DOI:
10.1039/D1AN01934A
(Paper)
Analyst, 2022,
147, 80-86
Biomimetic -mineralized multifunctional nanoflowers for anodic-stripping voltammetric immunoassay of rehabilitation-related proteins
Received
26th October 2021
, Accepted 14th November 2021
First published on 15th November 2021
Abstract
C-reactive proteins (CRPs; an acute-phase protein) in patients with initial acute cerebral infarction neurological rehabilitation prediction have a significant correlation. In this work, a simple and sensitive anodic-stripping voltammetric (ASV) immunosensing system was innovatively designed for the quantitative screening of target CRPs using biomimetic-mineralized bifunctional antibody-Cu3(PO4)2 nanoflowers as molecular tags. In this system, a monoclonal anti-CRP antibody-anchored microtiter plate was utilized to specifically capture target CRPs from the sample. For detection, a sandwiched immunoreaction mode was employed with the antibody-Cu3(PO4)2 nanoflowers in the presence of analytes. Subsequent ASV measurement of copper ions (Cu2+) released under acidic conditions from the bifunctional nanoflowers was conducted at an in situ prepared mercury film electrode. The introduction of hybrid nanoflowers greatly increased the loading amount of copper ions on the molecular tag, thereby amplifying the detectable signal of electrochemical immunoassay. Meanwhile, factors influencing the analytical properties of the electrochemical immunoassay were investigated in detail. By combining the high-efficiency nanohybrids with signal amplification, the dynamic concentration range of electrochemical immunoassay spanned from 0.01 ng mL−1 to 100 ng mL−1 toward the target CRP. The limit of detection was calculated to be 0.0079 ng mL−1 at 3Sblank criterion. Intra- and interassay imprecisions (relative standard deviations: RSDs) were less than or equal to 6.72%. Good anti-interference ability, long-term storage stability, and acceptable accuracy for the evaluation of human serum specimens were observed during a series of procedures to determine the target protein. In addition, the bifunctional nanoflower-based immunosensing system offers promise for the simple, cost-effective analysis of disease-related proteins.
Introduction
C-reactive proteins (CRPs) are acute-phase proteins of hepatic origin whose levels have positive correlation with poor outcomes in patients with acute coronary syndrome, regardless of the etiology (infection, trauma and inflammation).1 One of the major functions of CRP is to recognize foreign pathogens and phospholipid components of damaged cells, resulting in the activation of the classical complement pathway.2 Upon arrival to a medical facility, initial peak CRP levels are not associated with treatment failure and 28-day mortality but when used in combination with other biomarkers or APACHE II score, CRP has higher predictability for mortality in sepsis scores.3 The measurement of serum CRP concentration may be helpful in patients with CSF findings consistent with meningitis, with a negative Gram's stain result such that the physician considers withholding antimicrobial therapy.4 In this regard, CRP is perhaps the most important marker of inflammation and is analyzed routinely in clinical practice.
Recently, different methods and strategies have been utilized for monitoring serous CRP samples,5 such as bioresponsive hydrogel-based surface relief diffraction gratings,6 peroxidase-mimicking nanozymes with surface-dispersed Pt atoms for the colorimetric lateral flow immunoassay,7 electrochemiluminescence-incorporated lateral flow immunoassays,8 tandem giant magneto-resistance biosensors,9 and impedimetric immunosensors.10 Among these systems, electrochemical immunosensing probes have gained gradually increasing interests due to their combined advantages of high-sensitivity electrochemistry and specific antigen–antibody immunoreactions.11,12 However, a key issue for the development of high-efficiency electrochemical immunoassays is to achieve a strongly detectable electrochemical signal because most antigen–antibody reactions cannot produce an electrical signal. Enzyme labels and nano labels are widely used for this purpose.13,14 Relative to enzyme labels, the rapidly emerging research field of bio-nano labels opens up new possibilities for bioanalytical applications.15 One major advantage of using nano labels is that one can control and tailor their properties in a very predictable manner to meet the needs of specific applications.16
Typically, nano labels involve inorganic nano labels, organic nano labels, and organic–inorganic hybrid nano labels.17 For example, Yu et al. used platinum or gold nanoparticle–labeled secondary antibodies for the design of immunoassays, respectively.18,19 The biomolecules were physically adsorbed onto the surface of nanoparticles. One basic disadvantage of using adsorption is the instability of antibody conjugation during the measurement.20 Moreover, it was susceptible to the ionic strength of the test solution. In contrast, Sun et al. synthesized cross-linked urease nanoparticles for the labeling of secondary antibodies to determine lipocalin-2 via carbodiimide coupling.21 Unfavorably, one of the problems commonly associated with covalent conjugation is the decrease in biomolecular activity upon exposure to reactive groups under harsh reaction conditions.22,23 Interestingly, the emergence of organic–inorganic hybrid nanosystems opens a new horizon of the use of nanomaterial labels.24 At this point, organic–inorganic hybrid materials enable the integration of useful organic and inorganic characteristics within a single molecular-scale composite, e.g., unique electronic and optical properties for this promising class of materials. Various metal–organic frameworks, e.g., metal-polydopamine frameworks25 and zeolitic imidazolate frameworks,26 have been utilized as nano labels for the immunoassay development. However, most biomolecules such as antibodies and enzymes were covalently conjugated or physically adsorbed onto the metal–organic frameworks. To improve this issue, the motivation of our work is to explore a new organic–inorganic nano label for the development of electrochemical immunoassays.
In this work, we prepared a new electrochemical signal-generation tag based on biomimetic-mineralized bifunctional antibody-Cu3(PO4)2 nanoflowers for the anodic-stripping voltammetric detection of CRP (Scheme 1). The antibody-Cu3(PO4)2 nanoflowers were synthesized by a facile and mild biomimetic-mineralizing process. The synthesized organic–inorganic nanotags are not only used to capture the target CRP in the sample, but also used as signal probes for electrochemical measurement via the released copper ions from the nanoflowers under acidic conditions. The use of antibody-Cu3(PO4)2 nanoflowers is expected to enhance the loading amount of copper ions, thus amplifying the voltammetric signal of electrochemical immunoassays with a sandwiched reaction mode on the capture antibody-coated microtiter plate. The major objective of this study is to construct an enzyme-free nano labelling probe with the bioactivity for the detection of disease-related biomolecules.
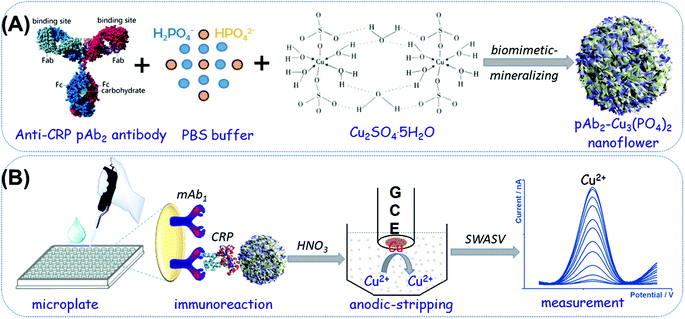 |
| Scheme 1 Schematic representation of the biomimetic-mineralized-functionalized nanoflowers for the anodic-stripping voltammetric immunoassay of the C-reactive protein (CRP): (A) synthesis process of anti-CRP pAb2 antibody-Cu3(PO4)2 hybrid nanoflowers; (B) immunoreaction procedure and square-wave anodic-stripping voltammetric (SWASV) measurement on an in situ mercury drop glassy carbon electrode (GCE) (note: mAb1: rabbit anti-CRP monoclonal capture antibody; pAb2: rabbit anti-CRP polyclonal detection antibody). | |
Experimental section
Chemicals and reagents
Rabbit monoclonal [EPR22862-10] antibodies to C-reactive protein (abbreviation: mAb1; application: IHC-P and WB; reactivity: human; conjugate: unconjugated; Cat# no.: ab256492), rabbit polyclonal antibodies to C-reactive protein (abbreviation: pAb2; application: IHC-P and WB; reactivity: human and rat; conjugate: unconjugated; Cat# no.: ab227507), and a human CRP ELISA kit (C-reactive protein) (one-wash 90 min protocol; sensitivity: 1.23 pg mL−1; range: 1.95–8000 pg mL−1; sample type: cell culture supernatant, Cit plasma, EDTA plasma, Hep plasma, serum; detection method: fluorescent; assay type: sandwiched; reacts with: human) were purchased from Abcam (Shanghai, China). All high-binding polystyrene microplates were acquired from Greiner (Product no.: 655061, Frickenhausen, Germany). All other chemicals including bovine serum albumin (BSA, refined grade, ≥98.0%), CuSO4·5H2O and phosphate-buffered saline (PBS) solutions with different pH values were obtained from Aladdin (Shanghai, China). All other reagents were of analytical grade and used without further purification. Distilled water or ultrapure water used in this work was provided by a Millipore purification system at a resistivity of 18.2 MΩ cm (Branstead, USA).
Synthesis of anti-CRP pAb2 antibody-Cu3(PO4)2 hybrid nanoflowers
The hybrid nanoflowers based on polyclonal anti-CRP antibodies and Cu3(PO3)2 were synthesized via a facile and mild biomimetic-mineralizing process similar to previous works.27,28 In detail, rabbit polyclonal anti-CRP antibodies (20 μL, 5.0 mg mL−1) were first dispersed into PBS (975 μL, 10 mM, pH 7.4). Then, a CuSO4 aqueous solution (5.0 μL, 100 mM) was rapidly dropped into the resulting solution. After that, the suspension was gently shaken using a shaker at 4 °C for 72 h. During this process, organic–inorganic doped nanostructures were formed on the basis of chemical reactions. The prepared suspension was centrifuged for 15 min with a centrifugal force of 12
000g at 4 °C. The obtained precipitation (i.e., pAb2-Cu3(PO4)2 nanoflowers) was dispersed in PBS (10 mM, pH 7.4) at a concentration of ∼5.0 mg mL−1 and stored at 4 °C for further use.
Coating of mAb1 on microplates and immunoreaction processes
The mAb1-modified microtiter plates were prepared as follows: initially, anti-CRP mAb1 antibodies (50 μL, 10 μg mL−1 in 50 mM sodium carbonate buffer, pH 9.6) were added into each high-binding polystyrene microplate and incubated at 4 °C for 12 h with the covering of an adhesive plastic plate sealing film. Then, the microplate was washed three times with a washing buffer (0.05% Tween 20, v/v in PBS, 10 mM, pH 7.4). Following that, 300 μL of the blocking buffer (1.0% BSA, w/v, in PBS, 10 mM, pH 7.4) was injected into each well and reacted at 37 °C for 60 min with slight shaking on a shaker. The microplates were washed as explained before. Then, 50 μL of different concentrations of the CRP standard/sample (May be diluted with PBS, 10 mM, pH 7.4) and 50 μL of the as-prepared pAb2-Cu3(PO4)2 suspension (5.0 mg mL−1) were added into the well, and immunoreacted at 37 °C for 50 min under gentle shaking using a shaker. The resulting microplate was washed again and measured using the following electrochemical system.
Anodic-stripping voltammetric measurement processes
To carry out the anodic-stripping voltammetric measurement, a 20 μL aliquot of 1.0 M HNO3 was initially added to each well to dissolve the pAb2-Cu3(PO4)2 nanoflowers for the release of Cu2+ ions under slight shaking (∼8.0 min). Then, the resulting acidic solution containing copper ions was transferred into 2.0 mL acetate buffer (0.2 M, pH 5.6) containing 10.0 μg mL−1 mercury ions (from HgII acetate). The square-wave-anodic-stripping voltammetric (SWASV) measurement was conducted using an AutoLab electrochemical workstation (μAUTIII.FRA2.V, Eco Chemie, Netherlands) with a conventional three-electrode system, containing a platinum-wire as the counter electrode, an Ag/AgCl electrode as the reference electrode, and an in situ formed mercury drop on a glassy carbon electrode as the working electrode with an effective working area of 3.14 mm2. The stripping process involved the pretreatment (1.0 min, +0.6 V) and the accumulation (2.0 min, −1.4 V). All the SWASV measurements were carried out after a 15-second rest period (without stirring) in an applied potential from −0.2 V to +0.2 V with a potential step of 4.0 mV, a frequency of 25 Hz, and an amplitude of 25 mV. A baseline correction of the resulting voltammogram was performed using the μAUTIII.FRA2.V software.
Results and discussion
Characterization of pAb2-Cu3(PO4)2 hybrid nanoflowers
In this system, the anodic-stripping voltammetric immunoassay mainly involves the mAb1-coated microplate preparation, pAb2-Cu3(PO4)2 and electrochemical measurement. Anti-CRP mAb1 antibodies were attached to the microtiter plates by physical adsorption between high-binding polystyrene and the protein. pAb2-Cu3(PO4)2 hybrid nanoflowers were synthesized by a one-pot method accompanying a typical chemical reaction. The introduction of the target CRP triggers the sandwiched immunoreaction between mAb1 antibody and pAb2-Cu3(PO4)2. Under acidic conditions, the released copper ions can be determined by an anodic-stripping voltammetric method with high sensitivity.
To realize our design, a transmission electron microscope (TEM; FEI Talo F200S; Thermo Scientific FEI, USA) was employed to characterize the as-synthesized pAb2-Cu3(PO4)2 hybrid nanostructures. As shown in Fig. 1A, the nanoscales exhibited a flower-like structure with an average size of 115 nm diameter. Meanwhile, we also observed many grooves on the surface of these nanomaterials. Such small and ultra-thin sheet-like structures were conducive to the reaction of biological molecules and the dissolution of particles. The elemental mapping indicated the presence of Cu, P, O and C elements in the hybrid nanoflowers (Fig. 1B–E). Logically, another puzzling question arises as to whether anti-CRP pAb2 antibodies were doped into the nanoflowers. As a control test, pure Cu3(PO4)2 nanostructures were synthesized by the same method in the absence of anti-CRP pAb2 antibodies. Thereafter, we used UV-vis absorption spectrometer (Cary 7000, Agilent, USA) to investigate three components including Cu3(PO4)2, anti-CRP pAb2 antibody and pAb2-Cu3(PO4)2 nanoflowers, respectively (Fig. 1F). Curve ‘a’ shows UV-vis absorption spectra of anti-CRP pAb2 antibodies, and an obvious characteristic peak at 278 nm was observed as a result of the antibody proteins. In contrast, no characteristic peaks were achieved toward Cu3(PO4)2 nanoscales within the wavelength of 200–500 nm (curve ‘b’). Significantly, the characteristic peak for anti-CRP pAb2 antibodies appeared after the formation of pAb2-Cu3(PO4)2 hybrid nanostructures (curve ‘c’). On the basis of these comparative results, we could preliminarily judge the successful synthesis of pAb2-Cu3(PO4)2 nanoflowers.
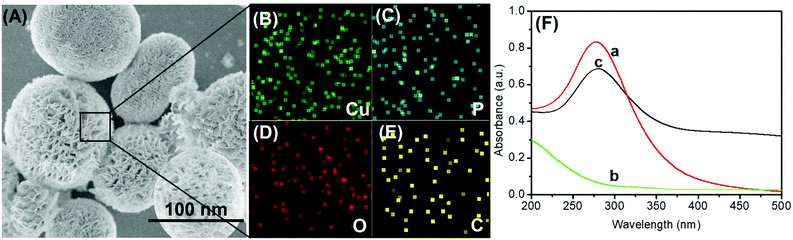 |
| Fig. 1 (A) SEM image of pAb2-Cu3(PO4)2 hybrid nanoflowers; (B–E) elemental mapping of Cu, P, O and C elements; (F) UV-vis absorption spectra of (a) anti-CRP pAb2 antibody, (b) Cu3(PO4)2 nanoparticles and (c) pAb2-Cu3(PO4)2 hybrid nanoflowers. | |
Electrochemical characteristics of pAb2-Cu3(PO4)2 under different conditions
As mentioned above, the anodic-stripping voltammetric signal of the electrochemical immunoassay stemmed from the solution of copper ions under acidic conditions toward pAb2-Cu3(PO4)2 nanoflowers. To demonstrate this issue, a pAb2-Cu3(PO4)2 suspension before and after the reaction with 1.0 M HNO3 was prepared in 2.0 mL acetate buffer (0.2 M, pH 5.6) containing 10.0 μg mL−1 mercury ions using an in situ formed mercury-drop glassy carbon electrode (Fig. 2A). A very weak anodic-stripping voltammetric peak was obtained within the applied potentials for pAb2-Cu3(PO4)2 nanoflowers in the absence of 1.0 M HNO3 (curve ‘a’), which mainly derived from the dissolution of Cu3(PO4)2 through an acetate buffer solution. Favourably, a strong anodic-stripping voltammetric peak appeared after the reaction of pAb2-Cu3(PO4)2 with 1.0 M HNO3 (curve ‘b’), which originated from the water-soluble copper ions. In this case, we also utilized TEM to characterize the resulting solution, and no particles or nanoflowers were found in the HNO3-treated solution (data not shown). These results indicated that the strong anodic-stripping voltammetric peak appeared due to the copper ion solution, but not pAb2-Cu3(PO4)2 nanoflowers.
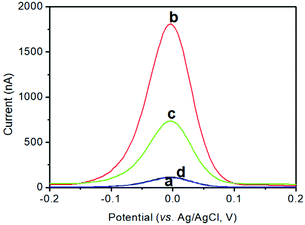 |
| Fig. 2 (a and b) SWASV diagrams of the various components in 2.0 mL acetate buffer (0.2 M, pH 5.6) containing 10.0 μg mL−1 mercury ions (from HgII acetate) toward pAb2-Cu3(PO4)2 (a) before and (b) after the reaction with 1.0 M HNO3. (c and d) SWASV responses of the developed voltammetric immunoassay in the (c) presence and (d) absence of the target CRP (1.0 ng mL−1 used in this case). | |
Next, the as-prepared pAb2-Cu3(PO4)2 nanoflowers were used for the detection of target CRP (1.0 ng mL−1 used as an example) on the mAb1-coated microplate by coupling with the square-wave-anodic-stripping voltammetric (SWASV) measurements. As seen from curve ‘c’, the obtained voltammetric peak current was 708.2 nA toward 1.0 ng mL−1 CRP. Maybe, one question to be produced was whether the SWASV peak was from non-specific adsorption of pAb2-Cu3(PO4)2. For comparison, the pAb2-Cu3(PO4)2 suspension was directly incubated with a mAb1-coated microtiter plate (i.e., in the absence of the target CRP). As indicated from curve ‘d’, the voltammetric peak current was close to zero, suggesting that the strong voltammetric peak currents were obtained from the specific antigen–antibody reaction between the target CRP and pAb2-Cu3(PO4)2/mAb2. Therefore, pAb2-Cu3(PO4)2 could be used preliminarily for CRP detection.
Optimization of experimental conditions
As described above, this optimization was carried out through the antigen–antibody reaction, followed by the anodic-stripping voltammetric measurement. Generally, the formation of the immune complexes needs to be carried out within a certain period of time through the hydrogen bond. In this work, a monoclonal anti-CRP antibody (mAb1) and a polyclonal anti-CRP antibody (pAb2) reacted with the target CRP in the same solution. Fig. 3A represents the effect of immunoreaction time on the SWASV peak current of electrochemical immunoassay using 1.0 ng mL−1 CRP as an example. The voltammetric currents increased with the increase in immunoreaction time and then reached a plateau after 50 min, indicating that the antigen–antibody reaction reached a dynamic equilibrium. To save the assay time, 50 min was chosen for the formation of immunocomplexes.
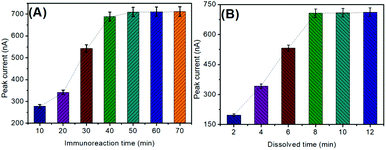 |
| Fig. 3 Effects of (A) immunoreaction time on the antigen–antibody reaction and (B) dissolution time of Cu3(PO4)2 with HNO2 on the SWASV peak current of the developed immunosensing platform (1.0 ng mL−1 CRP was used in this case). | |
Another factor influencing the anodic-stripping voltammetric current of the electrochemical immunoassay depends on the amount of the dissolved copper ions under acidic conditions. In this regard, the chemical reaction time between Cu3(PO4)2 and HNO3 was crucial for the nanoflower dissolution. As shown in Fig. 3B, the optimum peak currents were achieved after 8.0 min, suggesting that the reaction of Cu3(PO4)2 nanoflowers with HNO3 was relatively slow. To achieve an adequate signal, 8.0 min was selected for the dissolution of Cu3(PO4)2 nanoflowers to obtain the copper ion solution.
Calibration plots of the electrochemical immunoassay toward CRP standards
Using the as-prepared pAb2-Cu3(PO4)2 labels, target CRP standards of different concentrations were determined on the mAb1-coated microplates with a sandwiched immunoreaction mode (Scheme 1). The final signal was acquired on the basis of square-wave anodic-stripping voltammetric measurement on an in situ formed mercury drop electrode. Fig. 4A shows the SWASV diagrams of the electrochemical immunoassay toward different concentrations of CRP standards within the applied potentials from −200 mV to +200 mV. The overall trend was that the peak currents increased with the increase in CRP levels. As seen from Fig. 4B, however, the peak current tended to be flat (i.e., no obvious changes) within the range of low-level or high-level CRP standards. Significantly, a good linear relationship between the decimal logarithm of CRP concentrations and SWASV peak currents could be achieved from 10 pg mL−1 to 100 ng mL−1. The linear regression equation was fitted to y (nA) = 311.2 × log
C[CRP] (ng mL−1) + 709.8 (r = 0.9932, n = 5). The limitation of detection (LOD) was calculated for 7.9 pg mL−1 CRP at a signal-to-noise ratio of 3. Moreover, the analytical properties including the linear range and LOD were acceptable relative to previously reported CRP analytical methods (Table 1).
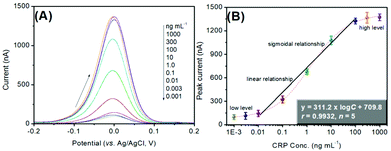 |
| Fig. 4 (A) SWASV responses of the pAb2-Cu3(PO4)2-based immune sensing system toward CRP standards with different concentrations, and (B) the corresponding calibration plots. | |
Table 1 Comparison of analytical properties between the voltammetric immunoassay and other CRP analytical methods on the linear range and LOD (unit: ng mL−1)
Method |
Linear range |
LOD |
Ref. |
UV-vis absorption spectroscopy |
3.0–100 |
1.2 |
29
|
Lateral flow immunoassay |
No application |
0.015 |
7
|
Lateral flow immunoassay |
50–10 000 |
1.3 |
30
|
Voltammetric immunoassay |
1.0–106 |
0.3 |
31
|
Electrochemiluminescence |
0.01–1000 |
0.0046 |
8
|
Impedimetric immunoassay |
No application |
1.2 |
32
|
Magnetoresistance biosensor |
3.0–350 000 |
1.0 |
9
|
Surface acoustic wave sensor |
10–100 |
4.0 |
33
|
Colorimetric immunoassay |
10–180 000 |
10 |
34
|
Lateral flow immunoassay |
100–5000 |
1.0 |
35
|
Electrochemiluniescence |
0.05–6.25 |
0.011 |
36
|
ASV immunoassay |
0.01–100 |
0.0079 |
This work |
Repeatability, specificity and stability of our system
The repeatability of electrochemical immunoassay mainly involved mAb1-coated microplates and the as-prepared pAb2-Cu3(PO4)2 nano labels. In this system, mAb1-coated microplates and pAb2-Cu3(PO4)2 could not be repeatedly used for the detection of target CRP (i.e., disposable) since the hydrogen bond between the antigen and the antibody is not repeatable. Based on this consideration, the immunosensing probes with the different batches were studied for the repeatability. As shown in Table 2, the maximum relative standard deviations (RSDs) were 3.04% (n = 4) and 6.72% (n = 4) for the same-batch (intraassay) and various-batch (interassay) immunosensing probes, respectively, indicating good reproducibility.
Table 2 Repeatability of the mAb1-coated microplates and pAb2-Cu3(PO4)2 prepared through different batches (conc.: ng mL−1; assay time: nA)
|
Conc. |
1 |
2 |
3 |
4 |
RSD (%) |
Intrassay |
0.1 |
398.5 |
394.6 |
401.2 |
405.6 |
1.16 |
10 |
1119.5 |
1165.3 |
1107.3 |
1178.9 |
3.04 |
Interassay |
0.1 |
403.2 |
389.7 |
396.5 |
378.5 |
2.69 |
10 |
1021.3 |
1132.5 |
1198.2 |
1087.4 |
6.72 |
The selective detection of the pAb2-Cu3(PO4)2-based immunosensing system toward CRP is very important for the newly developed analytical method.37 To monitor this concern, other acute-phase reactants [e.g., α-antitrypsin (ATS), α-antichymotrypsin (ACTS), and ceruloplasmin (CP)] and biomarkers [e.g., alpha-fetoprotein (AFP) and carcinoembryonic antigen (CEA)] were measured using our developed electrochemical immunoassay (note: the comparison of peak currents toward these analytes was carried out between the low-level CRP and high-level non-target). Fig. 5A shows the experimental results for these analytes. It was found that the strong peak currents could be acquired in the presence of the target CRP, and other non-targets gave the very weak signal. Meanwhile, the voltammetric signal of the mixture including the CRP and non-target was almost the same as the target CRP alone, thus exhibiting good specificity.
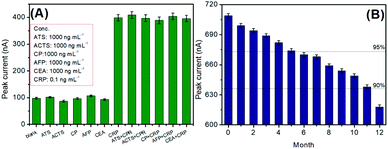 |
| Fig. 5 (A) Selectivity of the pAb2-Cu3(PO4)2-based immune sensing system, and (B) the storage stability of mAb1-coated microplates and pAb2-Cu3(PO4)2 probes. | |
Certainly, the long-term stability of the prepared immunosensing probes is necessary for CRP analysis. When not in use, mAb1-coated microplates and pAb2-Cu3(PO4)2 probes were stored at 4 °C. During the storage period, they were intermittently used to analyze 1.0 ng mL−1 CRP. As seen from Fig. 5B, the detectable voltammetric signals could maintain 95% and 90% of the initial current after 5 and 11 months, respectively, thereby suggesting acceptable stability.
Evaluation of real samples
According to local ethics, we collected three human serum samples including the target CRP from the Department of Clinical Laboratory, Fujian Provincial Hospital, China. To investigate the merits of our system, sample no. 2 was diluted to different folds with a blank human serum. These samples were assayed using the developed electrochemical immunoassay, and the obtained results were compared with those analysed at the hospital (for the reference). As shown in Table 3, the RSDs per sample between the two methods were less than 8.99%, indicating the good accuracy for the analysis of real samples.
Table 3 Measurements for human serum samples before and after dilution using the electrochemical immunoassay and human CRP ELISA kit (unit: ng mL−1)
|
Sample no.a |
Method [mean ± SD (RSD, %), n = 3] |
RSDb (%) |
Electrochemistry |
ELISA kit |
Samples 4–6 were obtained via diluting sample 2 to 10×, 100× and 1000× fold with blank human serum sample.
RSD values were calculated on the basis of the mean value per sample between two methods.
|
Undiluted |
1 |
78.2 ± 2.1 (2.69) |
82.1 ± 1.3 (1.58) |
3.44 |
2 |
84.6 ± 1.4 (1.65) |
79.3 ± 1.9 (2.40) |
4.57 |
3 |
36.4 ± 1.6(4.40) |
38.4 ± 0.9 (2.34) |
3.78 |
Diluted |
4 |
8.16 ± 0.4 (4.90) |
8.01 ± 0.7 (8.74) |
1.31 |
5 |
0.87 ± 0.06 (6.90) |
0.93 ± 0.02 (2.15) |
4.71 |
6 |
0.092 ± 0.007 (7.61) |
0.081 ± 0.004 (4.94) |
8.99 |
Conclusions
In this contribution, we have successfully designed an in situ amplified anodic-stripping voltammetric immunoassay for the sensitive detection of C-reactive proteins. This system combined the high-sensitive anodic-stripping voltammetric method with the specific antigen–antibody immunoreaction. The immunosensing probes such as mAb1 capture antibody-coated microplates and pAb2-Cu3(PO4)2 nano labels were simply prepared or synthesized prior to measurement. The introduction of strong HNO3 acid was performed after specific immunoreaction to release the detectable copper ions. The signal-generation tags with the flower-like Cu3(PO4)2 nanostructures were favourable for acidic dissolution. In addition, numerous copper ions could be released from the nanoflowers, thereby resulting in the ASV signal amplification of the electrochemical immunoassay.
Compliance with ethical standards
All the experiments were performed in accordance with the Guidelines of Fujian University of Traditional Chinese Medicine (China), and approved by the ethics committee at Fujian University of Traditional Chinese Medicine (China). Informed consent was obtained for any experimentation with human subjects.
Conflicts of interest
There are no conflicts to declare.
Acknowledgements
Authors received financial support from the Natural Science Foundation of Fujian Province (grant no.: 2020J01311402).
References
- I. Letchumanan, M. Arshad and S. Gopinath, Curr. Med. Chem., 2021, 28, 986–1002 CrossRef CAS PubMed.
- X. Chen, J. Shi, L. Liu, L. Liao, Y. Wang and H. Mo, Acta Medica Mediterr., 2021, 37, 1347–1354 Search PubMed.
- X. Li and Z. Chen, Medicine, 2021, 100, e25977 CrossRef CAS PubMed.
- M. Socha, B. Malinowski, O. Puk, M. Wartega, P. Bernard, M. Nowaczyk, B. Wolski and M. Wicinski, Crit. Rev. Oncol. Hematol., 2021, 164, 103419 CrossRef PubMed.
- T. Nagy-Simon, A. Hada, S. Suarasan and M. Potara, J. Mol. Struct., 2021, 1246, 131178 CrossRef CAS.
- M. Lucio, A. Montoto, E. Fernandez, S. Alamri, T. Kunze, M. Banuals and A. Maquieira, Biosens. Bioelectron., 2021, 193, 113561 CrossRef CAS PubMed.
- V. Panferov, N. Byzova, A. Zherdev and B. Dzantiev, Microchim. Acta, 2021, 188, 309 CrossRef CAS PubMed.
- D. Hong, K. Kim, E. Jo and M. Kim, Anal. Chem., 2021, 93, 7925–7932 CrossRef CAS PubMed.
- F. Meng, L. Zhang, W. Huo, J. Lian, A. Jesorka, X. Shi and Y. Gao, ACS Omega, 2021, 6, 12923–12930 CrossRef CAS PubMed.
- P. Kanyong, C. Catli and J. Davis, Anal. Chem., 2020, 92, 4707–4710 CrossRef CAS PubMed.
- L. Huang, J. Chen, Z. YU and D. Tang, Anal. Chem., 2020, 92, 2809–2814 CrossRef CAS PubMed.
- Z. Luo, Q. Qi, L. Zhang, R. Zeng, L. Su and D. Tang, Anal. Chem., 2019, 91, 4149–4156 CrossRef CAS PubMed.
- B. Zhang, B. Liu, D. Tang, R. Niessner, G. Chen and D. Knopp, Anal. Chem., 2012, 84, 5392–5399 CrossRef CAS PubMed.
- X. Pei, B. Zhang, J. Tang, B. Liu, W. Lai and D. Tang, Anal. Chim. Acta, 2013, 758, 1–18 CrossRef CAS PubMed.
- J. Shu and D. Tang, Anal. Chem., 2020, 92, 363–377 CrossRef CAS PubMed.
- R. Zeng, K. Lian, B. Su, L. Lu, J. Lin, D. Tang, S. Lin and X. Wang, Angew. Chem., Int. Ed., 2021, 133, 25259–25266 CrossRef.
- L. Zhu, Z. Yin, Z. Lv, M. Li and D. Tang, Analyst, 2021, 146, 4487–4494 RSC.
- Z. Yu, G. Cai, X. Liu and D. Tang, Anal. Chem., 2021, 93, 2916–2925 CrossRef CAS PubMed.
- Z. Yu, H. Gong, Y. Li, J. Xu, J. Zhang, Y. Zeng, X. Liu and D. Tang, Anal. Chem., 2021, 93, 13389–13397 CrossRef CAS PubMed.
- A. M. Lenhoff, J. Chromatogr., 2011, 1218, 8748–8759 CrossRef CAS PubMed.
- A. Sun, Q. Qi and L. Zhi, Microchim. Acta, 2020, 187, 485 CrossRef CAS PubMed.
- B. Liu, K. Singh, S. Gong, M. Canakci, B. Osborne and S. Thayumanavan, Angew. Chem., Int. Ed., 2021, 60, 12831–12818 Search PubMed.
- J. Chen, F. Xue, Z. Yu, L. Huang and D. Tang, Analyst, 2020, 145, 7186–7190 RSC.
- M. Romero, D. Mombru, F. Pignanelli, R. Faccio and A. Monbru, Front. Chem., 2020, 8, 537 CrossRef CAS PubMed.
- R. Ren, G. Cai, Z. Yu, Y. Zeng and D. Tang, Anal. Chem., 2018, 90, 11099–11105 CrossRef CAS PubMed.
- S. Lv, K. Zhang, L. Zhu and D. Tang, Anal. Chem., 2020, 92, 1470–1476 CrossRef CAS PubMed.
- Q. Tang, L. Zhang, X. Tan, L. Jiao, Q. Wei and H. Li, Biosens. Bioelectron., 2019, 133, 94–99 CrossRef CAS PubMed.
- R. Ye, C. Zhu, y. Song, Q. Lu, X. Ge, X. Yang, M. Zhu, D. Du, H. Li and Y. Lin, Small, 2016, 12, 3094–3100 CrossRef CAS PubMed.
- A. Kokorina, R. Rashchevskaya and I. Goryacheva, Anal. Bioanal. Chem., 2021, 413, 6867–6875 CrossRef PubMed.
- R. Pang, Q. Zhu, J. Wei, Y. Wang, F. Xu, X. Meng and Z. Wang, RSC Adv., 2021, 11, 28288–28394 Search PubMed.
- Y. Cheng, T. Zhan, X. Feng and G. Han, J. Electroanal. Chem., 2021, 895, 115417 CrossRef CAS.
- A. Adesina and P. Mashazi, Front. Chem., 2021, 9, 587142 CrossRef CAS PubMed.
- M. Jeng, Y. Li, M. Sharma, C. Chen, C. Tsai, Y. Lin, S. Huang, L. Chang and C. Lai, Chemosensors, 2021, 9, 106 CrossRef CAS.
- P. Gupta, S. Son and G. Seong, Microchim. Acta, 2021, 188, 119 CrossRef CAS PubMed.
- Y. Panraksa, X. Apilux, S. Jampasa, S. Puthong, C. Henry, S. Rengpipat and O. Chailapskul, Sens. Actuators, B, 2021, 329, 129241 CrossRef CAS.
- R. Zhou, C. Fang, J. Yan and Y. Tu, Talanta, 2019, 205, 120135 CrossRef PubMed.
- Z. Cheng, R. Wang, Y. Xing, L. Zhao, J. Choo and F. Yu, Analyst, 2019, 144, 6533–6540 RSC.
|
This journal is © The Royal Society of Chemistry 2022 |