DOI:
10.1039/D2NR03609F
(Minireview)
Nanoscale, 2022,
14, 15596-15606
The role of crystal facets and disorder on photo-electrosynthesis
Received
1st July 2022
, Accepted 23rd August 2022
First published on 23rd September 2022
Abstract
Photoelectrochemistry has the potential to play a crucial role in the storage of solar energy and the realisation of a circular economy. From a chemical viewpoint, achieving high conversion efficiencies requires subtle control of the catalyst surface and its interaction with the electrolyte. Traditionally, such control has been hard to achieve in the complex multinary oxides used in PEC devices and consequently the mechanisms by which surface exposed facets influence light-driven catalysts are poorly understood. Yet, this understanding is critical to further improve conversion yields and fine-tune reaction selectivities. Here, we review the impact that crystal facets and disorder have on photoelectrochemical reactivity. In particular, we discuss how the crystal orientation influences the energetics of the surface, the existence of defects and the transport of reactive charges, ultimately dictating the PEC activity. Moreover, we evaluate how facet stability dictates the tendency of the solid to undergo reconstructions during catalytic processes and highlight the experimental and computational challenges that must be overcome to characterise the role of the exposed facets and disorder in catalytic performance.
Introduction
Photoelectrocatalysis (PEC) holds the promise of providing a new paradigm for sustainable energy conversion schemes and green synthetic pathways, in favour of a circular economy, as opposed to the current fossil-based methods.1 Solar energy is used to upgrade chemicals, rather than to deliver electricity, which explains why PEC is also termed “artificial photosynthesis”.2
The overall mechanistic picture of the PEC process involves the use of a semiconductor material or complex semiconducting structure, where sequential steps take place, namely: (i) photon absorption, (ii) charge separation, (iii) charge transport towards the surface, and (iv) charge transfer at the interface with the liquid or gaseous medium, thus promoting the reaction.3 Although all these steps are critical to the functional operation of materials, step (iv) brings an additional degree of complexity, when comparing to photovoltaics, since charge carriers need to drive interfacial catalytic processes with high activity, selectivity, and stability. The magnitude of this challenge explains why PV (solar to electricity) rapidly became an established technology, while PEC (solar to chemicals) is only now starting to permeate into the market with promising prototypes.4–6
In order to narrow the gap between the technological development of both technologies, the rational design of materials, synthetic and operation conditions of PEC systems should be built on the detailed understanding of the complex thermodynamic and kinetic properties at the semiconductor/liquid (or gas phase) interface.7–10 In practice, this involves understanding the proceses at very different length-scales, from the operation of an electrochemical cell and its losses, to how the macroscopic structure of the electrode influences the reactive area or how the atomic structure of the surface and its facets, controls the transfer of charges (Fig. 1). Achieving such control is paramount to attain a competitive performance in terms of catalytic activity, selectivity, and stability.
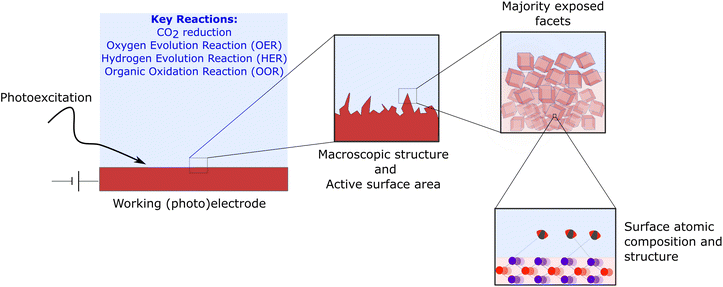 |
| Fig. 1 Multi-scale dimension of photo-electrocatalysis and crystal facets. The development of an efficient PEC cell requires optimising a working electrode (typical area 1 cm2 for lab-scale devices and >50 cm2 for industrial prototypes) and the electrolyte conditions.11 Overall optimisation takes into account the macroscopic structure of the catalyst to enhance the exposed area as well as controlling the dominant exposed facets. The energy of the surface and its atomic compositions determines the binding of intermediates and products and controls the transfer of charge across the interface. | |
Crystal facets and reactivity
When crystalline solids grow, they might exhibit distinct flat surfaces that reflect the symmetry of the crystal. These surfaces are known as facets. Under thermodynamic equilibrium, facets will tend to grow aligned to the crystallographic direction with the lowest energy planes – those that maximise packing. This corresponds to the Wulff structure.12 However, this process can be tuned to favour growth in other directions. For example, a growth-substrate can induce strain in the system and promote growth along a higher energy direction. Similarly, synthesis conditions can trigger or hinder faceting; for instance, high temperatures, close to the melting point, often favour the formation of round-shaped crystals as atoms move more vigorously. In contrast, low temperatures can stimulate the formation of sharp-edged crystals. (See note- before reference list) Fig. 2a shows different facets – labelled as (hkl) using the Miller indices in a cubic unit cell.
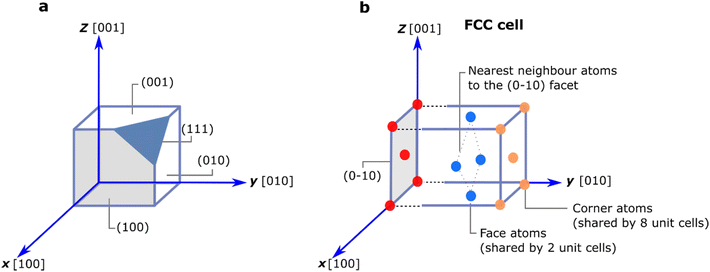 |
| Fig. 2 Facet nomenclature and selection. (a) Different facets of a cubic crystal. hkl are the Miller indices that characterise the lattice plane of a Bravais lattice. (b) In a Face Centered Cubic (FCC) unit cell, the exposure of the (0–10) 2D facet (shown in red), which is parallel to the (010) facet, involves breaking 4 bonds with the nearest neighbour atoms (in blue) per atom in the facet. In general, facets that require breaking a larger number of bonds will have a higher energy. Nonetheless the system can minimise the number of broken bonds and the total energy by undergoing reconstructions and shifting atomic positions. | |
Tuning or exposing a specific facet has important implications for catalysis as the facets relate to the energy of the surface. Depending on the facet, the process involves breaking a different number of bonds (Fig. 2b); generally, surfaces with open surface structures and a large number of dangling bonds (i.e. unsaturated atoms) will exhibit a larger surface energy and thus higher reactivity.13,14 Material scientists have the difficult task of growing and exposing large, high-energy surfaces that are very reactive towards the targeted reaction but also stable under catalytic conditions. Currently, advances in synthetic chemistry are allowing great strides in this direction. However, developments in surface characterisation are also revealing that in many cases pristine catalysts undergo reconstruction and/or disordering processes when brought to catalytic conditions in order to minimise the number of broken bonds.15–19
Facets in photoelectrocatalysis
Several recent reports have highlighted the facet dependent photoelectrocatalysis with metal oxide photoelectrodes like TiO2, WO3, and BiVO4. For example, TiO2 synthesised from a Ti7O4(OEt)20 titanium oxo/alkoxy cluster solution using aerosol assisted chemical vapor deposition, led to a high density of exposed (010) facets, exhibiting an excellent PEC performance towards the Oxygen Evolution Reaction (OER) with an incident photon-to-current efficiency (IPCE) of ∼100% at 350 nm and a Faradaic Efficiency (FE) ∼90%.20
Similarly, in WO3—based OER photocatalysts, synthetic strategies like laser ablation21 or the presence of different crystal growth agents during hydrothermal synthesis,22 led to enhanced PEC performances.22 In particular, it was reported that the exposure of the (002) facet effectively facilitated the separation of photogenerated electron–hole pairs and suppressed the formation of peroxo species. The (010) facet was found to provide more favourable adsorption of reactive ˙OH species, leading to significantly stronger activity towards CH4 oxidation to ethylene glycol under ambient conditions.23
The broad and intricate impact of crystal facets in catalytic performance is also exemplified in BiVO4, a material widely used in water splitting PEC cells.24,25 For instance, catalysts prepared by electrodeposition with the use of lactic acid were found to strengthen the atomic arrangement of the (010) orientation, which improved the transmittance of the BiVO4 photoanode thin film.26 Similarly, the development of this (010) facet in BiVO4 was shown to provide adequate band bending and a deep valence band level, enhancing the production of high-value-added hydrogen peroxide (H2O2) with an average Faradaic efficiency of 70% from water oxidation.27 However, there is intense debate about the reactivity of BiVO4 surfaces, as discussed below.
In the following sections we outline the key roles that different facets have on photo-electrochemistry. We primarily focus on examples of BiVO4 in order to provide a framework to understand the wide-ranging impact of crystal orientation on catalysis. In addition, we discuss the difficulty in stabilising reactive facets and highlight the impact that surface reconstructions and disorder have on electrode performance. Finally, we discuss the need to approach facet control from a holistic view, integrating theory and experiments, and outline exciting areas for future development.
Impact of crystal orientation on chemical reactivity
A new facet can be exposed when a crystal is split, or cleaved, along a specific crystallographic plane (Fig. 2b). Structurally, this involves the rupture of chemical bonds and the generation of a new surface composition in which atoms will have different coordination environments compared to the bulk. This plane cleavage changes the total energy of the surface, the stoichiometry of the termination and thus alters the electrostatic potential leading to changes in the spatial arrangement of the atoms and the motifs exposed.28 Moreover, the new exposed facet will also have a specific orbital orientation and will sustain a unique distribution of electronic charge both along the surface and towards the bulk.
The stoichiometry, electronic and structural patterns that emerge when selecting a specific facet have a direct impact on the catalytic properties of semiconductors.29,30 First, a new exposed facet can change the energy of the frontier orbitals (Conduction Band Minima and Valence Band Maxima, CBM and VBM, respectively) and the Fermi level, impacting band alignment and band bending (Fig. 3a).31–33 Second, the new surface termination changes the adsorption energy of reactants, intermediates and products, thus modifying reaction mechanisms and product distributions (Fig. 3b). Third, the new surface energetics might favour the formation of defects and polarons (Fig. 3c) with implications for both reaction mechanisms and the transport and accumulation of charges (Fig. 3d).34–36 Finally, a new surface termination might influence the ability to deposit co-catalysts for enhanced performance (Fig. 3e) and change interface energetics and the molecular structure of the electrochemical double layer (EDL) (Fig. 3f).37–39
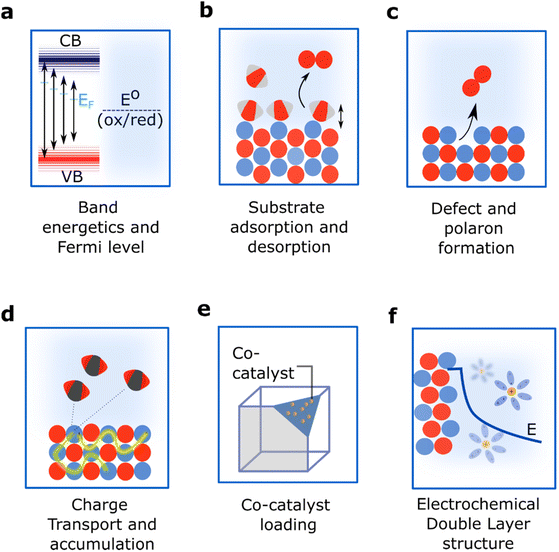 |
| Fig. 3 The role of facets on semiconductor photoelectrocatalysis. The exposed facet will influence (a) the energy of the valence and conduction bands as well as the Fermi level (EF) thus influencing the equilibration with the electrolyte redox level (E°) and the band bending (b) the energy of adsorbates, (c) the tendency to form defects (and polarons) at the surface, (d) the ability of charge carriers to move through the surface and towards the bulk of the solid, (e) the selectivity to deposit co-catalysts and (f) the molecular structure of the interface. | |
In the next sections, we discuss the different role of facets and disorder; for clarity we focus on examples of BiVO4. This metal oxide has a monoclinic crystal structure and belongs to the C62h point group and the space group C2/c (no. 15 in the international tables for crystallography) in the conventional setting, which is used here. Note that different settings, such as the body centered I2/b setting, where the axis and facet notations vary, are sometimes used in the literature.40 Its most stable cleavage plane is orthogonal to the [010] direction, along the longest crystallographic axis in the C2/c setting. The resulting (010) facet is composed of 6-fold coordinated Bi atoms, 4-fold fully coordinated V atoms and 2-fold coordinated O atoms (Fig. 4a).41 This facet has been found to possess good reactivity towards water oxidation and is usually present in high-performing BiVO4-based PEC cells.42,43 The second most stable surfaces include the chemically similar (110), (−110), (−111), and (11–1) facets. Together, they actually provide the largest surface in a thermodynamic equilibrium, as expressed by the Wulff construction.44 Other facets can also be prepared,40 such as the (001) that contain 4-fold and 5-fold coordinated Bi atoms instead.31
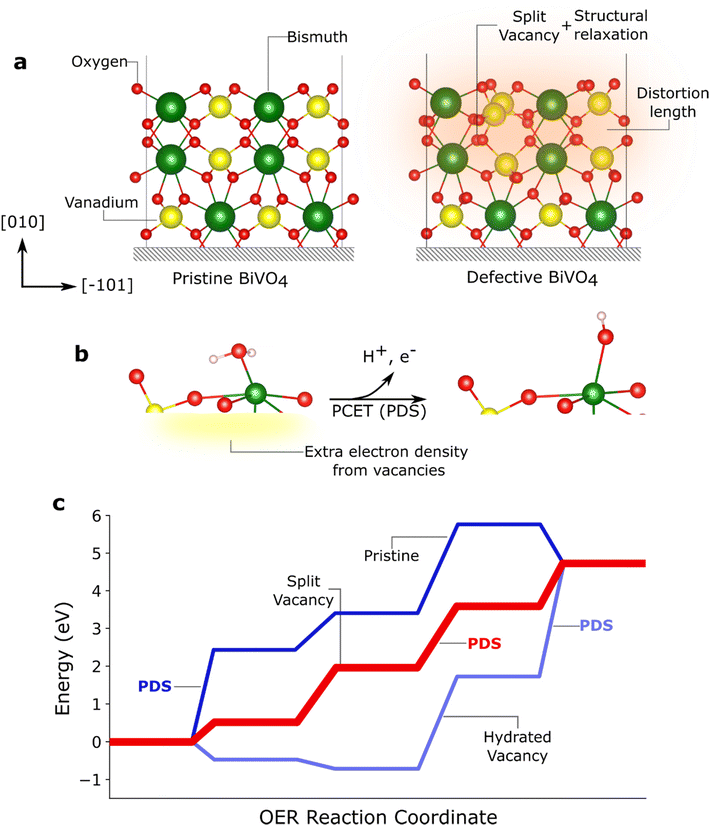 |
| Fig. 4 Role of crystal facets on the formation of defects. (a) Pristine and defective BiVO4 showing how the formation of a split vacancy defect changes the atomic positions along the [010] direction (labelled [001] in the I2/b setting in ref. 34 and 35). The shaded orange area indicates the extent of the structural distortion and movement of atoms due to the formation of the defect. (b) Diagram showing how the presence of a split oxygen vacancy introduces extra electrons which can be more readily removed from the system, lowering the Potential Determining Step (PDS) and enabling a more favourable mechanism. (c) Gibbs free energy profile of the Oxygen Evolution Reaction (OER) on the (011) BiVO4 surface. The step with the highest energy is the PDS. Figure a was adapted from ref. 34 and figure b and c were adapted from ref. 35. | |
Band alignment and intermediate adsorption
Theoretical studies have shown that the exposed facet in BiVO4 influences the band energies. While all facets exhibit a highly oxidising VBM (high position in the energy scale), suitable for OER, the CBM of (010) is more positive than the H+/H2 equilibrium potential, and thus the termination is unreactive towards the hydrogen evolution reaction (HER). Structure sensitivity is clear for this material as, for example, the CBM of the (001) facet is suitable for proton reduction making this facet a good candidate for the full water splitting reaction.31,33 Similar behaviour is observed in p-type Cu2O in which the CBM of rhombic dodecahedral (110)-dominated oxide is more positive than that of the cubic (100) faceted Cu2O. Moreover, the Fermi level of the rhombic Cu2O is located at a more positive potential leading to larger band bending upon equilibration.36
In addition to these effects, modelling of the OER mechanism in BiVO4 has also revealed that the (110) and (−110) surfaces exhibit more favourable energetics for the reaction compared to other facets. The authors, attributed this to a stronger adsorption energy for the O* intermediate that is generated from the oxidation of adsorbed H2O.33
Defect formation and surface reactivity
Preparation of oxides is mostly performed through thermal treatments; thus, these semiconductors are prone to lose oxygen, generating vacancies and leaving uncoordinated atoms in the lattice.45–49 The resulting defective surface or material can be thermodynamically stabilised by structural rearrangements, in which atoms attempt to maximise their coordination number. This ability of a surface to stabilise a vacancy is closely related to the properties of the constituent atoms and consequently, defect formation is facet dependent. Indeed, in BiVO4 the (010) facet has been found to sustain oxygen vacancies that are stabilised by a splitting mechanism (Fig. 4a).34 The presence of these vacancies has been found to influence OER catalysis. It has been proposed that these defects change the OER reaction mechanism by providing new active sites as well as by lowering energy barrier for the first Proton Coupled Electron Transfer (PCET). This occurs because the vacancy-associated electrons are more readily available for transfer (Fig. 4b).35,50 Vacancies might also provide additional pathways via hydration, which further influence the reaction mechanism (Fig. 4c).35 In addition, oxygen depletion has been experimentally observed to increase the selectivity towards the oxygen evolution reaction, in detriment of the competing H2O2 formation.51 On the other hand, hydrogen peroxide production is predicted to be more favourable in vacancy-free (−111) surfaces.
It is important to note that the role of defects in catalysis is controversial and there are discrepancies between theoretical and experimental work. For example, in Fe2O3 photoanodes, DFT + U calculations have suggested that the potential determining step (PDS) during OER is reduced when increasing the density of oxygen vacancies compared to a pristine surface.52 However, kinetic analysis in Fe2O3 containing different vacancy concentrations have shown that the OER rate determining step (RDS) is chemical in nature and is independent of the vacancy concentration.53 Such discrepancy might be related to the chemical nature of the RDS54,55 or to the difficulty in experimentally separating the contributions of vacancies within a large surface. As discussed below, further studies integrating modelling and experiments, are needed to unambiguously expose the role of defects and disorder in the catalytic mechanisms.
Charge transport and accumulation
A further consequence of defect formation is the introduction of electronic charge into the lattice. For example, the formation of an oxygen vacancy results in the injection of two electrons into the structure. Such excess charge might minimise its energy by displacing the surrounding atoms forming a polaronic state and change the conductivity of the material. Polarons can also be formed upon photoexcitation.7,56–58 The tendency to form polarons depends on the atomic structure surrounding the charge and is, consequently, facet-dependent. Indeed, recent experiments have shown that the timescale of polaron generation at the bulk is different than at the surface, suggesting that the coordination environment is a critical parameter.59,60
Achieving high catalytic activities requires an efficient transport of photogenerated carriers to active sites and the accumulation of charges for a sufficient amount of time (i.e. beyond milliseconds to drive the OER).61 Photocatalytic oxides typically exhibit anisotropic charge transport – different crystal directions show different transport properties. Moreover, charge carriers often move via thermally activated hopping mechanisms, requiring geometric changes in the atomic structure for effective polaron motion. As expected for a polaronic system, in BiVO4 the activation barrier for electron (approx. 0.2–0.6 eV) and hole (approx. 0.4–0.8 eV) hopping are highly anisotropic leading to directional dependent mobility.37 A direct consequence of such anisotropic transport is a facet dependent charge accumulation: the (010) facet has been experimentally observed to favour electron accumulation while holes are more readily available at (110) terminations.39,42
Maximising charge transport and controlling the localisation and accumulation of long-lived charges is critical for photoelectrochemical reactions. For example, the rate determining step of the OER in Fe2O3 photoanodes has been measured to involve the diffusion of three oxidation equivalents to the active site, thus requiring an efficient transport mechanism.62,63 Similar observations have been made in other catalytic oxides, including BiVO4, yet the role of the exposed facet on the kinetics of the rate determining step is largely unknown.
Co-catalyst deposition and electrochemical double layer structure
Anisotropic accumulation of electrons and holes implies that some facets might be more likely to act as reduction or oxidation sites respectively. This has a direct consequence in co-catalyst deposition which normally involves the photo-reduction of ions into a metallic catalyst (e.g. Pt, Au) or the photo-oxidation of ions into oxides (e.g.: MnO2). Experimental studies with BiVO4 have found that metals selectively deposit on the (010) facet, while oxides are selectively formed at the (110) facet.39
The role of the selected facet in controlling the distribution of surface charge in solid electrodes has also an impact on how the electrochemical double layer (EDL) is established when the electrode interfaces a liquid electrolyte. In particular, different facets might exhibit a different potential of zero free charge (pzfc)—the potential at which the capacitive charge at the surface is zero.64 This is important because the pzfc dictates the strength of the interfacial electric filed, which depends on the difference between the pzfc and the applied potential.38 Consequently, the facet-dependent pzfc will influence the molecular structure of the electrode-electrolyte interface and impact the rate of charge transfer.
Furthermore, the different atomic arrangement on each facet influences the molecular arrangement of the electrolyte molecules. For instance, hematite immersed in water forms hydrogen bonds with its (012) and (110) facets, while its (001) facet reorders the water structure only weakly.65 Despite the recognised importance of understanding the role of the structure of the EDL on the electrochemical activity, theoretical and experimental assessments are scarce. Theoretical modelling of the atomic structure of the EDL requires molecular dynamics (MD) simulations with explicit inclusion of solvent molecules. Those can be done via parametrised force-fields,65 which typically lack transferability, or ab initio approaches.66,67 The latter are computationally expensive if one aims to reproduce long-range effects in the solvent but significant progress has been made over the last years.68,69
It is important to note that, to date, experimental studies are often limited to metallic surfaces. While in semiconductor catalysts the potential drop occurs primarily within the solid, forming a space charge layer,70,71 the ability to charge the surface is likely to impact the molecular structure of the electrolyte and consequently impact reactivity. To date, this is an uncharted territory.
Determining the best facet for photocatalysis is particularly difficult as many processes, ranging from light absorption to the desorption of reaction products, should synchronise. Therefore, the optimal facet might not be the one that is most reactive, or more prone to form active defects, but the one that best balances the different steps involved in the photocatalytic mechanisms. Addressing such complexity necessarily requires a holistic approach to facet engineering involving in situ characterisation and theory guidance.
Facet stability and control
Thermodynamic stability and surface reconstructions
The dynamic rearrangement of materials under photoelectrochemical conditions is a largely unexplored area. To a first approximation, the driving force behind these rearrangements is the maximisation of the crystal packing and the atomic coordination once the solid is in contact with the electrolyte. However, photo-electrosynthesis is a dynamic process and the electrochemical conditions (pH, ionic strength…) might vary during the reaction inducing structural transformations in the electrode. For example, during a reaction, a pH gradient might develop rendering a specific crystal phase unstable and trigger a solid—solid phase transition.
Recent work has stated to explore the correlation between the reconstruction of a specific facet and catalytic selectivity. These studies have primarily been performed in well-defined metallic surfaces. For example, operando AFM has been used to observe changes in the surface morphology of a Cu(100) cathode for CO2 reduction as a function of potential.18 The authors proposed a correlation between the reconstructed surface at different potentials with CO2 reduction intermediates, such as CO species, in order to explain the bias dependence of the product selectivity.
Reconstructions have also been proposed to be an integral part of the catalytic cycle.7,72–74 For example, in oxides like IrO2 and Co2O3 used for the OER, isotopic labelling studies have shown that some of the O2 formed contains lattice oxygen atoms.75,76 Similar observations have been made for TiO2 both for the OER and the oxidation of organic compounds such as benzene.77–79 In contrast, NiFeOxHy surfaces have been shown not to lose lattice oxygen during the OER but Fe is incorporated from the electrolyte.80–82 The reactivity and the tendency to undergo reconstructions will depend on the crystal facet and the specific terminations, for example, hydroxylated disordered structures are known to work better than the organised rutile terminations.
The preferential dissolution of the different elements in the salts under the electrolyte potential and photocatalytic conditions is a major issue which is poorly explored due to its complexity. Yet, indications of leaching of cations (under positive potentials) and anions (at negative ones) start to be gathered.83,84 The ultimate consequence of such leaching is that surface disorder appears, leading to atom-enriched surfaces, new structural motifs, and disorder in the packaging of polyhedral subunits or the materials themselves. Disorder allows for a fine adjustment of the electronic levels that can be accommodated by the extra degrees of freedom on the surface of the material.
Synthetic manipulation of facets
The ultimate goal of manipulating the crystal structure through the control of the exposed facet is to achieve a highly reactive surface. However, as with the reconstructions, very reactive facets usually rapidly disappear during the crystal growth process while facets with lower surface energy grow slower, giving rise to the final shape of the crystal. As a consequence, special synthetic methods are required to stabilise reactive terminations such as selective etching, kinetic and thermodynamic control. One powerful method explored to prepare catalysts is to use organic and inorganic additives as Morphology Controlling Agents (MCAs), which act as selective surface stabilisers during synthesis. MCAs adsorb on specific terminations reducing the surface energy and deviating the crystal growth from its thermodynamically preferred path.14,85,86 Typical MCAs are: Poly Vinyl Pyrrolidone (PVP), Cetyl Trimethyl Ammonium Bromide (CTAB), Sodium Dodecyl Benzene Sulfonate (SDBS), stearic acid, oleic acid and potassium iodide (KI). Importantly, these stabilisers must be removed to obtain a clean surface before the solid can be used in photoelectrocatalytic applications. While facet control in metal oxides is a challenging topic, there have been important synthetic advances and we refer the readers to reviews on this topic.87,88
Challenges and outlook
Since the pioneering work by Fujishima and Honda in the early 70s, photo(electro)chemistry has made great strides thanks to synthetic advances powered by better mechanistic understanding. Such has been the success that, in many cases, the technology is becoming commercially viable. Given the demand for ever more complex chemicals, the next step in the design of photoelectrocatalytic materials is, unavoidably, going to involve the fine tuning of the reactive surface in order to drive selective multi-electron chemistry (e.g. CO2 reduction into targeted C2+ products).
Such control will require better understanding of how the electrochemical surface evolves during catalysis and identifying how to prepare not the pristine solid, but the active catalyst. In this context, recent technological advances are opening the door to operando and in situ studies.89–91 This includes exciting opportunities in microscopies, such as transmission electron and atomic force microscopies (TEM and AFM, respectively), as well as spectroscopies, such as IR, Raman and X-ray absorption, photoelectron and emission spectroscopies (XAS, XPS and XES, respectively).92–96
Experimentally, one challenge that lies ahead of performing these advanced operando studies, will be to establish whether the observed surface changes result from the catalytic reaction or the measurement itself. For example, it has recently been shown that a chemical segregation of Bi in BiVO4 crystals takes place during TEM measurements forming Bi nanoparticles on the photoanode surface.97 Indications seem to point out towards a preferential dissolution of vanadyl species (Bi presenting a lower detachment rate). Accounting for these processes and transformations is crucial to understand the real surface structure that determines the structure-activity relationships governing the photoelectrocatalytic process. Such mechanistic understanding is only nowadays beginning to be experimentally probed and introduced in the simulations.98,99
Beyond the monitoring of the surface, efficient catalyst development requires identifying how the surface changes correlate with reactivity and selectivity. This will entail the further coupling of optical spectroscopy and microscopy with product detection methods such as Inductively Coupled Plasma Mass Spectrometry (ICP-MS), Differential Electrochemical Mass Spectrometry (DEMS) or on-line Electrochemical Mass Spectrometry (EC-MS) as well as the close integration of characterisation techniques with first principles modelling.
From a theoretical viewpoint, to understand how disorder and facet changes influence reaction mechanisms, product distributions and reaction selectivity, it is going to be crucial to establish how the reconstructions of the solid during catalysis change the electronic structures and surface motifs. This will require modelling at different time and length scales as well as establishing the origin and control of the preferential dissolution. Importantly, it will also demand explicitly introducing disorder in the simulations;100 this is essential to provide meaningful structure-activity relationships, since lowering the symmetry of the materials can break linear-scaling relationships that limit the activity or selectivity in catalysed processes.101 Despite the complexity of the photoelectrocatalytic process, the recent advances in synthesis, characterisation, and simulations will ensure an exciting and thriving future for photo-electrosynthesis research and development.
Note
Information about facets and more generally about crystallography can be found in these useful sites: (i) https://www.its.caltech.edu/~atomic/snowcrystals/faceting/faceting.htm (ii) https://sethna.lassp.cornell.edu/CrystalShapes/Equilibrium_Crystal_Shapes.html (iii) https://www.xtal.iqfr.csic.es/Cristalografia/index-en.html.
Conflicts of interest
There are no conflicts to declare.
Acknowledgements
The project that gave rise to these results received the support of a fellowship from “la Caixa” Foundation (ID 100010434). The fellowship code is LCF/BQ/PR22/11920013. S. G. thanks the support from project PID2020-116093RB-C41 funded by MCIN/AEI/10.13039/501100011033/ and Prometeo Grant (No. PROMETEU/2020/028) funded by Generalitat Valenciana. C. A. M. and S. G. acknowledge Generalitat Valenciana for the postdoctoral fellowship APOSTD/2021/251 and University Jaume I through project UJI-B2020-50 and postdoc fellowship POSDOC/2019/20. C. A. M. also thanks MinCiencias Colombia through the Fondo Nacional de financiamiento para la ciencia, la tecnología y la innovación “Francisco José de Caldas”, call 848-2019 for funding. S. G., A. G. B. and L. M. acknowledge the financial support from the European Commission associated to the European project “SUN2CHEM”, through the Grant Agreement 884444.
References
- R. J. Detz, J. N. H. Reek and B. C. C. van der Zwaan, The future of solar fuels: when could they become competitive?, Energy Environ. Sci., 2018, 11, 1653–1669 RSC.
- A. J. Bard and M. A. Fox, Artificial Photosynthesis: Solar Splitting of Water to Hydrogen and Oxygen, Acc. Chem. Res., 1995, 28, 141–145 CrossRef CAS.
- M. G. Walter,
et al., Solar Water Splitting Cells, Chem. Rev., 2010, 110, 6446–6473 CrossRef CAS PubMed.
- M. Dumortier, S. Tembhurne and S. Haussener, Holistic design guidelines for solar hydrogen production by photo-electrochemical routes, Energy Environ. Sci., 2015, 8, 3614–3628 RSC.
- V. Andrei, B. Reuillard and E. Reisner, Bias-free solar syngas production by integrating a molecular cobalt catalyst with perovskite–BiVO4 tandems, Nat. Mater., 2020, 19, 189–194 CrossRef CAS.
- M. T. Spitler,
et al., Practical challenges in the development of photoelectrochemical solar fuels production, Sustainable Energy Fuels, 2020, 4, 985–995 RSC.
- E. Pastor,
et al., Electronic defects in metal oxide photocatalysts, Nat. Rev. Mater., 2022, 7, 503–521 CrossRef CAS.
- J. Bisquert, P. Cendula, L. Bertoluzzi and S. Gimenez, Energy Diagram of Semiconductor/Electrolyte Junctions, J. Phys. Chem. Lett., 2014, 5, 205–207 CrossRef CAS PubMed.
- K. Sivula and R. van de Krol, Semiconducting materials for photoelectrochemical energy conversion, Nat. Rev. Mater., 2016, 1, 15010 CrossRef CAS.
- W. A. Smith, I. D. Sharp, N. C. Strandwitz and J. Bisquert, Interfacial band-edge energetics for solar fuels production, Energy Environ. Sci., 2015, 8, 2851–2862 RSC.
- I. Y. Ahmet,
et al., Demonstration of a 50 cm2 BiVO4 tandem photoelectrochemical-photovoltaic water splitting device, Sustainable Energy Fuels, 2019, 3, 2366–2379 RSC.
- G. D. Barmparis, Z. Lodziana, N. Lopez and I. N. Remediakis, Nanoparticle shapes by using Wulff constructions and first-principles calculations, Beilstein J. Nanotechnol., 2015, 6, 361–368 CrossRef PubMed.
- Z.-Y. Zhou, N. Tian, J.-T. Li, I. Broadwell and S.-G. Sun, Nanomaterials of high surface energy with exceptional properties in catalysis and energy storage, Chem. Soc. Rev., 2011, 40, 4167 RSC.
- Y. Shang and L. Guo, Facet-Controlled Synthetic Strategy of Cu2O-Based Crystals for Catalysis and Sensing, Adv. Sci., 2015, 2, 1500140 CrossRef PubMed.
- Y. Li,
et al., Recent Progress on Surface Reconstruction of Earth–Abundant Electrocatalysts for Water Oxidation, Small, 2019, 15, 1901980 CrossRef.
- S. Chen, L. Ma, Z. Huang, G. Liang and C. Zhi, In situ/operando analysis of surface reconstruction of transition metal-based oxygen evolution electrocatalysts, Cell Rep. Phys. Sci., 2022, 3, 100729 CrossRef CAS.
- Z. Kou,
et al., Dynamic Surface Chemistry of Catalysts in Oxygen Evolution Reaction, Small Sci., 2021, 1, 2100011 CrossRef.
- G. H. Simon, C. S. Kley and B. Roldan Cuenya, Potential-Dependent Morphology of Copper Catalysts During CO2 Electroreduction Revealed by In Situ Atomic Force Microscopy, Angew. Chem., Int. Ed., 2021, 60, 2561–2568 CrossRef CAS PubMed.
- S. H. Lee,
et al., Oxidation State and Surface Reconstruction of Cu under CO2 Reduction Conditions from In Situ X-ray Characterization, J. Am. Chem. Soc., 2021, 143, 588–592 CrossRef CAS PubMed.
- M. Regue,
et al., TiO2 photoanodes with exposed {0 1 0} facets grown by aerosol-assisted chemical vapor deposition of a titanium oxo/alkoxy cluster, J. Mater. Chem. A, 2019, 7, 19161–19172 RSC.
- H. S. Han,
et al., (0 2 0)-Textured tungsten trioxide nanostructure with enhanced photoelectrochemical activity, J. Catal., 2020, 389, 328–336 CrossRef CAS.
- G. Zheng,
et al., Sandwich structured WO3 nanoplatelets for highly efficient photoelectrochemical water splitting, J. Mater. Chem. A, 2019, 7, 26077–26088 RSC.
- J. Ma,
et al., Efficient Photoelectrochemical Conversion of Methane into Ethylene Glycol by WO3 Nanobar Arrays, Angew. Chem., 2021, 133, 9443–9447 CrossRef.
- Y. Pihosh,
et al., Photocatalytic generation of hydrogen by core-shell WO3/BiVO4 nanorods with ultimate water splitting efficiency, Sci. Rep., 2015, 5, 1–2 Search PubMed.
- T. W. Kim and K.-S. Choi, Nanoporous BiVO4 Photoanodes with Dual-Layer Oxygen Evolution Catalysts for Solar Water Splitting, Science, 2014, 343, 990–994 CrossRef CAS.
- S. Ho-Kimura, W. Soontornchaiyakul, Y. Yamaguchi and A. Kudo, Preparation of Nanoparticle Porous-Structured BiVO4 Photoanodes by a New Two-Step Electrochemical Deposition Method for Water Splitting, Catalysts, 2021, 11, 136 CrossRef CAS.
- K. Zhang,
et al., Tuning Selectivity of Photoelectrochemical Water Oxidation via Facet-Engineered Interfacial Energetics, ACS Energy Lett., 2021, 6, 4071–4078 CrossRef CAS.
-
R. Memming, Semiconductor Electrochemistry, WILEY-VCH Verlag GmbH, 2000 Search PubMed.
- D. Wang,
et al., Crystal Facet Dependence of Water Oxidation on BiVO4 Sheets under Visible Light Irradiation, Chem. – Eur. J., 2011, 17, 1275–1282 CrossRef CAS PubMed.
- S. Chen,
et al., Facet-Engineered Surface and Interface Design of Monoclinic Scheelite Bismuth Vanadate for Enhanced Photocatalytic Performance, ACS Catal., 2020, 10, 1024–1059 CrossRef CAS.
- S. Lardhi, L. Cavallo and M. Harb, Significant Impact of Exposed Facets on the BiVO4 Material Performance for Photocatalytic Water Splitting Reactions, J. Phys. Chem. Lett., 2020, 11, 5497–5503 CrossRef CAS.
- M. Harb, G. Jeantelot and J.-M. Basset, Insights into the Most Suitable TiO2 Surfaces for Photocatalytic O2 and H2 Evolution Reactions from DFT Calculations, J. Phys. Chem. C, 2019, 123, 28210–28218 CrossRef CAS.
- J. Hu, W. Chen, X. Zhao, H. Su and Z. Chen, Anisotropic Electronic Characteristics, Adsorption, and Stability of Low-Index BiVO4 Surfaces for Photoelectrochemical Applications, ACS Appl. Mater. Interfaces, 2018, 10, 5475–5484 CrossRef CAS PubMed.
- F. S. Hegner, D. Forrer, J. R. Galán-Mascarós, N. López and A. Selloni, Versatile Nature of Oxygen Vacancies in Bismuth Vanadate Bulk and (001) Surface, J. Phys. Chem. Lett., 2019, 10, 6672–6678 CrossRef CAS PubMed.
- P. Nikačević, F. S. Hegner, J. R. Galán-Mascarós and N. López, Influence of Oxygen Vacancies and Surface Facets on Water Oxidation Selectivity toward Oxygen or Hydrogen Peroxide with BiVO4, ACS Catal., 2021, 11, 13416–13422 CrossRef.
- C. Y. Toe,
et al., Facet-dependent carrier dynamics of cuprous oxide regulating the photocatalytic hydrogen generation, Mater. Adv., 2022, 3, 2200–2212 RSC.
- T. Liu, X. Zhou, M. Dupuis and C. Li, The nature of photogenerated charge separation among different crystal facets of BiVO4 studied by density functional theory, Phys. Chem. Chem. Phys., 2015, 17, 23503–23510 RSC.
- P. Sebastián-Pascual, Y. Shao-Horn and M. Escudero-Escribano, Toward understanding the role of the electric double layer structure and electrolyte effects on well-defined interfaces for electrocatalysis, Curr. Opin. Electrochem., 2022, 32, 100918 CrossRef.
- R. Li,
et al., Spatial separation of photogenerated electrons and holes among {010} and {110} crystal facets of BiVO4, Nat. Commun., 2013, 4, 1432 CrossRef PubMed.
- G.-L. Li, First-principles investigation of the surface properties of fergusonite-type monoclinic BiVO4 photocatalyst, RSC Adv., 2017, 7, 9130–9140 RSC.
- M. Favaro,
et al., Chemical, Structural, and Electronic Characterization of the (010) Surface of Single Crystalline Bismuth Vanadate, J. Phys. Chem. C, 2019, 123, 8347–8359 CrossRef CAS.
- H. L. Tan, X. Wen, R. Amal and Y. H. Ng, BiVO4 {010} and {110} Relative Exposure Extent: Governing Factor of Surface Charge Population and Photocatalytic Activity, J. Phys. Chem. Lett., 2016, 7, 1400–1405 CrossRef CAS.
- L. Zhang, D. Chen and X. Jiao, Monoclinic Structured BiVO4 Nanosheets: Hydrothermal Preparation, Formation Mechanism, and Coloristic and Photocatalytic Properties, J. Phys. Chem. B, 2006, 110, 2668–2673 CrossRef CAS.
- Z. Zhao, Z. Li and Z. Zou, Structure and energetics of low-index stoichiometric monoclinic clinobisvanite BiVO4 surfaces, RSC Adv., 2011, 1, 874 RSC.
- M. Sachs,
et al., Effect
of oxygen deficiency on the excited state kinetics of WO3 and implications for photocatalysis, Chem. Sci., 2019, 10, 5667–5677 RSC.
- S. Selim,
et al., Impact of Oxygen Vacancy Occupancy on Charge Carrier Dynamics in BiVO4 Photoanodes, J. Am. Chem. Soc., 2019, 141, 18791–18798 CrossRef CAS PubMed.
- R. Fernández-Climent, S. Giménez and M. García-Tecedor, The role of oxygen vacancies in water splitting photoanodes, Sustainable Energy Fuels, 2020, 4, 5916–5926 RSC.
- W. Wang,
et al., The Role of Surface Oxygen Vacancies in BiVO 4, Chem. Mater., 2020, 32, 2899–2909 CrossRef CAS.
- H. Seo, Y. Ping and G. Galli, Role of Point Defects in Enhancing the Conductivity of BiVO 4, Chem. Mater., 2018, 30, 7793–7802 CrossRef CAS.
- J. Hu, X. Zhao, W. Chen, H. Su and Z. Chen, Theoretical Insight into the Mechanism of Photoelectrochemical Oxygen Evolution Reaction on BiVO4 Anode with Oxygen Vacancy, J. Phys. Chem. C, 2017, 121, 18702–18709 CrossRef CAS.
- L. Wang,
et al., Suppressing Water Dissociation via Control of Intrinsic Oxygen Defects for Awakening Solar H2O-to-H2O2 Generation, Small, 2021, 17, 2100400 CrossRef CAS.
- X. Zhang, P. Klaver, R. van Santen, M. C. M. van de Sanden and A. Bieberle-Hütter, Oxygen Evolution at Hematite Surfaces: The Impact of Structure and Oxygen Vacancies on Lowering the Overpotential, J. Phys. Chem. C, 2016, 120, 18201–18208 CrossRef CAS.
- C. A. Mesa,
et al., Impact of the Synthesis Route on the Water Oxidation Kinetics of Hematite Photoanodes, J. Phys. Chem. Lett., 2020, 11, 7285–7290 CrossRef CAS PubMed.
- R. R. Rao,
et al., Spectroelectrochemical Analysis of the Water Oxidation Mechanism on Doped Nickel Oxides, J. Am. Chem. Soc., 2022, 144, 7622–7633 CrossRef CAS PubMed.
- S. W. Boettcher and Y. Surendranath, Heterogeneous electrocatalysis goes chemical, Nat. Catal., 2021, 4, 4–5 CrossRef CAS.
- E. Pastor,
et al., In situ observation of picosecond polaron self-localisation in α-Fe2O3 photoelectrochemical cells, Nat. Commun., 2019, 10, 3962 CrossRef PubMed.
- L. M. Carneiro,
et al., Excitation-wavelength-dependent small polaron trapping of photoexcited carriers in α-Fe2O3, Nat. Mater., 2017, 16, 819–825 CrossRef CAS PubMed.
- S. Biswas, J. Husek, S. Londo and L. R. Baker, Highly Localized Charge Transfer Excitons in Metal Oxide Semiconductors, Nano Lett., 2018, 18, 1228–1233 CrossRef CAS PubMed.
- S. Biswas, J. Husek and L. R. Baker, Elucidating ultrafast electron dynamics at surfaces using extreme ultraviolet (XUV) reflection–absorption spectroscopy, Chem. Commun., 2018, 54, 4216–4230 RSC.
- S. Biswas, S. Wallentine, S. Bandaranayake and L. R. Baker, Controlling polaron formation at hematite surfaces by molecular functionalization probed by XUV reflection-absorption spectroscopy, J. Chem. Phys., 2019, 151, 104701 CrossRef.
- R. Steinitz-Eliyahu,
et al., Mixed excitonic nature in water-oxidized BiVO4 surfaces with defects, Phys. Rev. Mater., 2022, 6, 065402 CrossRef CAS.
- C. A. Mesa,
et al., Multihole water oxidation catalysis on haematite photoanodes revealed by operando spectroelectrochemistry and DFT, Nat. Chem., 2020, 12, 82–89 CrossRef CAS PubMed.
- F. le Formal,
et al., Rate Law Analysis of Water Oxidation on a Hematite Surface, J. Am. Chem. Soc., 2015, 137, 6629–6637 CrossRef CAS.
- A. Auer, X. Ding, A. S. Bandarenka and J. Kunze-Liebhäuser, The Potential of Zero Charge and the Electrochemical Interface Structure of Cu(111) in Alkaline Solutions, J. Phys. Chem. C, 2021, 125, 5020–5028 CrossRef CAS PubMed.
- S. Kerisit, Water structure at hematite–water interfaces, Geochim. Cosmochim. Acta, 2011, 75, 2043–2061 CrossRef CAS.
- B. C. Wood, E. Schwegler, W. I. Choi and T. Ogitsu, Hydrogen-Bond Dynamics of Water at the Interface with InP/GaP(001) and the Implications for Photoelectrochemistry, J. Am. Chem. Soc., 2013, 135, 15774–15783 CrossRef CAS PubMed.
- T. A. Pham, Y. Ping and G. Galli, Modelling heterogeneous interfaces for solar water splitting, Nat. Mater., 2017, 16, 401–408 CrossRef CAS PubMed.
- O. Björneholm,
et al., Water at Interfaces, Chem. Rev., 2016, 116, 7698–7726 CrossRef.
- X. Zhou and H. Dong, A Theoretical Perspective on Charge Separation and Transfer in Metal Oxide Photocatalysts for Water Splitting, ChemCatChem, 2019, 11, 3688–3715 CrossRef CAS.
- S. Corby,
et al., Charge Separation, Band-Bending, and Recombination in WO3 Photoanodes, J. Phys. Chem. Lett., 2019, 10, 5395–5401 CrossRef CAS.
-
L. M. Peter, Chapter 1. Photoelectrochemistry: From Basic Principles to Photocatalysis, in Photocatalysis: Fundamentals and Perspectives, 2016, pp. 1–28. 10.1039/9781782622338-00001.
- A. Grimaud, W. T. Hong, Y. Shao-Horn and J.-M. Tarascon, Anionic redox processes for electrochemical devices, Nat. Mater., 2016, 15, 121–126 CrossRef CAS PubMed.
- A. Grimaud,
et al., Activation of surface oxygen sites on an iridium-based model catalyst for the oxygen evolution reaction, Nat. Energy, 2017, 2, 16189 CrossRef CAS.
- A. Grimaud,
et al., Activating lattice oxygen redox reactions in metal oxides to catalyse oxygen evolution, Nat. Chem., 2017, 9, 457–465 CrossRef CAS PubMed.
- H. M. A. Amin and H. Baltruschat, How many surface atoms in Co3O4 take part in oxygen evolution? Isotope labeling together with differential electrochemical mass spectrometry, Phys. Chem. Chem. Phys., 2017, 19, 25527–25536 RSC.
- S. Fierro, T. Nagel, H. Baltruschat and C. Comninellis, Investigation of the oxygen evolution reaction on Ti/IrO2 electrodes using isotope labelling and on-line mass spectrometry, Electrochem. Commun., 2007, 9, 1969–1974 CrossRef CAS.
- S. Melchers, J. Schneider and D. W. Bahnemann, Isotopic studies on the degradation of acetaldehyde on anatase surfaces, Catal. Today, 2020, 340, 318–322 CrossRef CAS.
- C. Günnemann, D. W. Bahnemann and P. K. J. Robertson, Isotope Effects in Photocatalysis: An Underexplored Issue, ACS Omega, 2021, 6, 11113–11121 CrossRef PubMed.
- J. F. Montoya,
et al., Catalytic Role of Surface Oxygens in TiO2 Photooxidation Reactions: Aqueous Benzene Photooxidation with Ti18O2 under Anaerobic Conditions, J. Phys. Chem. Lett., 2013, 4, 1415–1422 CrossRef CAS PubMed.
- Y. Zhou and N. López, The Role of Fe Species on NiOOH in Oxygen Evolution Reactions, ACS Catal., 2020, 10, 6254–6261 CrossRef CAS.
- C. Roy,
et al., Impact of nanoparticle size and lattice oxygen on water oxidation on NiFeOxHy, Nat. Catal., 2018, 1, 820–829 CrossRef CAS.
- L. Trotochaud, S. L. Young, J. K. Ranney and S. W. Boettcher, Nickel–Iron Oxyhydroxide Oxygen-Evolution Electrocatalysts: The Role of Intentional and Incidental Iron Incorporation, J. Am. Chem. Soc., 2014, 136, 6744–6753 CrossRef CAS PubMed.
- B. Zhang,
et al., Homogeneously dispersed multimetal oxygen-evolving catalysts, Science, 2016, 352, 333–337 CrossRef CAS PubMed.
- A. Venugopal, R. Kas, K. Hau and W. A. Smith, Operando Infrared Spectroscopy Reveals the Dynamic Nature of Semiconductor–Electrolyte Interface in Multinary Metal Oxide Photoelectrodes, J. Am. Chem. Soc., 2021, 143, 18581–18591 CrossRef CAS PubMed.
- H. G. Yang,
et al., Anatase TiO2 single crystals with a large percentage of reactive facets, Nature, 2008, 453, 638–641 CrossRef CAS PubMed.
- L. Montañés,
et al., Facile Surfactant-Assisted Synthesis of BiVO4 Nanoparticulate Films for Solar Water Splitting, Catalysts, 2021, 11, 1244 CrossRef.
- R. A. Rather,
et al., Influence of exposed facets, morphology and hetero-interfaces of BiVO4 on photocatalytic water oxidation: A review, Int. J. Hydrogen Energy, 2021, 46, 21866–21888 CrossRef CAS.
- S. Wang, G. Liu and L. Wang, Crystal Facet Engineering of Photoelectrodes for Photoelectrochemical Water Splitting, Chem. Rev., 2019, 119, 5192–5247 CrossRef CAS PubMed.
- D. Yao,
et al., The Controllable Reconstruction of Bi–MOFs for Electrochemical CO2 Reduction through Electrolyte and Potential Mediation, Angew. Chem., Int. Ed., 2021, 60, 18178–18184 CrossRef CAS PubMed.
- W. T. Osowiecki,
et al., Factors and Dynamics of Cu Nanocrystal Reconstruction under CO2 Reduction, ACS Appl. Energy Mater., 2019, 2, 7744–7749 CrossRef CAS.
- C. A. Mesa, E. Pastor and L. Francàs, UV–Vis
operando spectroelectrochemistry for (photo)electrocatalysis: Principles and guidelines, Curr. Opin. Electrochem., 2022, 35, 101098 CrossRef CAS.
- J. Timoshenko and B. Roldan Cuenya, In Situ/Operando Electrocatalyst Characterization by X-ray Absorption Spectroscopy, Chem. Rev., 2021, 121, 882–961 CrossRef CAS PubMed.
- L. C. Pardo Pérez,
et al., Determining Structure–Activity Relationships in Oxide Derived Cu−Sn Catalysts During CO2 Electroreduction Using X–Ray Spectroscopy, Adv. Energy Mater., 2022, 12, 2103328 CrossRef.
- J. Li and J. Gong, Operando characterization techniques for electrocatalysis, Energy Environ. Sci., 2020, 13, 3748–3779 RSC.
- J. Bai,
et al., Molybdenum–Promoted Surface Reconstruction in Polymorphic Cobalt for Initiating Rapid Oxygen Evolution, Adv. Energy Mater., 2022, 12, 2103247 CrossRef CAS.
- D. Grumelli,
et al., Electrochemical Stability of the Reconstructed Fe3O4 (001) Surface, Angew. Chem., Int. Ed., 2020, 59, 21904–21908 CrossRef CAS.
- R. Arcas,
et al., Direct Observation of the Chemical Transformations in BiVO4 Photoanodes upon Prolonged Light–Aging Treatments, Sol. RRL, 2022, 2200132 CrossRef CAS.
- F. Dattila, R. García-Muelas and N. López, Active and Selective Ensembles in Oxide-Derived Copper Catalysts for CO2 Reduction, ACS Energy Lett., 2020, 5, 3176–3184 CrossRef CAS.
- A. S. Raman, R. Patel and A. Vojvodic, Surface stability of perovskite oxides under OER operating conditions: a first principles approach, Faraday Discuss., 2021, 229, 75–88 RSC.
- M. Capdevila-Cortada and N. López, Entropic contributions enhance polarity compensation for CeO2(100) surfaces, Nat. Mater., 2017, 16, 328–334 CrossRef CAS PubMed.
- J. Pérez-Ramírez and N. López, Strategies to break linear scaling relationships, Nat. Catal., 2019, 2, 971–976 CrossRef.
|
This journal is © The Royal Society of Chemistry 2022 |