflict.
Acknowledgements
The author declares that no funds, grants, or other support were received during the preparation of this manuscript.
References
- A. Boretti and L. Rosa, Reassessing the projections of the world water development report, npj Clean Water, 2019, 2, 1–6 CrossRef.
- H. N. Tran and S.-J. You, et al., Mistakes and inconsistencies regarding adsorption of contaminants from aqueous solutions: a critical review, Water Res., 2017, 120, 88–116 CrossRef CAS PubMed.
- S. Sharma and A. Bhattacharya, Drinking water contamination and treatment techniques, Appl. Water Sci., 2017, 7, 1043–1067 CrossRef CAS.
- Z. Fu and X. Shuhua, The effects of heavy metals on human metabolism, Toxicol. Mech. Methods, 2019, 167–176 Search PubMed.
- M. L. Sall, A. K. D. Diaw and D. Gningue-Sall, et al., Toxic heavy metals: impact on the environment and human health, and treatment with conducting organic polymers, a review, Environ. Sci. Pollut. Res., 2020, 27, 29927–29942 CrossRef CAS PubMed.
- S. Tiwari and T. N. Dhole, Assessment of enteroviruses from sewage water and clinical samples during eradication phase of polio in North India, Virol. J., 2018, 15, 1–8 CrossRef PubMed.
- M. E. Shaji, K. V. Santosh and P. P. Sarath, et al., Arsenic contamination of groundwater: a global synopsis with focus on the Indian Peninsula, Geosci. Front., 2021, 12, 101079 CrossRef.
- G. Abbas, B. Murtaza and I. Bibi, et al., Arsenic uptake, toxicity, detoxification, and speciation in plants: physiological, biochemical, and molecular aspects, Int. J. Environ. Res. Public Health, 2018, 15, 59 CrossRef PubMed.
- A. J. A. Bobb, S. A. Fathi and S. Moussa, et al., Laser synthesis of magnetite-partially reduced graphene oxide nanocomposites for arsenate removal from water, J. Mater. Sci., 2020, 55, 5351–5363 CrossRef.
- S. Lata and S. R. Samadder, Removal of arsenic from water using nano adsorbents and challenges: a review, J. Environ. Manage., 2016, 166, 387–406 CrossRef CAS PubMed.
- J. Guo, S. Ci and P. Cai, et al., Loading NiCo alloy nanoparticles onto nanocarbon for electrocatalytic conversion of arsenite into arsenate, Electrochem. Commun., 2019, 104, 106477 CrossRef CAS.
- V. R. Moreira, Y. A. Lebron and L. V. Santos, et al., Arsenic contamination, effects, and remediation techniques: a special look onto membrane separation processes, Process Saf. Environ. Prot., 2021, 148, 604–623 CrossRef CAS.
- E. C. Monteiro De Oliveira, E. S. Caixeta and V. S. Santos, et al., Arsenic exposure from groundwater: environmental contamination, human health effects, and sustainable solutions, J. Toxicol. Environ. Health, Part B, 2021, 24, 119–135 CAS.
- A. Chikkanna, L. Mehan, P. K. Sarath, et al., Arsenic exposures, poisoning, and threat to human health: arsenic affecting human health, in Environmental exposures and human health challenges, IGI Glob, 2019, pp. 86–105 Search PubMed.
- B. Senthil Rathi and P. Senthil Kumar, A review on sources, identification and treatment strategies for the removal of toxic Arsenic from the water system, J. Hazard. Mater., 2021, 418, 126299 CrossRef PubMed.
- Y. Yoon, et al., Synthesis of magnetite/non-oxidative graphene composites and their application for arsenic removal, Sep. Purif. Technol., 2017, 178, 40–48 CrossRef CAS.
- B. Thomas, C. Vinka and L. Pawan, et al., Sustainable groundwater treatment technologies for underserved rural communities in emerging economies, Sci. Total Environ., 2022, 813, 152633 CrossRef CAS PubMed.
- B. S. Rathi, P. S. Kumar and R. Ponprasath, et al., An effective separation of toxic arsenic from Aquatic environment using electrochemical ion exchange process, J. Hazard. Mater., 2021, 412, 125240 CrossRef CAS PubMed.
- M. Pessoa Lopes, C. F. Galinha and J. G. Crespo, et al., Optimisation of arsenate removal from water by an integrated ion-exchange membrane process coupled with Fe co-precipitation, Sep. Purif. Technol., 2020, 246, 116894 CrossRef CAS.
- T. Nur, P. Loganathan and M. B. Ahmed, et al., Removing arsenic from water by coprecipitation with iron: Effect of arsenic and iron concentrations and adsorbent incorporation, Chemosphere, 2019, 226, 431–438 CrossRef CAS PubMed.
- H. Elcik, S. O. Celik and M. Cakmakci, et al., Performance of nanofiltration and reverse osmosis membranes for arsenic removal from drinking water, Desalin. Water Treat., 2016, 57, 20422–20429 CrossRef CAS.
- Y. Wang, J. Yu and Z. Wang, et al., A review on arsenic removal from coal combustion: advances, challenges and opportunities, Chem. Eng. J., 2021, 414, 128785 CrossRef CAS.
- N. K. Niazi, I. Bibi and M. Shahid, et al., Arsenic removal by perilla leaf biochar in aqueous solutions and groundwater: an integrated spectroscopic and microscopic examination, Environ. Pollut., 2018, 232, 31–41 CrossRef CAS PubMed.
- S. Pathan and S. Bose, Arsenic Removal Using “Green” Renewable Feedstock-Based Hydrogels: Current and Future Perspectives, ACS Omega, 2018, 3, 5910–5917 CrossRef CAS PubMed.
- P. V. Nidheesh and T. A. Singh, Arsenic removal by electrocoagulation process: recent trends and removal mechanism, Chemosphere, 2017, 181, 418–432 CrossRef CAS PubMed.
- D. Lee, J. Y. Lee and Y. Kim, et al., Investigation of the performance determinants in the treatment of arsenic-contaminated water by continuous electrodeionization, Sep. Purif. Technol., 2017, 179, 381–392 CrossRef CAS.
- L. Hao, N. Wang and C. Wang, et al., Arsenic removal from w.ater and river water by the combined adsorption – UF membrane process, Chemosphere, 2018, 202, 768–776 CrossRef CAS PubMed.
- D. Mohanty, Conventional as well as emerging arsenic removal technologies—a critical review, Water, Air, Soil Pollut., 2017, 228, 1–21 CrossRef CAS.
- B. Liu, K. H. Kim and V. Kumar, et al., A review of functional sorbents for adsorptive removal of arsenic ions in aqueous systems, J. Hazard. Mater., 2020, 388, 121815 CrossRef CAS PubMed.
- L. Hao, M. Liu and N. Wang, et al., A critical review on arsenic removal from water using iron-based adsorbents, RSC Adv., 2018, 8, 39545–39560 RSC.
- S. Rahdar, M. Taghavi and R. Khaksefdi, et al., Adsorption of arsenic (V) from aqueous solution using modified saxaul ash: isotherm and thermodynamic study, Appl. Water Sci., 2019, 9, 87 CrossRef.
- S. Ashraf, A. Siddiqa and S. Shahida, et al., Titanium-based nanocomposite materials for arsenic removal from water: a review, Heliyon, 2019, 5, e01577 CrossRef PubMed.
- J. Nikic, J. M. Jazic and M. Watson, et al., Application of nanomaterials in water treatment: arsenic and natural organic matter removal, Recent Pat. Nanotechnol., 2021, 15, 197–224 CrossRef CAS PubMed.
- S. Chaturvedi and P. N. Dave, Water purification using nanotechnology an emerging opportunities, Chem. Methodol., 2019, 3, 115–144 Search PubMed.
- S. Lata and S. R. Samadder, Removal of arsenic from water using nano adsorbents and challenges: a review, J. Environ. Manage., 2016, 166, 387–406 CrossRef CAS PubMed.
- M. Anjum, R. Miandad and M. Waqas, et al., Remediation of wastewater using various nano-materials, Arabian J. Chem., 2019, 12, 4897–4919 CrossRef CAS.
- P. D. Gupta, A. Muthukumar and V. Shilpa, et al., Nanotechnology in drinking water Purification: a critical review, J. Cell Tissue Res., 2017, 17, 6315–6321 CAS.
- S. Mosivand and I. Kazeminezhad, Magnetite nanoparticles functionalized with polypyrrole by pulsed sono-electrocrystallization and their applications for water treatment, J. Mater. Sci.: Mater. Electron., 2018, 29, 12466–12476 CrossRef CAS.
- F. Liu, K. Zhou and Q. Chen, et al., Comparative study on the synthesis of magnetic ferrite adsorbent for the removal of Cd(II) from wastewater, Adsorpt. Sci. Technol., 2018, 36, 1456–1469 CrossRef CAS.
- J. Li, H. Yao and W. Sun, et al., Hyaluronic acid-modified hydrothermally synthesized iron oxide nanoparticles for targeted tumor MR imaging, Biomaterials, 2014, 35, 3666–3677 CrossRef CAS PubMed.
- A. C. C. Arantes, L. Eduardo Silva and D. F. Wood, et al., Bio-based thin films of cellulose nanofibrils and magnetite for potential application in green electronics, Carbohydr. Polym., 2019, 207, 100–107 CrossRef CAS PubMed.
- T. M. Freire, L. M. U. Dutra and D. C. Queiroz, et al., Fast ultrasound assisted synthesis of chitosan-based magnetite nanocomposites as a modified electrode sensor, Carbohydr. Polym., 2016, 151, 760–769 CrossRef CAS PubMed.
- D. Luong, S. Sau and P. Kesharwani, et al., Polyvalent folate-dendrimer-coated iron oxide theranostic nanoparticles for simultaneous magnetic resonance imaging and precise cancer cell targeting, Biomacromolecules, 2017, 18, 1197–1209 CrossRef CAS PubMed.
- E.-G. Nour Sh and N. N. Hussein, Biosynthesized magnetite nanoparticles as an environmental opulence and sustainable wastewater treatment, Sci. Total Environ., 2021, 774, 145610 CrossRef PubMed.
- M. S. Alam, B. Bishop and N. Chen, et al., Reusable magnetite nanoparticles–biochar composites for the efficient removal of chromate from water, Sci. Rep., 2020, 10, 19007 CrossRef CAS PubMed.
- M. Kokate, K. Garadkar and A. Gole, One pot synthesis of magnetite–silica nanocomposites: applications as tags, entrapment matrix and in water purification, J. Mater. Chem. A, 2013, 1, 2022–2029 RSC.
- A. Abu-Nada, G. McKay and A. Abdala, Recent advances in applications of hybrid graphene materials for metals removal from wastewater, Nanomaterials, 2020, 10, 595 CrossRef CAS PubMed.
- E. Cheraghipour and M. Pakshir, Process optimization and modeling of Pb(II) ions adsorption on chitosan-conjugated magnetite nano-biocomposite using response surface methodology, Chemosphere, 2020, 260, 127560 CrossRef CAS PubMed.
- J. Sorwat, M. Adrian and M. Maisch, et al., Chromium (VI) removal kinetics by magnetite-coated sand: small-scale flow-through column experiments, J. Hazard. Mater., 2021, 415, 125648 CrossRef CAS PubMed.
- T. G. Asere, C. V. Stevens and G. Du Laing, Use of (modified) natural adsorbents for arsenic remediation: a review, Sci. Total Environ., 2019, 676, 706–720 CrossRef CAS PubMed.
- A. Criscuoli and A. Figoli, Pressure-driven and thermally-driven membrane operations for the treatment of arsenic-contaminated waters: a comparison, J. Hazard. Mater., 2019, 370, 147–155 CrossRef CAS PubMed.
- D. Mohan, S. Dey and S. B. Dwivedi, et al., Adsorption of arsenic using low cost adsorbents: guava leaf biomass, mango bark and bagasse, Curr. Sci., 2019, 117(4), 00113891 CrossRef.
- P. Song, Z. Yang and G. Zeng, et al., Electrocoagulation treatment of arsenic in wastewaters: a comprehensive review, Chem. Eng. J., 2017, 317, 707–725 CrossRef CAS.
- S. I. Siddiqui and S. A. Chaudhry, Arsenic removal from water using nano-composites: a review, Curr. Environ. Eng., 2017, 4, 81–102 CAS.
- W. W. Wong, H. Y. Wong and A. Borhan, et al., Recent advances in exploitation of nanomaterial for arsenic removal from water: a review, Nanotechnology, 2017, 28, 1–31 CrossRef PubMed.
- J. Wang, T. Zhang and M. Li, et al., Arsenic removal from water/wastewater using layered double hydroxide derived adsorbents, a critical review, RSC Adv., 2018, 8, 22694–22709 RSC.
- A. C. Dias and M. P. Fontes, Arsenic (V) removal from water using hydrotalcites as adsorbents: a critical review, Appl. Clay Sci., 2020, 191, 105615 CrossRef CAS.
- F. O. Ochedi, Y. Liu and A. Hussain, A review on coal fly ash-based adsorbents for mercury and arsenic removal, J. Cleaner Prod., 2020, 267, 122143 CrossRef CAS.
- S. B. Pillai, Adsorption in Water and Used Water Purification, in Handbook of Water and Used Water Purification, ed. J. Lahnsteiner, Springer, Cham, 2020 Search PubMed.
- R. Yousef, H. Qiblawey and M. H. El-Naas, Adsorption as a Process for Produced Water Treatment: a Review, Processes, 2020, 8, 1657 CrossRef CAS.
- S. K. Kulkarni, Nanotechnology: Principles and Practices, Capital Publishing Company, 2nd edn, 2011 Search PubMed.
- H. H. Willard, et al., Instrumental Methods of Analysis, Journal of Chemical Education, 7th edn, 1966 Search PubMed.
- G. L. Hornyak, et al., Introduction to Nanoscience and Nanotechnology, CRC Press, 1st edn, 2009 Search PubMed.
- M. Wilson, et al., Nanotechnology: Basic Science and Emerging Technologies, Chapman and Hall/CRC, 1st edn, 2002 Search PubMed.
- M. Kumar, R. Goswami and A. K. Patel, Scenario, perspectives and mechanism of arsenic and fluoride co-occurrence in the groundwater: a review, Chemosphere, 2020, 249, 126126 CrossRef CAS PubMed.
- B. S. Rathi, P. S. Kumar and P. L. Show, A review on effective removal of emerging contaminants from aquatic systems: current trends and scope for further research, J. Hazard. Mater., 2021, 409, 124413 CrossRef CAS PubMed.
- R. Ezzati, Derivation of Pseudo-First-Order, Pseudo-Second-Order and Modified Pseudo-First-Order rate equations from Langmuir and Freundlich isotherms for adsorption, Chem. Eng. J., 2020, 392, 123705 CrossRef CAS.
- C. S. T. Araújo, I. L. S. Almeida and H. C. Rezende, et al., Elucidation of mechanism involved in adsorption of Pb(II) onto lobeira fruit (Solanum lycocarpum) using Langmuir, Freundlich and Temkin isotherms, Microchem. J., 2018, 137, 348–354 CrossRef.
- F. Liu, K. Zhou and Q. Chen, et al., Comparative study on the synthesis of magnetic ferrite adsorbent for the removal of Cd(II) from wastewater, Adsorpt. Sci. Technol., 2018, 36, 1456–1469 CrossRef CAS.
- Y. Zhang, S. Ni and X. Wang, et al., Ultrafast adsorption of heavy metal ions onto functionalized lignin-based hybrid magnetic nanoparticles, Chem. Eng. J., 2019, 372, 82–91 CrossRef CAS.
- M. I. A. Abdel Maksoud, A. M. Elgarahy and C. Farrell, Insight on water remediation application using magnetic nanomaterials and biosorbents, Coord. Chem. Rev., 2020, 403, 213096 CrossRef.
- R. K. Gautam, P. K. Gautam and S. Banerjee, et al., Removal of Ni(II) by magnetic nanoparticles, J. Mol. Liq., 2015, 204, 60–69 CrossRef CAS.
- U. Yenial, G. Bulut and F. Pagnanelli, Manganese ferrite nanoparticle production from industrial wastes as sorbent material for arsenic removal from aqueous solutions, Part. Sci. Technol., 2020, 38, 433–442 CrossRef CAS.
- K. Peeters, G. Lespes and T. Zuliani, et al., The fate of iron nanoparticles in environmental waters treated with nanoscale zero-valent iron, FeO NPs and Fe3O4 NPs, Water Res., 2016, 94, 315–327 CrossRef CAS PubMed.
- H. Fan and X. Ma, et al., Highly efficient removal of heavy metal ions by carboxymethyl cellulose immobilized Fe3O4 nanoparticles prepared via high-gravity technology, Carbohydr. Polym., 2019, 213, 39–49 CrossRef CAS PubMed.
- S. Guo, P. Jiao and Z. Dan, et al., Synthesis of magnetic bioadsorbent for adsorption of Zn(II), Cd(II) and Pb(II) ions from aqueous solution, Chem. Eng. Res. Des., 2017, 126, 217–231 CrossRef CAS.
- F. I. El-Dib, D. E. Mohamed and A. Omnia, et al., Study the adsorption properties of magnetite nanoparticles in the presence of different synthesized surfactants for heavy metal ions removal, Egypt. J. Pet., 2020, 29, 1–7 CrossRef.
- F. Almomani, R. Bhosale and M. Khraisheh, et al., Heavy metal ions removal from industrial wastewater using magnetic nanoparticles (MNP), Appl. Surf. Sci., 2020, 506, 144924 CrossRef CAS.
- A. R. Bagheri, M. Ghaedi and A. Asfaram, et al., Comparative study on ultrasonic assisted adsorption of dyes from single system onto Fe3O4 magnetite nanoparticles loaded on activated carbon: Experimental design methodology, Ultrason. Sonochem., 2017, 34, 294–304 CrossRef CAS PubMed.
- S. Rajput, C. U. Pittman and D. Mohan, Magnetic magnetite (Fe3O4) nanoparticle synthesis and applications for lead (Pb2+) and chromium (Cr6+) removal from water, J. Colloid Interface Sci., 2016, 468, 334–346 CrossRef CAS PubMed.
- Y. Bagbi, A. Sarswat and D. Mohan, et al., Lead and Chromium Adsorption from Water using L-Cysteine Functionalized Magnetite (Fe3O4) Nanoparticles, Sci. Rep., 2017, 7, 7672 CrossRef PubMed.
- L. Lai, Q. Xie and L. Chi, et al., Adsorption of phosphate from water by easily separable Fe3O4@SiO2 core/shell magnetic nanoparticles functionalized with hydrous lanthanum oxide, J. Colloid Interface Sci., 2016, 465, 76–82 CrossRef CAS PubMed.
- J. Ma, S. Sun and K. Chen, Facile and scalable synthesis of magnetite/carbon adsorbents by recycling discarded fruit peels and their potential usage in water treatment, Bioresour. Technol., 2017, 233, 110–115 CrossRef CAS PubMed.
- R. E. Morsi, A. M. Al-Sabagh and Y. M. Moustafa, et al., Polythiophene modified chitosan/magnetite nanocomposites for heavy metals and selective mercury removal, Egypt. J. Pet., 2018, 27, 1077–1085 CrossRef.
- H. C. Vu, A. D. Dwivedi and T. Thanh Le, et al., Magnetite graphene oxide encapsulated in alginate beads for enhanced adsorption of Cr(VI) and As(V) from aqueous solutions: Role of crosslinking metal cations in pH control, Chem. Eng. J., 2017, 307, 220–229 CrossRef CAS.
- A. A. Babaei, M. Ahmadi and G. Goudarzi, et al., Adsorption of Cr(VI) from saline wastewater using spent tea-supported magnetite nanoparticle, Desalin. Water Treat., 2016, 57, 12244–12256 CrossRef CAS.
- S. Kango and R. Kumar, Magnetite nanoparticles coated sand for arsenic removal from drinking water, Environ. Earth Sci., 2016, 75, 381 CrossRef.
- L. P. Lingamdinne, S. Lee and J. S. Choi, et al., Potential of the magnetic hollow sphere nanocomposite (graphene oxide-gadolinium oxide) for arsenic removal from real field water and antimicrobial applications, J. Hazard. Mater., 2021, 402, 123882 CrossRef CAS PubMed.
- B. Ramalingam, T. Parandhaman and P. Choudhary, et al., Biomaterial Functionalized Graphene-Magnetite Nanocomposite: A Novel Approach for Simultaneous Removal of Anionic Dyes and Heavy-Metal Ions, ACS Sustainable Chem. Eng., 2018, 6, 6328–6341 CrossRef CAS.
- K. S. Padmavathy, G. Madhu and P. V. Haseena, A study on effects of pH, adsorbent dosage, time, initial concentration, and adsorption isotherm study for the removal of hexavalent chromium (Cr (VI)) from wastewater by magnetite nanoparticles, Proc. Technol., 2016, 24, 585–594 CrossRef.
- W. K. Park, Y. Yoon and S. Kim, et al., Feasible water flow filter with facilely functionalized Fe3O4-non-oxidative graphene/CNT composites for arsenic removal, J. Environ. Chem. Eng., 2016, 4, 3246–3252 CrossRef CAS.
- Y. Yoon, W. K. Park and T.-M. Hwang, et al., Comparative evaluation of magnetite–graphene oxide and magnetite-reduced graphene oxide composite for As(III) and As(V) removal, J. Hazard. Mater., 2016, 304, 196–204 CrossRef CAS PubMed.
- A. L. Bursztyn Fuentes, F. Barraqué and R. C. Mercader, et al., Efficient low-cost magnetic composite based on eucalyptus wood biochar for arsenic removal from groundwater, Groundw. Sustain. Dev., 2021, 14, 100585 CrossRef.
- B. Sajjadi, R. M. Shrestha and W.-Y. Chen, et al., Double-layer magnetized/functionalized biochar composite: Role of microporous structure for heavy metal removals, J. Water Process. Eng., 2021, 39, 101677 CrossRef.
- C. M. Navarathna, A. G. Karunanayake and S. R. Gunatilake, et al., Removal of Arsenic(III) from water using magnetite precipitated onto Douglas fir biochar, J. Environ. Manage., 2019, 250, 109429 CrossRef CAS PubMed.
- S. Lunge, S. Singh and A. Sinha, Magnetic iron oxide (Fe3O4) nanoparticles from tea waste for arsenic removal, J. Magn. Magn. Mater., 2014, 356, 21–31 CrossRef CAS.
- B. Paul, V. Parashar and A. Mishra, Graphene in the Fe3O4 nano-composite switching the negative influence of humic acid coating into an enhancing effect in the removal of arsenic from water, Environ. Sci.: Water Res. Technol., 2015, 1, 77–83 RSC.
- I. L. A. Ouma, E. B. Naidoo and A. E. Ofomaja, Thermodynamic, Kinetic and Spectroscopic Investigation of Arsenite Adsorption Mechanism on Pine Cone–Magnetite Composite, J. Environ. Chem. Eng., 2018, 6, 5409–5419 CrossRef CAS.
- J. Nikić, A. Tubić and M. Watson, et al., Arsenic Removal from Water by Green Synthesized Magnetic Nanoparticles, Water, 2019, 11, 2520 CrossRef.
- P. Gogoi, A. J. Thakur and R. R. Devi, et al., Adsorption of As(V) from contaminated water over Chitosan-coated magnetite nanoparticle: Equilibrium and kinetics study, Environ. Nanotechnol., Monit. Manage., 2017, 8, 297–305 Search PubMed.
- F. Zhang, X. Wang and X. Ji, et al., Efficient arsenate removal by magnetite-modified water hyacinth biochar, Environ. Pollut., 2016, 216, 575–583 CrossRef CAS PubMed.
- F. M. Jais, S. Ibrahim and Y. Yoon, et al., Enhanced arsenate removal by lanthanum and nano-magnetite composite incorporated palm shell waste-based activated carbon, Sep. Purif. Technol., 2016, 169, 93–102 CrossRef CAS.
- E. Darezereshki, A. K. Darban and M. Abdollahy, et al., Influence of heavy metals on the adsorption of arsenate by magnetite nanoparticles: Kinetics and thermodynamic, Environ. Nanotechnol., Monit. Manage., 2018, 10, 51–62 Search PubMed.
- Y. Yang, L. Yu and K. Shih, et al., Yttrium-doped iron oxide magnetic adsorbent for enhancement in arsenic removal and ease in separation after applications, J. Colloid Interface Sci., 2018, 521, 252–260 CrossRef PubMed.
- R. S. Bangari, A. K. Singh and S. Namsani, et al., Magnetite-Coated Boron Nitride Nanosheets for the Removal of Arsenic(V) from Water, ACS Appl. Mater. Interfaces, 2019, 11, 19017–19028 CrossRef CAS PubMed.
- M. Karanac, M. Đolić and Z. Veličković, et al., Efficient multistep arsenate removal onto magnetite modified fly ash, J. Environ. Manage., 2018, 224, 263–276 CrossRef CAS PubMed.
- M. Kashif Shahid, S. Phearom and Y.-G. Choi, Adsorption of arsenic (V) on magnetite-enriched particles separated from the mill scale, Environ. Earth Sci., 2019, 78, 65 CrossRef.
- B. C. Bostick, C. Chen and S. Fendorf, Arsenite retention mechanisms within estuarine sediments of Pescadero, CA, Environ. Sci. Technol., 2004, 38, 3299–3304 CrossRef CAS PubMed.
- S. Goldberg and C. T. Johnston, Mechanisms of arsenic adsorption on amorphous oxides evaluated using macroscopic measurements, vibrational spectroscopy, and surface complexation modeling, J. Colloid Interface Sci., 2001, 234, 204–216 CrossRef CAS PubMed.
- M. Shi, X. Min and Y. Ke, et al., Recent progress in understanding the mechanism of heavy metals retention by iron (oxyhydr)oxides, Sci. Total Environ., 2021, 752, 141930 CrossRef CAS PubMed.
- X. Liang, X. Lin and G. Wei, et al., Competitive adsorption geometries for the arsenate As(V) and phosphate P(V) oxyanions on magnetite surfaces: Experiments and theory, Am. Mineral., 2021, 106, 374–388 CrossRef.
|
This journal is © The Royal Society of Chemistry 2022 |