DOI:
10.1039/D2RA05844H
(Paper)
RSC Adv., 2022,
12, 32185-32196
Synthesis, nonlinear optical analysis and DFT studies of D–π–D and A–π–A configured Schiff bases derived from bis-phenylenediamine†
Received
16th September 2022
, Accepted 6th October 2022
First published on 9th November 2022
Abstract
Herein, an integral approach has been made towards the exploration of electronic and structural parameters of four synthesized (DMA with an A–π–A configuration and DMM, DAM, and DMD with a D–π–D configuration) and one designed (DMB-D) novel Schiff base compounds. Bis phenylenediamine derivatives were prepared by condensation of 4,5-dimethyl-o-phenylenediamine (1) with various substituted benzaldehydes (2a–d). The structures of compounds were confirmed by spectroscopic techniques, i.e., UV-visible, FT-IR, and NMR spectroscopy. The DFT-based analysis of entitled compounds was performed via density functional theory utilizing the M06-2X functional in conjugation with the 6-311G(d,p) basis set to acquire geometrical parameters, natural bonding orbital (NBO), the density of states (DOS), non-linear optical (NLO), molecular electrostatic potential (MESP), and natural population analyses. The smallest band gap of (5.446 eV) was noted for DMA via frontier molecular orbital (FMO) analysis. GRPs were obtained with the aid of Egap values as DMA with the lowest band gap displayed a small magnitude of hardness (2.723 eV) and a large magnitude of softness (0.183 eV). The βtot values of DMA, DMM, DMB-D, DAM, and DMD were 56.95, 0.43, 2.53, 8.98, and 68.47 times larger than urea (βtot = 3.71 × 10−31 e.s.u.), respectively. The observed fascinating NLO properties of these novel compounds might be helpful for further advancement in non-linear optics.
Introduction
Schiff bases are synthesized when a primary amine is treated with either a ketone or an aldehyde under certain circumstances. Schiff bases are also known as azomethine or an imine, due to the presence of the HC
N chromophore.1,2 Schiff bases have been discovered in the biological sciences to have antioxidant, antimalarial, antifungal, anticancer, antibacterial, anti-inflammatory, and antiviral properties, as well as catalyzing the reduction of thionyl chloride, polymerization reaction, aldol condensation reaction, reduction of ketones, hydrosilylation of ketones, Henry reaction, epoxidation of alkenes, production of bis(indolyl) methane and Diels Alder reaction.3 A series of metal-based compounds are synthesized by using Schiff bases as ligands because they can form stable complexes with metal ions.4,5 Schiff bases with a solvatochromic UV-vis spectrum (solvatochromicity) can serve as NLO active materials.6 The ligands could be beneficial in solid phase extraction and the development of ion-selective electrodes for determining anions in analytical samples.7
The NLO compounds play an excellent part in electro-optics for signal processing, especially in fiber optics, telecommunications, and information technology.8–10 They have simple chemistry, a low cost of creation, and a tendency to allow structural modifications to permit diverse NLO behaviors. NLO materials formed from the organic networks have become the focus of recent research.11–13 The unique property of NLO materials is the transfer of intramolecular charge transfer (ICT) from electron donating to electron accepting moieties through conjugation links.14–16 Experimental and computational data suggest that introducing strong donor (D) and acceptor (A) parts on paradoxical edges of the spacer, i.e., D–A, D–D–A, and A–D–A, can result in a broad second-order NLO response.13 Charge transfer is improved in compounds containing delocalized electrons in a D–A configuration.17,18 In optoelectronics and photonics, the demand for the production of new NLO materials has expanded dramatically in recent years. The intriguing photo-physical behavior of NLO materials, when exposed to strong laser light, is responsible for their wide range of applicability.19,20 The synthetic organic compounds have a lot of interest in developing fast response rate, higher laser impairment approach, greater photo-electric quantum, a small value of dielectric constant, fabrication adaptability, and an inexpensive development price.
Experimental
Chemistry
All the chemicals used in this research were in extra pure form and purchased from Sigma-Aldrich. A Bruker FT-IR IFS48 spectrophotometer was used to record the FT-IR spectrum (KBr discs). On the melting point device (Büchi 434), melting points were measured. A Bruker Avance 400 spectrometer was used to capture the NMR spectrum in DMSO-d6. The thin layer chromatographs were visualized under the influence of ultraviolet light to check the progress of reaction.
General method for the synthesis of phenylenediamine derivatives. 4,5-Dimethylbenzene-1,2-diamine (1) (5 mmol) was added to a stirred solution of suitable benzaldehyde (2a–d) (5 mmol) in 10 mL of methanol with the catalytic quantity (1–2 drops) of glacial acetic acid. The resulting mixture was allowed to cool at room temperature after being refluxed overnight and continuously monitored by the TLC. The resulting crystalline products (DMA, DMM, DAM and DMD) were filtered, cleaned with hot methanol, and allowed to air dry. The desired products with 75–90% yield were recrystallized from the methanol (Scheme 1).
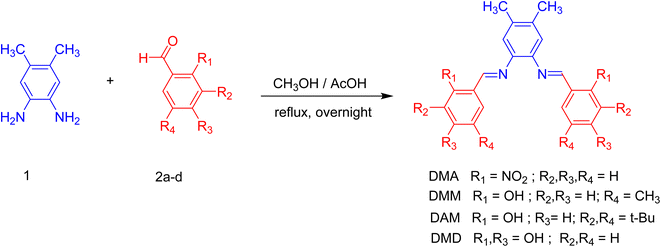 |
| Scheme 1 Synthesis of phenylenediamine derivatives. | |
The targeted compounds are characterized as follows:
(1E,1′E)-N,N'-(4,5-Dimethyl-1,2-phenylene)bis(1-(2-nitrophenyl)methanimine) (DMA). Yield 80%, mp. 156–158 °C; FT-IR (cm−1): 1586 cm−1 (C
N), 3287 cm−1 (N–H), 1450 cm−1 (NO2); 1H NMR (DMSO-d6) δ-ppm; 2.28 (s, 3H, CH3), 7.06 (s, 1H), 7.73 (t, J = 7.6 Hz, 1H), 7.82 (t, J = 7.5 Hz, 1H), 8.05 (d, J = 8.0 Hz, 1H), 8.15 (d, J = 7.7 Hz, 1H), 8.76 (s, 1H, CH
N); 13C-NMR (δ-ppm) 19.2, 122.0, 124.5, 129.7, 130.1, 131.9, 133.6, 135.7, 141.4, 149.3, 156.9; anal. calcd for C22H18 N4O4 (402.13): C = 65.66, H = 4.51, N = 13.92; found (%): C = 65.74, H = 4.36, N = 14.01.
2,2′-((1E,1′E)-((4,5-Dimethyl-1,2-phenylene)bis(azaneylylidene))bis(methaneylylidene))bis(4-methylphenol) (DMM). Yield 78%, mp. 157–159 °C. FT-IR (cm−1): 1586 cm−1 (C
N), 3287 cm−1 (N–H), 3050 cm−1 (OH); ˡH-NMR (DMSO-d6) δ-ppm; 2.26 (s, 3H, CH3), 2.29 (s, 3H, CH3), 6.85 (d, 2H, Ar-H, J = 8.4 Hz), 7.20 (s, 1H, Ar-H), 7.22 (dd, 2H, Ar-H, J = 8.4, 2 Hz), 7.43 (d, 2H, Ar-H, J = 2 Hz), 8.85 (s, 1H, CH
N), 12.81 (s, 1H, OH),; 13C-NMR (δ-ppm); 19.4, 20.3, 115.6, 128.9, 129.6, 130.6, 130.9, 136.2, 140.8, 154.3, 154.9, 163.0; anal. calcd for C24H24 N2O2 (372.18): C = 77.39, H = 6.50, N = 7.52; found (%): C = 77.51, H = 6.59, N = 7.67.
6,6'-((1E,1′E)-((4,5-Dimethyl-1,2-phenylene)bis(azaneylylidene))bis(methaneylylidene))bis(2,4-di-tert-butylphenol) (DAM). Yield 90%, mp. 3.66–368 °C.; FT-IR (cm−1): 1586 cm−1 (C
N), 3287 cm−1 (N–H), 3570 cm−1 (OH); ˡH-NMR (DMSO-d6); δ-ppm; 1.29 (s, 9H, CH3), 1.39 (s, 9H, CH3), 2.31 (s, 3H, CH3), 7.35–7.38 (m, 2H, Ar-H), 7.48 (d, 1H, J = 8.0 Hz, Ar-H), 8.97 (s, 1H, CH
N), 13.8 (s, 1H, OH); 13C-NMR (δ-ppm); 19.4, 20.3, 24.1, 115.6, 116.4, 117.3, 117.6, 128.9, 130.9, 132.5, 140.8, 154.8, 168.4; anal. calcd for C38H52 N2O2 (568.40): C = 80.24, H = 9.21, N = 4.92; found (%): C = 80.45, H = 9.05, N = 4.99.
4,4'-((1E,1′E)-((4,5-Dimethyl-1,2-phenylene)bis(azaneylylidene))bis(methaneylylidene))bis(benzene-1,3-diol) (DMD). Yield 75%, mp. 315–317 °C; FT-IR (cm−1): 1586 cm−1 (C
N), 3287 cm−1 (N–H); ˡH-NMR (DMSO-d6); δ-ppm; 2.28 (s, 3H, CH3), 6.28 (d, 1H, Ar-H, J = 2.2 Hz), 6.38 (dd, 1H, Ar-H, J = 2.2 Hz, 8.4 Hz), 7.20 (s, 1H, Ar-H), 7.42 (d, 1H, Ar-H, J = 8.4 Hz), 8.74 (s, 1H, CH
N), 12.25 (s, 1H, NH), 13.54 (s, 1H, OH); 13C-NMR(δ-ppm) 19.5, 102.8, 108.1, 112.7, 120.6, 134.7, 135.7, 139.8, 162.4, 162.8, 163.8; anal. calcd for C22H20 N2O4 (376.14): C = 70.20, H = 5.36, N = 7.44; found (%): C = 70.45, H = 5.31, N = 7.59.
Computational details
The DFT-based calculations of the studied compounds: DMA, DMM, DMB-D, DAM, and DMD were performed by using Gaussian 09 computational database package.21 The obtained output files were further analyzed by GaussView,22 Avogadro,23 Chemcraft 24PyMOlyze 2.0,25 Origin 8.0,26 and Multiwfn 3.7,27programs. The analysis of NBO was done by NBO 3.1 program28 at M06-2X/6-311G(d,p). Moreover, UV-vis spectrum were studied using the same level of theory to find out the maximum wavelength of light absorbed by the compounds. Moreover, NLO characteristics (dipole moment, 1st and 2nd hyperpolarizability) along with their tensor components in x, y, and z coordinates were computed at the abovementioned functional. NPA, DOS, and MEP were also computed at the same level. The FMO inspection was executed by using the same level of DFT and the energy difference between its orbitals was utilized to compute the global reactivity indices. The dipole moment (μ),29 average linear polarizability 〈α〉, first hyperpolarizability (βtot), and second hyperpolarizability γtot amplitudes were calculated by applying the given formulas27 (eqn (1)–(4)). |
μ = (μx2 + μy2 + μz2)1/2
| (1) |
|
〈α〉 = (axx + ayy + azz)/3
| (2) |
|
βtot = (βx2 + βy2 + βz2)1/2
| (3) |
where βx = βxxx+βxyy+βxzz, βy = βyxx+βyyy+βyzz and βz = βzxx+βzyy+βzzz. |
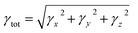 | (4) |
where
.
Results and discussion
The structures of the synthesized Schiff bases were determined by employing microanalysis (CHN) and spectral outcomes, i.e., UV-vis, FT-IR, 13C-NMR, 1H-NMR spectroscopy. The C
N stretching band for different compounds in FT-IR was emerged in the range of 1484–1605 cm−1, whereas N–H stretching appeared in the range of 3152–3469 cm−1. In 1H-NMR, the O–CH2 signal appeared as a quartet at δ 4.04–4.07 ppm, and CH3 attached to O–CH2 appeared as a triplet due to the presence of neighboring CH2 at δ 1.35 ppm, while the –CH
N– signal appeared as a singlet at δ 8.70–8.90 ppm. The spectral results of other aromatic and aliphatic protons were also in correspondence with the suggested structures of the molecules in the Tables S1, S4, S7 and S10.† In this work, DFT calculations were done to obtain the NLO parameters of four different synthesized compounds: DMA, DMM, DAM, and DMD and one designed compound DMB-D. DMB-D was designed from DAM in order to check the effect of single tertiary butyl group over the donor region. Compound DMA is an A–π–A type, whereas the configuration of DMM, DMB-D, DAM, and DMD is D–π–D (see Fig. S1†). The Chemdraw structures of compounds namely (N1E,N2E)-4,5-dimethyl-N1,N2-bis(2-nitrobenzylidene)benzene-1,2-diamine (DMA), 2,2'-((1E,1′E)-((4,5-dimethyl-1,2-phenylene)bis(azanylylidene))bis(methanylylidene))bis(4-methylphenol) (DMM), 6,6'-((1E,1′E)-((4,5-dimethyl-1,2-phenylene)bis(azanylylidene))bis(methanylylidene))bis(2-(tert-butyl)phenol) (DMB-D), 6,6'-((1E,1E)-((4,5-dimethyl-1,2-phenylene)bis(azanylylidene))bis(methanylylidene))bis(2,4-di-tert-butylphenol) (DAM), and 4,4'-((1E,1′E)-((4,5-dimethyl-1,2-phenylene)bis(azanylylidene))bis(methanylylidene))bis(benzene-1,3-diol) (DMD) are displayed in Scheme 2. The core unit, i.e., (4,5-dimethyl-N1,N2-dimethylenebenzene-1,2-diamine) is the same in all the explored compounds, which act as π-bridge. DFT calculations were accomplished to compute the UV-vis spectrum, FMO, NBO, linear polarizability 〈α〉, first hyperpolarizability (βtot), and second hyperpolarizability (γtot). The energy gap had a great influence on the optical and NLO characteristics of the compounds. The consequences obtained from the computational analysis of the mentioned compounds show that these compounds are effective in non-linear optics. The chemical and optimized geometries of the investigated molecules are displayed in Fig. 1 and S13†, respectively.
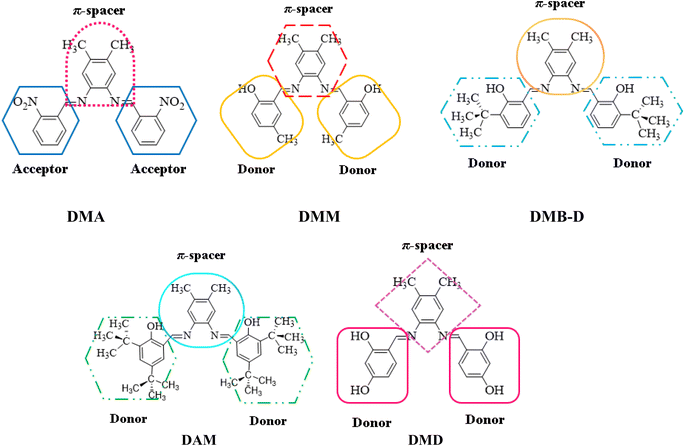 |
| Scheme 2 A sketch map of the entitled compounds. | |
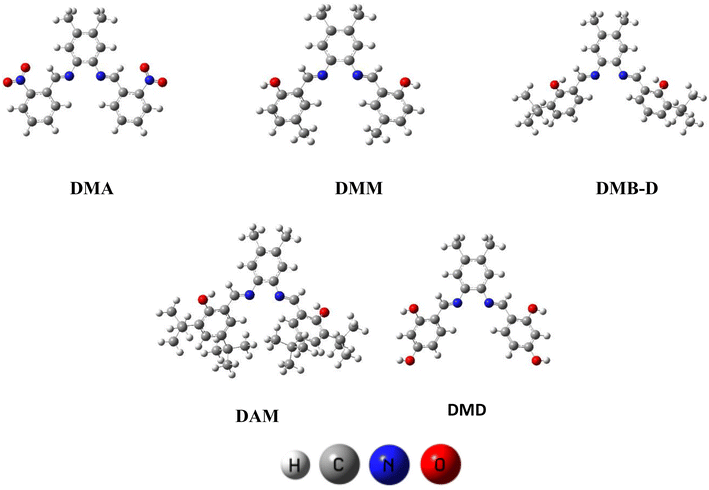 |
| Fig. 1 Optimized geometries of the synthesized compounds. | |
Frontier molecular orbital (FMO) investigation
The energy difference in the frontier molecular orbitals is utilized to evaluate the optical polarizability, chemical response, global hardness, and softness of the examined molecules.30,31 The HOMO is termed as a donor orbital as it is filled with electrons, while the LUMO is a partially filled or vacant orbital, which is called as an acceptor orbital.32,33 The pictographic representation is shown in Fig. 2, and Table 1 shows the band gap values between LUMO–HOMO of the studied compounds. The EHOMO − ELUMO gap is directly associated with the hardness (η) and chemical potential (μ) of a compound and is inversely associated with the global softness (σ) and reactivity.34 The lesser the energy gap, the better will be the electron transport from HOMO to LUMO, and the greater will be the polarizability of the molecules.30
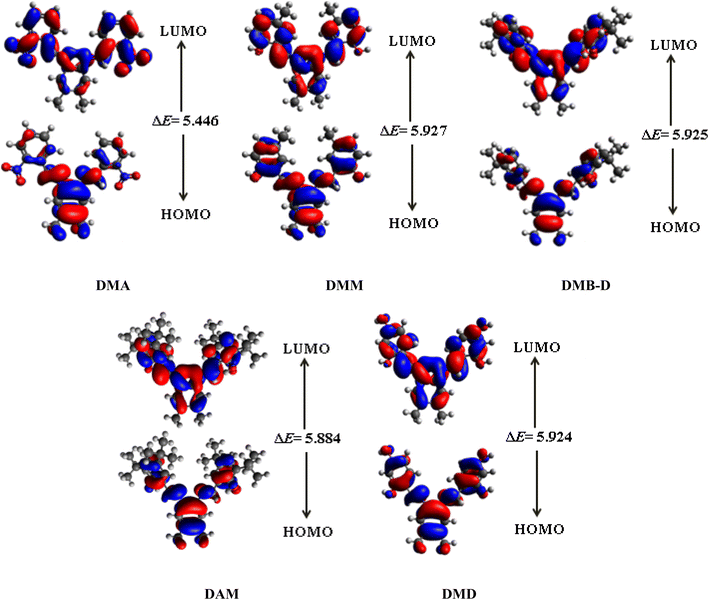 |
| Fig. 2 Frontier molecular orbitals of the entitled compounds. | |
Table 1 HOMO/LUMO energies and band gaps of FMOs of the investigated structuresa
Comp. |
EHOMO |
ELUMO |
ΔE (eV) |
Units are in eV. |
DMA |
−7.338 |
−1.892 |
5.446 |
DMM |
−6.986 |
−1.059 |
5.927 |
DMB-D |
−7.129 |
−1.204 |
5.925 |
DAM |
−7.066 |
−1.182 |
5.884 |
DMD |
−6.832 |
−0.908 |
5.924 |
It is shown in Table 1 that the minimum band gap value (5.446 eV) is observed in DMA, which illustrates good intramolecular charge transfer. This excellent charge transfer in DMA may be found to occur due to the strong electron withdrawing effect of NO2 attached at the acceptor site. While the maximum value of the energy gap is noted in DMM as 5.927 eV. The decreasing order of energy gap is DMM > DMB-D > DMD > DAM > DMA. Thus, DMA is an unstable and chemically reactive compound. It can be seen from Fig. 2 that electronic charge density is largely observed to be concentrated over the spacer region in HOMOs, whereas in the LUMO pictograph, it is found over terminal regions and partially over the spacer part, which indicates a significant charge transfer.
Global reactivity descriptors (GRDs)
The LUMO–HOMO band gap is utilized to calculate the GRDs. The results of these indices, such as ionization potential (I),35 electron affinity (EA), global hardness (η),36 global electrophilicity (ω),37 electronegativity (X),38 global softness (σ), and chemical potential (μ)39 are estimated with the help of eqn (5)–(11), and their outcomes are tabulated in Table 2. |
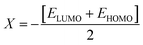 | (7) |
|
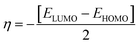 | (8) |
|
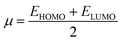 | (9) |
|
 | (10) |
|
 | (11) |
Table 2 Global reactivity parameters of DMA, DMM, DMB-D, DAM, and DMD a
Comp. |
IP |
EA |
X |
η |
μ |
ω |
σ |
Units are in eV. |
DMA |
7.338 |
1.892 |
4.615 |
2.723 |
−4.615 |
3.910 |
0.183 |
DMM |
6.986 |
1.059 |
4.022 |
2.963 |
−4.022 |
2.729 |
0.168 |
DMB-D |
7.129 |
1.204 |
4.166 |
2.962 |
−4.166 |
2.929 |
0.168 |
DAM |
7.066 |
1.182 |
4.124 |
2.942 |
−4.124 |
2.890 |
0.169 |
DMD |
6.832 |
0.908 |
3.870 |
2.962 |
−3.870 |
2.528 |
0.168 |
The electron giving and gaining the capability of molecules is calculated from their EA and IP values, respectively.40 The ionization potential values of DMA, DMM, DMB-D, DAM, and DMD are 7.338, 6.986, 7.129, 7.066, and 6.832 eV, respectively, while electron affinity (EA) values are 1.892, 1.059, 1.204, 1.182, and 0.908 eV, respectively. The EA values of the entitled compounds are smaller than IP, which indicates that the studied compounds have greater electron-donating capacity. The highest 0.183 eV softness is shown by compound DMA, and the lowest 0.168 eV global softness value is exhibited by compounds DMM, DMB-D, and DMD, respectively. The overall descending order of softness is as follows: DMA > DAM > DMM > DMB-D > DMD. The chemical potential of the investigated compounds in declining order is DMA > DMB-D > DAM > DMM > DMD. Among the studied compounds, DMA has the greatest amplitude of electrophilicity index (3.910 eV) and DMD has the smallest value (2.528 eV). The electronegativity (X) values for DMA to DMD are found to be 4.615, 4.022, 4.166, 4.124 and 3.870 eV. These findings revealed that compound DMA has the highest softness value, which indicates that it is less stable, highly reactive and shows significant NLO response.
Natural bonding orbital (NBO) analysis
The NBO approach is utilized to observe the electronic charge transference phenomenon from electron donating to electron accepting moieties, hyper conjugative and non-covalent interactions (inter and intra-molecular hydrogen bonding) in the molecule. The second-order perturbation energy is calculated by using eqn (12). |
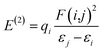 | (12) |
where, E(2) represents stability energy, εj − εi indicates diagonal elements, qi depicts orbital occupancy, and F(i,j) shows off-diagonal NBOs Fock matrix components.41 The NBO analysis of the studied compounds is performed via using the abovementioned functional and some of its outcomes are displayed in Table 3, while rest of the transitions are added in Tables S1–S4.†
Table 3 Natural bond orbital (NBO) analysis of the entitled compounds
Comp. |
Donor (i) |
Type |
Acceptor (j) |
Type |
E(2) [kcal mol−1] |
E(j) − E(i) (a.u.) |
F(i,j) (a.u.) |
DMA |
C19–C20 |
σ |
C24–N43 |
σ* |
5.89 |
1.11 |
0.073 |
C28–C33 |
σ |
C28–N46 |
σ* |
0.53 |
1.14 |
0.022 |
C21–C22 |
π |
C23–C24 |
π* |
34.52 |
0.34 |
0.097 |
N46–O48 |
π |
C28–C29 |
π* |
2.90 |
0.59 |
0.041 |
O44 |
LP(3) |
N43–O45 |
π* |
214.63 |
0.22 |
0.197 |
O47 |
LP(2) |
C28–C29 |
σ* |
0.65 |
1 |
0.023 |
DMM |
O47–H48 |
σ |
C28–C33 |
σ* |
5.72 |
1.46 |
0.082 |
C5–H8 |
σ |
C4–N17 |
σ* |
0.53 |
1.1 |
0.022 |
C20–C21 |
π |
C22–C23 |
π* |
32.90 |
0.35 |
0.096 |
N17–C49 |
π |
C19–C24 |
π* |
8.23 |
0.45 |
0.060 |
O45 |
LP(2) |
C19–C24 |
π* |
33.66 |
0.46 |
0.120 |
O45 |
LP(1) |
C19–C24 |
σ* |
0.55 |
1.31 |
0.024 |
DMB-D |
C20–H25 |
σ |
C19–C24 |
σ* |
5.06 |
1.21 |
0.070 |
C22–C23 |
σ |
C43–C48 |
σ* |
0.51 |
1.21 |
0.022 |
C28–C29 |
π |
C27–C32 |
π* |
30.73 |
0.35 |
0.094 |
N17–C37 |
π |
C19–C24 |
π* |
7.80 |
0.45 |
0.058 |
O35 |
LP(2) |
C19–C24 |
π* |
29.12 |
0.46 |
0.112 |
O36 |
LP(1) |
C27–C28 |
σ* |
0.50 |
1.29 |
0.023 |
C20–H25 |
σ |
C19–C24 |
σ* |
5.03 |
1.21 |
0.070 |
C22–C23 |
σ |
C41–C42 |
σ* |
0.52 |
1.21 |
0.022 |
C1–C6 |
π |
C4–C5 |
π* |
29.93 |
0.34 |
0.091 |
DAM |
C19–C28 |
π |
C19–C24 |
π* |
7.93 |
0.46 |
0.059 |
O35 |
LP(2) |
C19–C24 |
π* |
29.10 |
0.46 |
0.112 |
O36 |
LP(1) |
C27–C28 |
σ* |
0.50 |
1.29 |
0.023 |
DMD |
O37–H38 |
σ |
C27–C32 |
σ* |
5.62 |
1.46 |
0.081 |
C2–H7 |
σ |
C3–N18 |
σ* |
0.52 |
1.1 |
0.021 |
C22–C23 |
π |
C19–C24 |
π* |
36.31 |
0.36 |
0.105 |
C17–C39 |
π |
C19–C24 |
π* |
7.97 |
0.37 |
0.012 |
O45 |
LP(2) |
C22–C23 |
π* |
38.03 |
0.45 |
0.125 |
O45 |
LP(1) |
C21–C22 |
σ* |
0.55 |
1.3 |
0.024 |
Generally, four major kinds of electronic transitions are observed, i.e., σ → σ*, π → π*, LP → σ*, and LP → π*. π → π* is found to be the most probable electronic transition. From these, π(C21–C22) → π*(C23–C24), π(C20–C21) → π*(C22–C23), π(C28–C29) → π*(C27–C32), π(C1–C6) → π*(C4–C5), and π(C22–C23) → π*(C19–C24) have stabilization energy values of 34.52, 32.90, 30.73, 29.93, and 36.31 kcal mol−1 in DMA, DMM, DMB-D, DAM and DMD respectively. The transitions likeπ(N46–O48) → π*(C28–C29), π(N17–C49) → π*(C19–C24), π(N17–C37) → π*(C19–C24), π(C19–C28) → π*(C19–C24), and π(C17–C39) → π*(C19–C24) with energy values of 2.90, 8.23, 7.80, 7.93, and 7.97 kcal mol−1 are observed in DMA, DMM, DMB-D, DAM and DMD, accordingly. These are the lowest stability energy amplitudes among all the π → π* transitions in the examined compounds. Likewise, σ(C19–C20) → σ*(C24–N43), σ(O47–H48) → σ*(C28–C33), σ(C20–H25) → σ*(C19–C24), σ(C20–H25) → σ*(C19–N24), and σ(O37–H38) → σ*(C27–C32) transitions are observed with a maximum magnitude as 5.89, 5.72, 5.06, 5.03, and 5.62 kcal mol−1 in DMA, DMM, DMB-D, DAM and DMD, respectively. Similarly, σ(C28–C33) → σ*(C28–N46), σ(C5–H8) → σ*(C4–N17), σ(C22–C23) → σ*(C43–C48), σ(C22–C23) → σ* (C41–N42), and σ(C2–H7) → σ*(C3–N18) transitions with the lowest energy values of 0.53, 0.53, 0.51, 0.52, and 0.52 kcal mol−1 are detected in DMA, DMM, DMB-D, DAM and DMD, correspondingly.
Furthermore, the most prominent LP → π* transitions are LP3(O44) → π*(N43–O45), LP2(O45) → π*(C19–C24), LP2(O35) → π*(C19–C24), LP2(O35) → π*(C19–C24), and LP2(O45) → π*(C22–C23) with 214.63, 33.66, 29.12, 29.10, and 38.03 kcal mol−1 stabilization energy. Similarly, LP2(O47) → σ*(C28–C29), LP1(O45) → σ*(C19–C24),LP1(O36) → σ*(C27–C28), LP1(O36) → σ*(C27–C28), and LP1(O45) → σ*(C21–C22) manifested minimum energy of 0.65, 0.55, 0.50, 0.50, and 0.55 kcal mol−1, correspondingly. Some other electronic transitions are also observed, which are presented in the Tables S1–S4.† The NBO investigation disclosed the delocalization of electrons and hyper conjugative interactions, which in turn cause ICT, are the foremost reasons for the stability of the molecule and are crucial for good NLO response.
Density of states (DOS)
The scattering of electronic clouds on different parts of the compound is studied with the help of DOS. To obtain the DOS graph, we made fragments of our compounds, i.e., acceptor, π-spacer (linker) in DMA and donor, π-linker in DMA, DMM, DMB-D, and DMD. The PDOS (partial density of states) and TDOS (total density of states) graphs of the synthesized compounds are examined and presented in Fig. 3. In DMA, the contribution of 85.6% is made by the acceptor to LUMO, while it contributes 18.2% to HOMO, whereas the π-spacer shows 14.4% of its involvement to LUMO and 81.8% to HOMO. In the cases of DMM, DMB-D, DAM and DMD, the donor contributes 66.6, 64.9, 65.2, and 64.9% to LUMO, while a contribution of 30.3, 28.5, 34.4 and 37.6%, respectively and is observed towards HOMO. The π-spacer depicts a 33.4, 35.1, 34.8, and 35.1% contribution pattern to LUMO, while 69.7, 71.5, 62.4, and 65.6% towards HOMO from DMM, DMB-D, DAM and DMD, correspondingly (Table S14†). In the synthesized molecule DMA, most of the electron density is present on the π-spacer in HOMO, whereas in LUMO it is more concentrated on the acceptor part. The other synthesized molecules have identical patterns of distribution of electron density as HOMO has electron density on π-linker (4,5-dimethyl-N1,N2-dimethylenebenzene-1,2-diamine), while LUMO has more density on the donor and a little bit is present on π-linker.
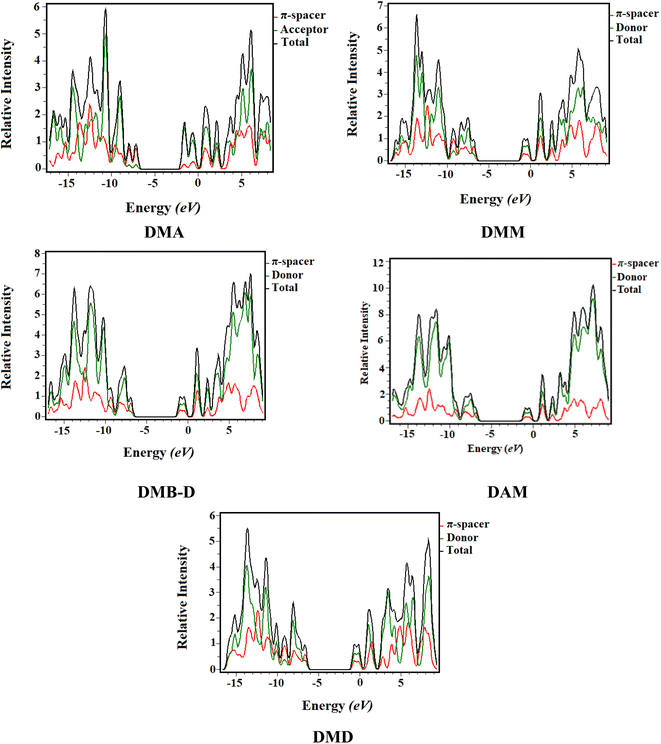 |
| Fig. 3 Graphical representation of DOS for the entitled compounds. | |
UV-vis analysis
UV-vis analysis provides significant information regarding the charge transfer capability, nature of transitions, and phenomenon of absorption in the synthesized compounds (DMA, DMM, DMB-D, DAM, and DMD).42 TD-DFT is employed to calculate the photophysical characteristics of DMA, DMM, DMB-D, DMB, and DMD using the dichloromethane (DCM) solvent. The maximum wavelength (λmax) of light absorbed, excitation energy (E), molecular orbital (MO) contributions, and oscillator strength (f)of the studied molecules are calculated by the same technique of TD-DFT and the outcomes are represented in Table 4, whereas, results of other molecular orbital contributions are displayed in Tables S5–S9.†
Table 4 Excitation energy (E), oscillator strength (f), and wavelength (λ) and of studied moleculesa
Comp. |
DFT λ (nm) |
E (eV) |
f |
MO contributions |
MO = molecular orbital, H=HOMO, L = LUMO, f = oscillator strength. |
DMA |
364.068 |
3.405 |
0.254 |
H → L(67%), H−1 → L+1(2%) |
DMM |
346.196 |
3.581 |
0.286 |
H → L(77%), H−1 → L+1(3%) |
DMB-D |
347.811 |
3.564 |
0.352 |
H → L(79%), H−1 → L+1(3%) |
DAM |
348.956 |
3.553 |
0.353 |
H → L(77%), H−1 → L+1(3%) |
DMD |
341.477 |
3.631 |
0.408 |
H → L(79%), H−1 → L+1(4%) |
It is observed from the results that transition energy is inversely proportional to λmax and directly related to oscillation strength. As the excitation energy value and oscillation strength increase, the wavelength of absorption maxima decreases. Therefore, the highest λmax value of 364.068 nm was obtained in DMA with 3.405 eV excitation energy and 0.254 oscillation strength. Hence, it showed a bathochromic shift. Remarkably, the highest value of λmax in DMA is due to the existence of strong electron withdrawing (–NO2) groups, which decrease the energy gap (EHOMO − ELUMO). An appreciable decrease in the value of λmax is observed in DMB-D (347.811 nm). The minimum value of λmax value is 341.477 nm, as observed in DMD. The descending trend of λmax of the investigated molecules is as follows: DMA > DAM > DMB-D > DMM > DMD (Fig. 4).
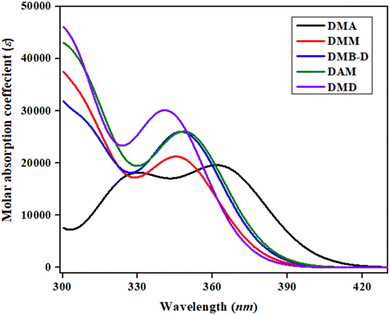 |
| Fig. 4 Absorption spectrum of DMA, DMM, DMB-D, DAM, and DMD. | |
Natural population analysis (NPA)
The electronic cloud distribution on an atom has a great impact on the chemical reactivity, dipole moment, and electrostatic interaction between the atoms and molecules, and many other properties of the chemical system. The effective calculation of the atomic charge of the titled compounds is essential for a better understanding of the conjugated system.43,44 The Mulliken charges of DMA, DMM, DMB-D, DAM and DMD were calculated at the aforementioned level and are presented in Fig. 5.
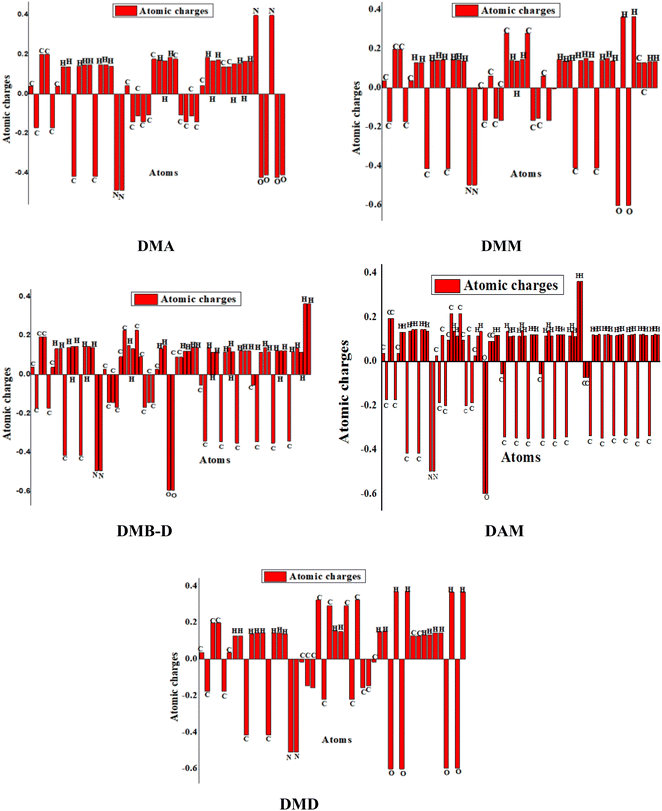 |
| Fig. 5 Natural population analysis of DMA, DMM, DMB-D, DAM, and DMD. | |
The charge density distribution revealed that the nitrogen atoms associated with oxygen in DMA are positively charged, whereas those attached to carbon and hydrogen in all of the titled compounds are negatively charged. Moreover, all the hydrogen atoms are positively charged. The oxygen atoms linked with carbon and hydrogen atoms are negatively charged. All the nitrogen atoms present in the π-linker are negatively charged (see Fig. 5). The overall analysis of Mulliken charges revealed that the unequal distribution of charges on the entitled compounds is because of the nitrogen and oxygen atoms.
Molecular electrostatic potential (MEP)
The MEP plot is widely used to predict electrostatic potential, chemical reactivity, electrophilic, and nucleophilic attacks on the reactive site in a molecular system. The MEP diagram is mapped over the optimized structure of the molecule to signify the overall electron density. The different colors are utilized to designate the electrostatic potential over the MEP surface, shown in Fig. 6. The enhancing trend of potential is: red < orange < yellow < green < blue.45,46 The MEP plot displayed that most of the red color is on oxygen atoms, which showed the probability of electrophilic attack. Although most of the green and blue bands which elucidate positive potential are positioned on carbon and hydrogen atoms that are efficient for nucleophilic reactions.
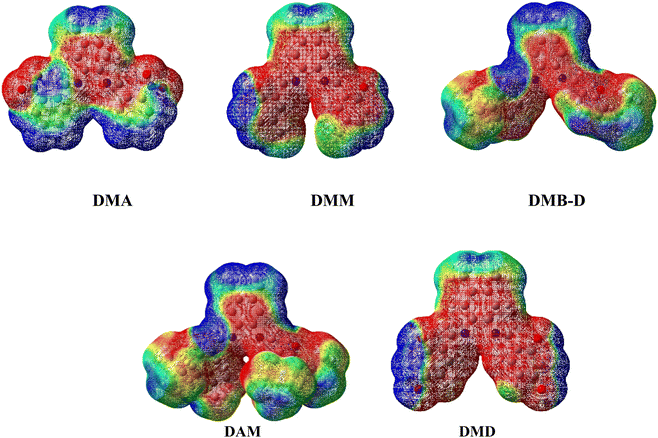 |
| Fig. 6 MEP representation of the entitled compounds. | |
Non-linear optical (NLO) properties
The materials that display NLO properties have become an extensive research discipline because of their widespread applications in optoelectronics, telecommunication, and optical switches.47 The dipole moment (μ) and hyperpolarizability (βtot and γot) display the electronic communication in different fragments of the molecule.48 The data computed for dipole moment (μ), average polarizability and hyper polarizabilities (βtot and γtot) are tabulated in Table 5 while the major contributing tensors are presented in Tables S10–S13.†
Table 5 The, average polarizability, dipole moment, first hyperpolarizability, second hyperpolarizability results for investigated moleculesa
Comp. |
μtot |
〈α〉 × 10−25 |
βtot × 10−33 |
γtot × 10−35 |
μtot has a unit in D, while 〈α〉, βtot and γtot have units in e.s.u. |
DMA |
2.385 |
561.702 |
21 129.545 |
20.761 |
DMM |
0.705 |
566.066 |
161.594 |
17.170 |
DMB-D |
0.882 |
700.910 |
942.348 |
19.320 |
DAM |
0.659 |
877.438 |
3335.274 |
21.239 |
DMD |
0.202 |
549.863 |
25 438.874 |
20.373 |
The utmost value of μtot (2.385 D) is viewed in DMA while the least possible value (0.202 D) is noticed in DMD. For a better analysis of NLO behavior, the dipole moment and first hyperpolarizability of the studied compounds are compared with the urea molecule, which has been regarded as a standard molecule in the literature study. The dipole moment of DMA is greater than urea (1.3732 D),42 while DMM, DMB-D, DAM, and DMD have smaller dipole moment values than urea.
The highest value of first-order polarizability (877.438 × 10−25 e.s.u.) is noted for compound DAM, whereas the lowest value (549.863 × 10−25 e.s.u.) is examined for compound DMD among the remaining synthesized compounds. The overall decreasing trend of 〈α〉 is in the following order: DAM > DMB-D > DMM > DMA > DMD. The 〈α〉 of the titled compounds is more dominant along the y-direction, which means that polarization predominantly takes place in the y-direction. The linear polarizability in αxx, αyy, and αzz directions is 981.133, 983.541, and 667.438 × 10−25 e.s.u., correspondingly in DAM, which is the highest than the linear polarizability of other entitled compounds as shown in Table S11.† The lowest values of dipole polarizability in x, y, and z-direction are 675.571, 716.639, and 257.399 × 10−25 e.s.u., respectively, observed in DMD.
In first hyperpolarizability (βtot), the tensor βxxy has the major contribution in βtot value with 12
747.514 × 10−33 e.s.u. amplitude in DMA while in DMM, DMB-D, DAM, and DMD the major involvement of βtot is made by βyzz (160.102, 6.038, 1176.196, and 380.946 × 10−33 e.s.u.), respectively. The declining trend βtot values are: DMD > DMA > DAM > DMB-D > DMM. A comparative study of βtot of the studied compounds with urea showed that the values of βtot of DMA, DMM, DMB-D, DAM, and DMD are 56.87, 0.43, 2.53, 8.98, and 68.47 times larger than urea (βtot = 3.71 × 10−31 e.s.u.),49 correspondingly.
Moreover, compound DAM shows the largest 21.239 × 10−35 e.s.u. second hyperpolarizability (γtot) value, and the smallest value of γtot 19.320 × 10−35 e.s.u., is depicted by compound DMM. The γtot values of other synthesized compounds are in the following order: DAM > DMA > DMD > DMB-D > DMM. In the case of the second hyperpolarizability, the greatest involvement of tensor γy is observed for compound DMM with a value of9.181 × 10−35 e.s.u.However, the tensor γz has a minor contribution in γtot value with an amplitude of 0.228 × 10−35 e.s.u. examined in compound DMM. From the above discussion, it is predicted that because of the higher values of dipole moment and first hyperpolarizability compound, DAM might act as an excellent NLO material.
Conclusion
In the present work, 4,5-dimethyl-o-phenylenediamine based Schiff base compounds (DMA, DMM, DMB-D, DAM, and DMD) were synthesized and computationally analyzed to investigate their NLO characteristics. The highest bathochromic shift (λmax = 364.068 nm) was shown by DMA as compared to other compounds. The band gap was lowered from 5.927 to 5.446 eV and among all the compounds, the lowest Egap was found in DAM (5.446 eV), which revealed a good transfer of charge within the molecule. However, in the case of NLO analysis, there was an enhancement in the βtot value (25
438.874 × 10−33 e.s.u.) of DMA as compared to other molecules. The σ → σ* and π → π* interactions with 5.89 and 34.52 kcal mol−1 stabilization energy in DMA observed are dominant over the rest of the studied compounds, which was further supported by the results of global reactivity indices and uncovered the greatest chemical potential, electron donating and accepting competency of DMA. So, it is concluded that DMA and DAM exhibit excellent NLO material properties and might be the best candidates for future applications.
Conflicts of interest
The authors declare no conflicts of interest.
Acknowledgements
The authors would like to thank King Khalid University's Deanship of Scientific Research for funding this study under grant number (R.G.P.1/28/43). Z. S. is thankful to Higher Education Commission, Islamabad, Pakistan through Project No. NRPU/6975 for financial support.
References
- A. Rauf, et al., Synthesis, spectroscopic characterization, DFT optimization and biological activities of Schiff bases and their metal (II) complexes, J. Mol. Struct., 2017, 1145, 132–140 CrossRef CAS.
- A. Rauf, et al., Synthesis, physicochemical elucidation, biological screening and molecular docking studies of a Schiff base and its metal (II) complexes, Arab. J. Chem., 2020, 13, 1130–1141 CrossRef CAS.
- C. R. Schmid, et al., Synthesis of 2, 3-O-isopropylidene-D-glyceraldehyde in high chemical and optical purity: observations on the development of a practical bulk process, J. Org. Chem., 1991, 56, 4056–4058 CrossRef CAS.
- B. B. Baldaniya, Synthesis and Characterizations ofN2 (Aryl)-N4, N6-bis (6, 7-dichloro-1, 3-benzothiazol-2-yl)-1, 3, 5-triazine-2, 4, 6-triamines as Biological Potent Agents, Electron. J. Chem., 2010, 7, 210–214 CAS.
- S. V. Bhandari, et al., Design, synthesis and pharmacological screening of novel nitric oxide donors containing 1, 5-diarylpyrazolin-3-one as nontoxic NSAIDs, Eur. J. Med. Chem., 2009, 44, 4622–4636 CrossRef CAS PubMed.
- L. Jiang, et al., Direct and mild palladium-catalyzed aerobic oxidative synthesis of imines from alcohols and amines under ambient conditions, Chem. Commun., 2011, 47, 10833–10835 RSC.
- K. Hoesch and T. v. Zarzecki, Eine neue Synthese aromatischer Ketone. II. Künstliche Darstellung des Maclurins und ihm verwandter Ketone, Berichte Dtsch. Chem. Ges., 1917, 50, 462–468 CrossRef CAS.
- M. Khalid, et al., NLO potential exploration for D–π–A heterocyclic organic compounds by incorporation of various π-linkers and acceptor units, Arab. J. Chem., 2021, 14, 103295 CrossRef CAS.
- M. Khalid, et al., Structural modulation of π-conjugated linkers in D–π–A dyes based on triphenylamine dicyanovinylene framework to explore the NLO properties, R. Soc. Open Sci., 2021, 8, 210570 CrossRef CAS PubMed.
- M. Khalid, H. M. Lodhi, M. U. Khan and M. Imran, Structural parameter-modulated nonlinear optical amplitude of acceptor–π–D–π–donor-configured pyrene derivatives: A DFT approach, RSC Adv., 2021, 11, 14237–14250 RSC.
- M. Khalid, et al., Enriching NLO efficacy via designing non-fullerene molecules with the modification of acceptor moieties into ICIF2F: an emerging theoretical approach, RSC Adv., 2022, 12, 13412–13427 RSC.
- M. U. Khan, et al., Influence of acceptor tethering on the performance of nonlinear optical properties for pyrene-based materials with A-π-D-π-D architecture, Arab. J. Chem., 2022, 15, 103673 CrossRef CAS.
- M. Ashfaq, et al., Synthetic approach to achieve halo imine units: Solid-state assembly, DFT based electronic and non linear optical behavior, Chem. Phys. Lett., 2022, 803, 139843 CrossRef CAS.
- J. Jia, et al., Effect of intramolecular charge transfer on nonlinear optical properties of chalcone derivatives: a visual description of the charge transfer process, Phys. Chem. Chem. Phys., 2022, 24, 955–965 RSC.
- Y. Shi, et al., Study of ultrafast nonlinear optical response and transient dynamics of pyrene derivatives with intramolecular charge transfer characteristics, Opt. Mater., 2022, 128, 112378 CrossRef CAS.
- D. Pant, N. Darla and S. Sitha, Roles of various bridges on intramolecular charge Transfers, dipole moments and first hyperpolarizabilities of Donor-Bridge-Acceptor types of organic Chromophores: Theoretical assessment using Two-State model, Comput. Theor. Chem., 2022, 1209, 113583 CrossRef CAS.
- H. Purandara, et al., Crystal structure elucidation, experimental and computational optical properties of novel acceptor-donor-acceptor organic material: A suitable candidate for nonlinear applications, J. Mol. Struct., 2022, 1248, 131492 CrossRef CAS.
- D. Pant, N. Darla and S. Sitha, Theoretical assessment of the influences of aromatic bridges on molecular second order nonlinear optical responses of Donor-Bridge-Acceptor types of molecular organic chromophores, Comput. Theor. Chem., 2022, 1207, 113522 CrossRef CAS.
- M. Khalid, et al., Structural modulation of π-conjugated linkers in D–π–A dyes based on triphenylamine dicyanovinylene framework to explore the NLO properties, R. Soc. Open Sci., 2021, 8, 210570 CrossRef CAS PubMed.
- F. Rasool, et al., Facile synthesis, DNA binding, Urease inhibition, anti-oxidant, molecular docking and DFT studies of 3-(3-Bromo-phenyl)-1-(2-trifluoromethyl-phenyl)-propenone and 3-(3-Bromo-5 chloro-phenyl)-1-(2-trifluoromethyl-phenyl)-propenone, J. Mol. Liq., 2021, 336, 116302 CrossRef CAS.
- A. Frisch, Gaussian 09W Reference, Wallingford, USA, 2009, p. 25 Search PubMed.
- R. D. Dennington, T. A. Keith and J. M. Millam, GaussView 5.0, Gaussian. Inc., Wallingford, 2008 Search PubMed.
- M. D. Hanwell, et al., Avogadro: an advanced semantic chemical editor, visualization, and analysis platform, J. Cheminformatics, 2012, 4, 1–17 CrossRef PubMed.
- G. A. Zhurko and D. A. Zhurko, ChemCraft, version 1.6, 2009, http://https//www.Chemcraftprog.com Search PubMed.
- N. M. O’boyle, A. L. Tenderholt and K. M. Langner, Cclib: a library for package-independent computational chemistry algorithms, J. Comput. Chem., 2008, 29, 839–845 CrossRef PubMed.
- R. A. T. Lima, et al., Functional and structural characterization of a novel GH3 β-glucosidase from the gut metagenome of the Brazilian Cerrado termite Syntermes wheeleri, Int. J. Biol. Macromol., 2020, 165, 822–834 CrossRef CAS PubMed.
- T. Lu and F. M. Chen, a multifunctional wavefunction analyzer, J. Comput. Chem., 2012, 33, 580–592 CrossRef CAS PubMed.
- F. Weinhold, C. R. Landis and E. D. Glendening, What is NBO analysis and how is it useful?, Int. Rev. Phys. Chem., 2016, 35, 399–440 Search PubMed.
- L. Kara Zaitri and S. M. Mekelleche, Computational study of linear and nonlinear optical properties of substituted thiophene imino dyes using long-range corrected hybrid DFT methods, Mol. Phys., 2020, 118, 1618508 CrossRef.
- M. N. Arshad, et al., A potent synthesis and supramolecular synthon hierarchy percipience of (E)-N′-(Napthalen-1-yl-methylene)-benzenesulfonohydrazide and 1-Napthaldehyde: A combined experimental and DFT studies, J. Mol. Struct., 2020, 1221, 128797 CrossRef CAS.
- S. H. Sumrra, et al., Synthesis, characterization, and biological screening of metal complexes of novel sulfonamide derivatives: Experimental and theoretical analysis of sulfonamide crystal, Appl. Organomet. Chem., 2020, 34, e5623 CrossRef CAS.
- G. Mahalakshmi and V. Balachandran, NBO, HOMO., LUMO analysis and vibrational spectra (FTIR and FT Raman) of 1-Amino 4-methylpiperazine using ab initio HF and DFT methods, Spectrochim. Acta. A. Mol. Biomol. Spectrosc., 2015, 135, 321–334 CrossRef CAS PubMed.
- M. Adeel, et al., Synthesis, X-ray crystallographic, spectroscopic and computational studies of aminothiazole derivatives, J. Mol. Struct., 2017, 1131, 136–148 CrossRef CAS.
- T. Hassan, et al., Development of non-fused acceptor materials with 3D-Interpenetrated structure for stable and efficient organic solar cells, Mater. Sci. Semicond. Process., 2022, 151, 107010 CrossRef CAS.
- C.-G. Zhan, J. A. Nichols and D. A. Dixon, Ionization potential, electron affinity, electronegativity, hardness, and electron excitation energy: molecular properties from density functional theory orbital energies, J. Phys. Chem. A, 2003, 107, 4184–4195 CrossRef CAS.
- R. G. Parr and R. G. Pearson, Absolute hardness: companion parameter to absolute electronegativity, J. Am. Chem. Soc., 1983, 105, 7512–7516 CrossRef CAS.
- R. Parthasarathi, V. Subramanian, D. R. Roy and P. K. Chattaraj, Electrophilicity index as a possible descriptor of biological activity, Bioorg. Med. Chem., 2004, 12, 5533–5543 CrossRef CAS PubMed.
- R. G. Parr, R. A. Donnelly, M. Levy and W. E. Palke, Electronegativity: the density functional viewpoint, J. Chem. Phys., 1978, 68, 3801–3807 CrossRef CAS.
- P. Politzer and D. G. Truhlar, Chemical applications of atomic and molecular electrostatic potentials: reactivity, structure, scattering, and energetics of organic, inorganic, and biological systems, Springer Science & Business Media, 2013 Search PubMed.
- A. Irfan, A. R. Chaudhry, S. Muhammad and A. G. Al-Sehemi, Exploring the potential of boron-doped nanographene as efficient charge transport and nonlinear optical material: a first-principles study, J. Mol. Graph. Model., 2017, 75, 209–219 CrossRef CAS PubMed.
- R. Hussain, et al., Enhancement in photovoltaic properties of N, N-diethylaniline based donor materials by bridging core modifications for efficient solar cells, ChemistrySelect, 2020, 5, 5022–5034 CrossRef CAS.
- M. Khalid, et al., Exploration of second and third order nonlinear optical properties for theoretical framework of organic D–π–D–π–A type compounds, Opt. Quantum Electron., 2021, 53, 1–19 CrossRef.
- Z. Demircioğlu, G. Kaştaş, Ç. A. Kaştaş and R. Frank, Spectroscopic, XRD, Hirshfeld surface and DFT approach (chemical activity, ECT, NBO, FFA, NLO, MEP, NPA& MPA) of (E)-4-bromo-2-[(4-bromophenylimino) methyl]-6-ethoxyphenol, J. Mol. Struct., 2019, 1191, 129–137 CrossRef.
- Z. Demircioğlu, Ç. A. Kaştaş and O. Büyükgüngör, X-ray structural, spectroscopic and computational approach (NBO, MEP, NLO, NPA, fukui function analyses) of (E)-2-((4-bromophenylimino) methyl)-3-methoxyphenol, Mol. Cryst. Liq. Cryst., 2017, 656, 169–184 CrossRef.
- R. Mathammal, K. Sangeetha, M. Sangeetha, R. Mekala and S. Gadheeja, Molecular structure, vibrational, UV, NMR, HOMO-LUMO, MEP, NLO, NBO analysis of 3, 5 di tert butyl 4 hydroxy benzoic acid, J. Mol. Struct., 2016, 1120, 1–14 CrossRef CAS.
- B. Khan, et al., Synthetic, spectroscopic, SC-XRD and nonlinear optical analysis of potent hydrazide derivatives: a comparative experimental and DFT/TD-DFT exploration, J. Mol. Struct., 2020, 1200, 127140 CrossRef CAS.
- M. Khalid, et al., First principles study of electronic and nonlinear optical properties of A–D–π–A and D–A–D–π–A configured compounds containing novel quinoline–carbazole derivatives, RSC Adv., 2020, 10, 22273–22283 RSC.
- H. S. Nalwa, T. Watanabe and S. Miyata, Optical Second-Harmonic Generation in Organic Molecular and Polymeric Materials, Photochem. Photophysics, 1992, 5, 103–185 CAS.
- P. N. Prasad and D. J. Williams, Introduction to nonlinear optical effects in molecules and polymers, Wiley, New York, 1991, vol. 1 Search PubMed.
|
This journal is © The Royal Society of Chemistry 2022 |