DOI:
10.1039/D1CP02596A
(Perspective)
Phys. Chem. Chem. Phys., 2021,
23, 23413-23427
Challenges in tin perovskite solar cells
Received
9th June 2021
, Accepted 20th August 2021
First published on 20th August 2021
Abstract
Perovskite solar cells are the rising star of third-generation photovoltaic technology. With a power conversion efficiency of 25.5%, the record efficiency is close to the theoretical maximum efficiency of a single-junction solar cell. However, lead toxicity threatens commercialization efforts and market accessibility. In this context, Sn-based perovskites are a safe alternative. Nevertheless, the efficiency of Sn-based devices falls far behind the efficiency of Pb-based counterparts. This concise review sheds light on the challenges that the field faces toward making Sn-based perovskites the perovskite photovoltaic benchmark. We identified four key challenges: materials and solvents, film formation, Sn(II) oxidation, and energy band alignment. We illustrate every single challenge and highlight the most successful attempts to overcome them. Finally, we provide our opinion on the most promising trends of this field in the future.
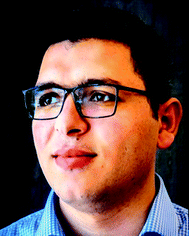
Mahmoud Aldamasy
| Mahmoud Aldamasy received his MPhil degree in Physical Chemistry from Zagazig University, Egypt. He has been working at Egyptian petroleum research institute (EPRI) on petroleum applications. He is now a DAAD PhD scholar at Helmholtz Center Berlin for Energy and Materials and working on finding new solvents for tin perovskites at the department of novel materials and interfaces for photovoltaic solar cells. |
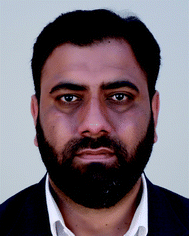
Zafar Iqbal
| Zafar Iqbal is a DAAD PhD Scholar at Helmholtz-Zentrum Berlin für Materialien und Energie (HZB). He has earned his MPhil degree in Physical Chemistry from Quaid-i-Azam University, Islamabad. He has been working as a researcher at Department of Chemistry and Chemical Engineering, LUMS, Lahore. He is working on Inorganic tin perovskite halides for energy applications. |
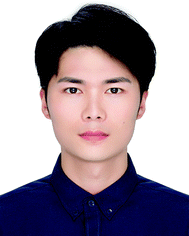
Guixiang Li
| Guixiang Li is currently working as a PhD student at Helmholtz-Zentrum Berlin für Materialien und Energie GmbH. Previously, he received his master degree from South China University of Technology in 2019. Guixiang's current research includes solution-engineered lead-free tin-based perovskite materials and their optoelectronic applications. |
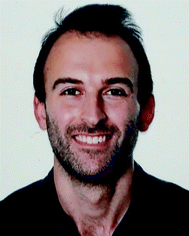
Jorge Pascual
| Jorge Pascual Mielgo is a post-doc in Helmholtz-Zentrum Berlin under the supervision of Antonio Abate. His current research focuses on the development of efficient and stable lead-free tin halide perovskite devices. He graduated in Chemistry from the University of the Basque Country and specialised in the field of Organic Chemistry. In 2019, he obtained his PhD degree in Polymat (Donostia-San Sebastian) on the study of fullerenes as additives in metal halide perovskite thin films. |
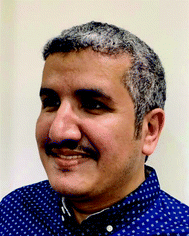
Fahad Alharthi
| Fahad A. Alharthi is an associate professor at King Saud University in Saudi Arabia. He got his PhD from Hull university, UK in 2016. His research interests are focussed on inorganic nanomaterials for renewable energy and environmental remediation. |
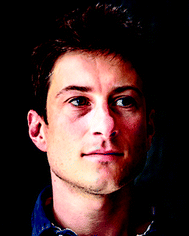
Antonio Abate
| Antonio Abate is team leader at Helmholtz-Centrum Berlin and a tenure track professor at University of Naples Federico II. He is researching solar energy conversion with perovskite solar cells. Before the current position, Antonio was leading the solar cell research at the University of Fribourg in Switzerland. He was a Marie SkłodowskaCurie Fellow at École Polytechnique Fédérale de Lausanne. He worked for four years as a postdoctoral researcher at the University of Oxford and the University of Cambridge. Antonio graduated from University of Naples Federico II in 2006, and he got his PhD at Politecnico di Milano in 2011. |
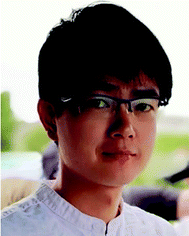
Meng Li
| Meng Li received his PhD degree in materials science and engineering under the supervision of Prof. Liang-Sheng Liao and Prof. Zhao-Kui Wang from Soochow University, P. R. China, in 2018. Currently, he is a postdoctoral research fellow in the laboratory of Prof. Antonio Abate at the Helmholtz-Zentrum Berlin für Materialien und Energie (HZB), Germany. His primary research interest is focused on organic and inorganic/organic hybrid materials for application in solar cells. His main research topic is non-toxic photovoltaic materials and devices for outdoor and indoor applications. |
1. Motivation
Exploring the photoactive properties of halide perovskites started twenty years ago as a scientific curiosity, and it resulted in a novel type of photovoltaics and optoelectronics.1 Perovskite solar cells (PSCs) grabbed the attention from the photovoltaic community due to their ease of fabrication, optimum optoelectronic properties, low cost, material abundance, and increasing power conversion efficiencies (PCEs) that quickly increased from 3.8% in 2009 to 25.5% in 2021.2 These advantageous characteristics encouraged an unprecedented number of researchers to work on developing PSCs. With the inherited knowledge and techniques from previously developed technologies, especially silicon and organic photovoltaics, notable progress has been made in the last ten years to overcome the hurdles that exist in industrialization and commercialization of PSCs.3 It is the first time an emerging technology has quickly overtaken older technologies like dye-sensitized solar cells (DSSC) and compete with other commercially available technologies such as crystalline silicon solar cells, cadmium telluride (CdTe), and copper indium gallium selenide (CIGS) in terms of power conversion efficiency (PCE).4–6 However, long-term stability7,8 and lead toxicity9,10 still hinder PSCs from accessing the market, at least on the short term.11
The toxicological nature of lead increases fears of environmental implications that could hinder PSCs' investments and market adoption. This perspective made the replacement or reduction of lead in PSCs a core activity of several research groups. Nevertheless, the current record efficiencies rely on lead as the metallic component inside the perovskite crystal.12
According to the US Environmental Protection Agency (EPA), the maximum allowed lead concentrations are 0.15 μg m−3 and 0.15 μg L−1 in air and water, respectively. The directives adopted by the European Union against the use of lead limit its concentration in electronic devices to less than 0.1% of the weight of each homogeneous material inside the device. However, current state-of-the-art lead-based perovskite materials exceed this threshold by at least tenfold taking into consideration the high water solubility of lead compounds used in PSCs.13 As a backup strategy, the photovoltaic community started looking for a lead-free perovskite technology14–16 in order to lower or even eliminate lead from PSCs completely.115
Applying the perovskite dimensionality rules, we realize that a very limited number of metal ions could form a high-performing and environmentally friendly perovskite structure,17,18 such as Bi/Sb-based halides, a semi-perovskite structure, double perovskites, and Sn/Ge-based perovskites.18 Sn attracted the most attention among these candidates as it fulfills the charge balance, coordination, and ionic size prerequisites.19 Tin perovskites carry many environmental advantages over lead perovskites. Tin could be more toxic to living organisms than lead when leaked to the environment. However, it is less bioavailable due to the lower water solubility of oxygenated Sn4+ compounds.20,21
Tin perovskites show exciting electrical and optoelectronic properties enabling them to excel lead perovskites in terms of photovoltaic performance. The outer electronic configuration of tin is very similar to that of lead (ns2, np2), and the atomic size of both elements is comparable (1.49 Å for Pb(II) and 1.35 Å for Sn(II)). However, the atomic number of lead is higher than that of tin, thanks to the relativistic effect.22 Compared to lead perovskites, tin perovskites show a very sharp absorption edge with a direct bandgap ranging from 1.35 to 1.4 eV, close to the Shockley–Queisser limit with an estimated theoretical efficiency of above 30% (e.g., 33.7% at 1.34 eV). Tin perovskites also show higher charge mobilities and a higher external photoluminescence quantum yield (PLQY).23,24 These properties would enable Sn-based perovskites to overcome their Pb analogs in terms of efficiency while already showing very promising applications for quantum dots, sensors, detectors, and transistors.25,26
Currently, tin perovskites still lie in the back behind Pb-based counterparts in terms of efficiency due to several causes. First, Sn(II) oxidation to Sn(IV), which causes self-p-doping and devastates the performance of the devices. Second, fast crystallization dynamics, Sn-based perovskites crystallize very fast compared to Pb-based perovskites, which results in nonuniform crystallization, smaller perovskite domains, pinholes, and a considerable amount of defects. Third, energy band alignment, most of the charge transport layers are designed primarily for Pb-based perovskites, but when used blindly for Sn-based perovskites, we find energy band mismatch, which is very harmful to the overall performance of Sn-perovskite devices (Fig. 1).22
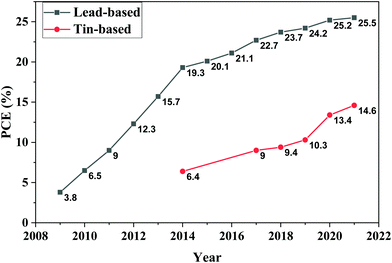 |
| Fig. 1 Efficiency progress of Sn- and Pb-based PSCs over time. | |
Chronological overview
The first successful attempt to synthesise Sn-based perovskites dates back to 1974 by Scaife and co-workers, where they synthesized and characterized all-inorganic CsSnX3, where X3 is Cl3, BrCl2, Br3, Br2I, BrI2, and I3.27 Five years later, Parry and co-workers prepared and studied the optoelectronic properties of the same all-inorganic Sn-based perovskite materials.28,29 However, hybrid Sn perovskites were never reported until 1988 when Yamada and co-workers prepared and investigated the phase transition properties of MASnBr3 (MA = CH3NH3+).30–32 From the mid-90s till 2001, Mitzi and co-workers studied the dimensionality and structural engineering of different structures of layered perovskites.33 In addition, they reported more detailed studies on the thermal, optical, magnetic, and electrical properties of layered Sn-based perovskites.19,34 It was also reported that the conductivity of such layered perovskites varies with the thickness or the number of perovskite layers; in other words, its electric behavior changes from semiconducting to metallic by increasing the number of perovskite layers.35,36 Furthermore, they exploited the optoelectronic properties, low cost, and low-temperature processability of Sn-based perovskites by demonstrating them as a semiconducting channel in thin-film field-effect transistors; this was the first reported electronic application of a hybrid lead-free tin-based halide perovskite material.37,38
Sn-Based perovskite materials started to attract attention from the photovoltaic community, especially after 2009 when Chung and co-workers reported the first solar cell based on MAPbI3 and MAPbBr3 perovskite photoactive materials.1 The first report about Sn perovskites was published in 2012 by Chung and co-workers, where they employed CsSnI3 doped with SnF2 as a hole transporting material (HTM) in a DSSC using dye N719 as the solar absorber, and they achieved a PCE of 10.2% (8.51% with a mask).39 Later on, in the same year, Shum and co-workers employed CsSnI3 with a bandgap of 1.3 eV as an independent light absorber in a Schottky solar cell and achieved a PCE of 0.9%.40 In 2013, the PV community started to reinvestigate and exploit the optoelectronic properties of Sn-based perovskites as a potential candidate for solar light harvesting.41–44 The first promising efficiencies were reported in 2014 when Snaith and co-workers reported a PCE of 6.4% based on MASnI3 as a light absorber.15 Four days later, Hao and co-workers reported an efficiency of around 5.7% utilizing MASnI3 and MASnI3−xBrx as photoactive absorber materials.16 These initial results gave the solar cell community a sense of trust in Sn-based materials as potential nontoxic contenders for the rocketing Pb-based perovskites.45–47
In early 2016, Lee and co-workers reported FASnI3-based PSCs (FA = NH2CH = NH2+) utilizing SnF2–pyrazine complexes to guarantee a uniform dispersion of SnF2 to avoid perovskite phase separation; an efficiency of 4.8% was achieved, and the encapsulated cells maintained 98% of its initial efficiency for 100 days.48,49 Later on, Liao and co-workers achieved a PCE of 6.22% based on inverted planar FASnI3 utilizing the solvent engineering method.50 In 2017, the PSC community witnessed remarkable achievements in terms of both efficiency and stability of Sn-based PSCs, most of which were based on inspired techniques from Pb-based PSCs such as cation mixing,51 incorporating low dimensional perovskites,52 and utilizing hollow53,54 and mixed 2D–3D perovskites.55 Such practices led to a jump in the PCE from 6% in 2016 to around 9%,55 and significantly enhanced stability.53 Zhao and co-workers adopted the cation mixing strategy to improve cell efficiency; by optimizing the ratio of the FA+ and MA+ cation mixture, a PCE of 8.12% was obtained along with an improved perovskite film morphology.51 Shao and co-workers mixed 92% of highly crystalline 3D FASnI3 with 8% of 2D Sn perovskites in a p–i–n planar device; this increased the PCE to 9% with negligible hysteresis, low shunt losses, and very low trap-assisted recombination.55 In early 2018, Ran and co-workers reported a 2D–3D heterojunction Sn-based PSC using phenylethylammoniumiodide (PEAI) as a 2D and FASnI3 as a 3D perovskite material and a PCE of 6.98% was obtained.56 At the same time, Liu and co-workers improved the morphology of perovskite layers by using hot antisolvent and annealing under a low partial pressure of DMSO.57 Both groups reported a PCE of around 7%. In 2019, PCEs of Sn-based PSCs continued to surge, approaching the 10% threshold through two different approaches. Jokar and co-workers reported the first approach by using bulk organic molecules such as ethylenediammonium diiodide (EDAI2) and butylammonium iodide (BAI) to improve film morphology and improve crystallinity in addition to surface passivation, and they achieved a PCE of 8.9%.58 Later on, the same group used guanidinium cation GA+ as a nonpolar organic cation mixed with an FA+ cation in the ratio of 20
:
80 respectively in addition to 1% ethylenediammonium diiodide (EDAI2) as an additive to suppress Sn(II) oxidation to Sn(IV) and passivate surface defects; 9.6% was achieved after storage in a glovebox environment for 2000 h; this gradual increase in efficiency was attributed to the slow surface passivation and crystal relaxation.59 The second approach was reported by Wang and co-workers. They grew parallel-orientated 2D PEA2SnI4 on the surface of 3D FASnI3, using pseudohalogen NH4SCN as a structure regulator. This hierarchical 2D-quasi-2D–3D Sn-based perovskite structure delivered a PCE of 9.41% with improved air stability and high reproducibility.60 In 2020, Nishimura and co-workers achieved a PCE of 13.24% by partially substituting FA+ with ethylammonium (EA+), which enhanced the film morphology and charge extraction.61 Recently, two high efficiencies were reported, 13.4%62 using phenylhydrazine with FA as mixed cations and a mixture of halogens Cl− and Br− as mixed anions by Wang and co-workers; however, the record efficiency in tin perovskites is 14.63% using one-step synthesis of SnI2-solvent adducts.63 In terms of stability, Liu and co-workers reported FASnI3 layers prepared via templated crystal growth with a PCE of 11.22% and decent stability of over 1000 h of light soaking while retaining 95% of the initial efficiency.64 These improvements and efficiencies approaching 15% were possible through the big effort of the community. And, despite all the strategies implemented, Sn-based PSCs must still face important challenges if they are to reach the expected performance. The photovoltaic parameters of the best performing tin-based perovskite devices in recent years is summarized in Table 1.
Table 1 Summary of the evolution of tin-based perovskite solar cells
Tin perovskite |
Year |
Photovoltaic parameters |
Ref. |
V
oc [V] |
J
sc [mA cm−2] |
FF |
PCE [%] |
MASnI3 |
2014 |
0.88 |
16.8 |
42 |
6.4 |
15
|
FASnI3 |
2016 |
0.46 |
22 |
61 |
6.2 |
50
|
FASnI3 |
2017 |
0.53 |
24.1 |
71 |
9.0 |
55
|
FASnI3 |
2018 |
0.58 |
21.3 |
72 |
8.9 |
58
|
FASnI3 |
2018 |
0.61 |
22 |
70 |
9.4 |
60
|
FASnI3 |
2019 |
0.62 |
21.2 |
73 |
9.6 |
59
|
FASnI3 |
2020 |
0.84 |
20.3 |
78 |
13.2 |
61
|
FASnI2Br |
2020 |
0.81 |
23 |
72 |
13.4 |
62
|
FASnI3 |
2021 |
0.91 |
20.6 |
77 |
14.6 |
63
|
In this review, we discuss the bottlenecks that hinder Sn-based PSCs from achieving efficiencies comparable to those of Pb-based PSCs.65 First, we discuss the effect of solvents and materials on the performance and stability of the devices and the recent efforts to find new solvent systems for FASnI3. Second, we discuss the film formation and crystallization dynamics in Sn-based perovskites and talk about the most effective tries to make oriented and highly crystalline films. In particular, we discuss the use of 2D/3D mixed perovskites to make highly efficient Sn-based PSCs. After that, we discuss the oxidation of Sn(II) to Sn(IV) and the most successful attempts to quench it. Finally, we discuss the energy band alignment and its effect on charge extraction and overall device efficiency.
2. Precursors & solvents
To find the main reason behind the low efficiency in Sn-based halide perovskites,66 we have to take a step backward and follow up the processing steps from the beginning. We should start with the materials we use to prepare the precursor solutions. For example, commercially available reagents may contain a small but effective amount of Sn(IV) (<0.1%), which is enough to convert an ASnX3 film into a p-type material, with devastating effects on the photovoltaic performance of devices.67 Therefore, analyzing the purity of raw materials is necessary to guarantee that Sn(IV) is not present as an initial component, minimizing the incorporation of oxidised species and alleviating self-p-doping. Furthermore, it is also vital for perovskite film formation, as we will explain in a later section.68
Several researchers placed great importance on material purification, and different approaches were reported. Ozaki and co-workers followed a chemical purification strategy in which they formed a tin halide-solvent complex [SnX2(S)n] where S is a solvent (S = DMF and DMSO).69,70 In the beginning, they characterized SnI2 using 119Sn magic-angle spinning (MAS) NMR spectroscopy, thermogravimetric analysis (TGA), and Karl Fischer titration. They found that SnI2 contains around 10% of SnI4 and about 10
000 ppm of water, although the bottle was labeled 99.99% purity. The 119Sn-NMR spectra in Fig. 2a show a peak at −1742 ppm, which is attributed to the SnI4. TGA confirmed the same results as 10.1% weight loss was observed at ∼150 °C, which corresponds to the sublimation of SnI4 (Fig. 2b), while SnI2 sublimates at 330 °C. Next, Ozaki and co-workers utilized the difference in the sublimation temperature between SnI2 and SnI4 to purify SnI2 using the vacuum sublimation technique where SnI4 sublimates and then condensates as an orange powder at ∼150 °C.69119Sn-NMR and TGA analyses of the purified SnI2 using the vacuum sublimation method are shown in Fig. 2c and d.
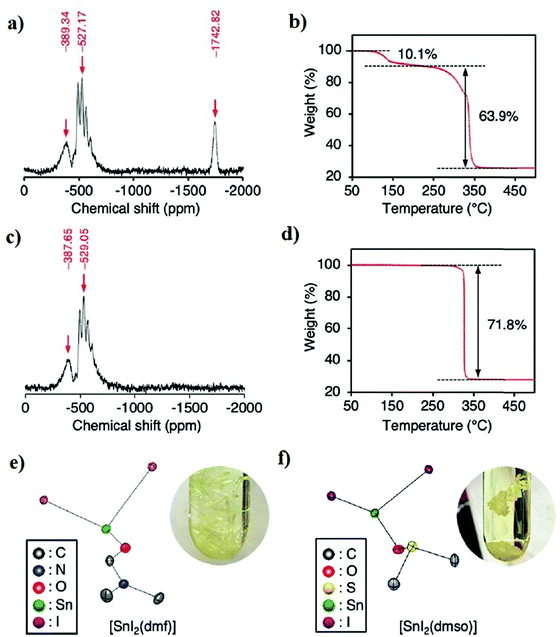 |
| Fig. 2 Purified SnI2 reagents, (a and b) 119Sn MAS NMR spectra, and TGA analysis of commercially purchased SnI2. (c and d) 119Sn MAS NMR spectra and TGA analysis of purified SnI2 using the vacuum sublimation method. (e and f) Structural illustrations of [SnI2(DMF)] and [SnI2(DMSO)].69 Reproduced with permission Copyright 2020, American Chemical Society. | |
They recrystallized SnI2 in DMF and DMSO to remove any SnO2 residuals from the vacuum sublimation step for further purity. SnI2 from the first step was dissolved in DMF and DMSO, and then toluene or dichloromethane was poured gently into the solution, which converted into needle crystals of [SnI2(DMSO)], [SnI2(DMSO)2] and [SnI2(DMF)] as determined by single-crystal XRD (Fig. 2e and f). The purity of these crystals was confirmed using elemental analysis and 119Sn MAS NMR spectroscopy and found to be stable under an inert atmosphere and could be used conveniently as a pure starting reagent for tin perovskites. The authors merged these purifying techniques with other film formation methods and additives to achieve a PCE of ∼12% for FASnI3.
Solvents have a very strong influence on perovskite film crystallization and, hence, on the device performance.71,72 Nevertheless, in Sn-based PSCs, solvents have a much more profound influence due to the sensitivity of Sn(II) towards oxidation.73 In parallel, Sargent's group and our group independently found that DMSO was undergoing a redox reaction with Sn(II), oxidizing it to Sn(IV).74,75 Solid-state 119Sn-NMR analysis of the received SnI2 did not show any Sn(IV) peaks, implying no content of it or a concentration lower than the measured detection limit of 1.5 wt%. By exposing the same sample to air, the Sn(IV) signal appeared and increased with exposure to air. After that, fresh precursor solutions of FASnI3 were prepared and characterized for the presence of Sn(IV). Fortunately, there was no Sn(IV) at room temp, but after heating the solutions at 100 °C for 30 minutes to simulate the conditions of the film formation, the color of the DMSO-based FASnI3 changed to dark red, and Sn(IV) peaks were detected in the 119Sn-NMR spectrum.75 To further understand the oxidation mechanism of Sn(II) to Sn(IV), 13C-NMR and 1H-NMR analyses were performed for the organic species in precursor solutions (DMSO, FAI, and MAI). 1H-NMR showed a peak at ∼1.9 ppm for both MA+ and FA+ containing solutions, and this peak matches with the expected peak of dimethylsulphide (DMS) which confirms the same finding reported earlier by Saidaminov and co-workers. The same peak was found in the 13C-NMR spectrum, which confirms the formation of DMS as a product of the Sn(II) oxidation reaction (Fig. 3b). Previous results confirm the occurrence of a redox reaction between DMSO and Sn(II) which is promoted in the presence of an acidic environment76,77 (Fig. 3c).
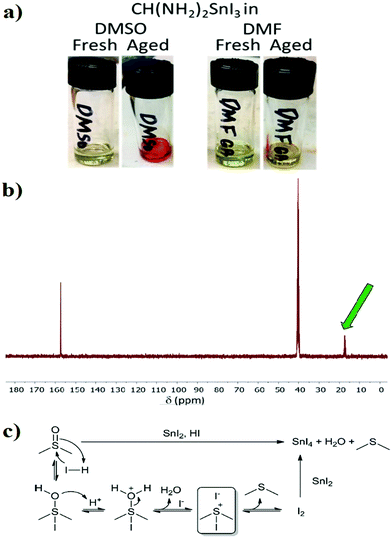 |
| Fig. 3 Oxidative effect of DMSO, (a) FASnI3 solutions in DMSO and DMF before and after ageing for 5 h at 120 °C.74 Reproduced with permission Copyright 2020, American Chemical Society. (b) Mechanism of Sn(II) oxidation by DMSO. (c) 13C-NMR spectra of FASnI3 heated at 100 °C for 30 min. The green arrow indicates the DMS-related peak.75 Reproduced with permission, Copyright 2021, The Royal Society of Chemistry. | |
Considering the negative impact of DMSO, so far the universal solvent for tin perovskites, on material stability, our group decided to replace it by new solvent combinations.78 We targeted solvents with the ability to form a perovskite solution with a concentration over 1 M and be stable after thermal soaking for at least 12 hours at 100 °C. For this, we screened most of the possible functional groups with particular attention to dipolar aprotic solvents which could dissolve ionic molecules such as FAI and SnI2 using their strong dipoles. Some solvents were excluded, such as strong acids, solvents with big aromatic structures commonly used as antisolvents, solvents with too low boiling point such as methylamine, and solvents with a high melting point. Out of 2000 suggested molecules, we tested 76 solvents for their solubility, stability, and ability to form a perovskite solution. Experimentally, we identified 12 solvents that could form a stable perovskite solution with six different functional groups with the amide group being the mostly presented (Fig. 4a). To ensure that these new precursors could form perovskite materials, the easiest way was to drop-cast the precursor on a glass substrate, and then anneal at 150 °C for 20 minutes with a thermal ramp of 12 °C min−1. It was impressive that 15 out of 17 solvents (including DMSO and DMF) formed a black phase. XRD characterization was performed for the drop-casted films of the new solvents, and the resultant peak positions are in agreement with the standard positions of crystalline FASnI3 films. However, some samples have low crystallinity, and some other samples have different phases; such optimizations can be dealt with in the film formation stage.
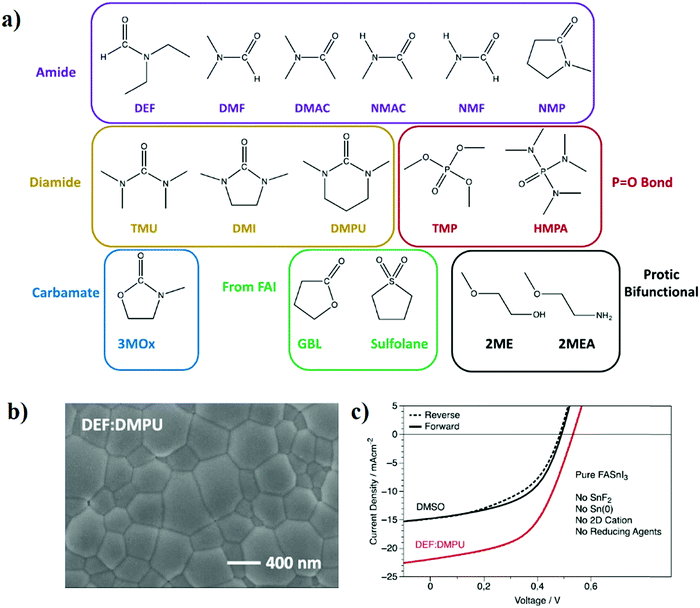 |
| Fig. 4 (a) 16 nonsulfoxide solvents that formed stable perovskite solution divided into groups according to the functional group. (b) SEM images of the DEF:DMPU film. (c) J–V curves of FASnI3 without any additives in DEF:DMPU against DMSO.78 Reproduced with permission Copyright 2021, American Chemical Society. | |
The ability of the new solvents to form a working perovskite device was also tested. A mixture of two solvents, namely N,N-diethylformamide (DEF) and N,N′-dimethylpropyleneurea (DMPU), in a 6
:
1 ratio achieved a PCE of 6.2% for FASnI3 without using any additives. Meanwhile, additive-free DMSO samples achieved a PCE of only 3.84% (Fig. 4b and c). These results prove that finding a new stable solvent for processing Sn-based perovskites could be an entrance towards achieving highly efficient and durable tin-based PSCs.
3. Film formation
The formation of high-quality perovskite films is a vital prerequisite to push the performance of PSCs forward.79 The crystalline quality and morphology of perovskite films are related directly to the overall performance of the device. To make high-quality perovskite films, we have to understand and manipulate the mechanism of crystal formation that starts from the precursor solution and ends at the end of the annealing step.80 The perovskite crystallization process depends on several factors such as the substrate nature, temperature, spin coating parameters, solvent, and antisolvent interaction.81 Film formation dynamics and crystallization kinetics in Sn-based perovskites are different from those of lead-based perovskites.82
Although many reports focused on studying the crystallization dynamics and kinetics of Pb-based perovskites through in situ techniques,82,83 very few reports dealt with the same point in Sn-based perovskites. Dong and co-workers studied the crystallization mechanism of tin perovskites using several techniques, offering essential insights that revealed the mechanism of structural revolution, material transformation, and crystallization dynamics in mixed 2D/3D Sn-based perovskites.84 They found that crystallization tends to start at the precursor/air interface, but in 3D Sn-based perovskites, the crystallization rate is comparable in bulk and at the precursor/air interface. Moreover, the addition of 2D molecules hinders the crystallization process in bulk, which allows uniform and highly oriented crystals with a similar structure to the 3D perovskites. The crystallization dynamics, in general, were found to be very fast compared to the lead-based counterpart.
The attempts to optimize the quality of Sn-based perovskite films are numerous. Most of them rely on chemical additives that could form 2D perovskites on top of the regular 3D perovskite layer, usually known as Ruddlesden–Popper perovskites.60,85,86
Liu and co-workers reported a PCE of 11.22% based on highly crystalline FASnI3 films with preferential orientation along the (001) plane.64 They reported a solvent pretreatment method using a mixture of n-propylammoniumiodide (PAI) and DMSO (1
:
100 v/v). DMSO acts as a solvent to provide the liquid interface for nucleation. At the same time, PAI aggregates around the newborn nuclei of FASnI3 and forms a template that regulates the crystal growth and orients it along the (100) plane. PAI was used in a minimal amount compared to DMSO (1
:
100 v/v) as it is not considered as an additive to the precursor solution or co-solvent because it is not forming 2D perovskite that covers the surface of 3D perovskites. The mechanism of action of PAI is shown in Fig. 5a. SEM images in Fig. 5b indicate a big difference in the grain sizes between FASnI3 with and without PAI. The bigger the grain and the domains, the less the grain boundaries and the less the defects. In templated growth FASnI3 (TG-FASnI3), the defect density was lowered from 2.89 × 1016 cm−3 to 5.41 × 1015 cm−3. The XRD patterns in Fig. 5c show an increasing intensity of the 100 plane peak with an increase in the concentration of PAI from 1 to 2 mg mL−1, while it decreases again after increasing the concentration to 5 mg mL−1, which indicates that the optimum concentration of PAI is around 2 mg mL−1. The effect of such a small quantity of PAI on the crystallization and the photovoltaic performance of FASnI3 was tremendous as shown in Fig. 5d–g. TG-FASnI3 shows an increase in PL intensity which confirms the low defect density and the longer carrier diffusion length than regular control FASnI3. The prepared devices based on TG-FASnI3 showed much better operational stability compared to the control devices. Fig. 5g shows the results of maximum power point tracking (MPPT) of both devices encapsulated and stored in an N2 filled glove box. 95% of the initial efficiency was maintained after 1000 h of operation, while the control devices lost around 60% of their initial efficiency after 500 h.
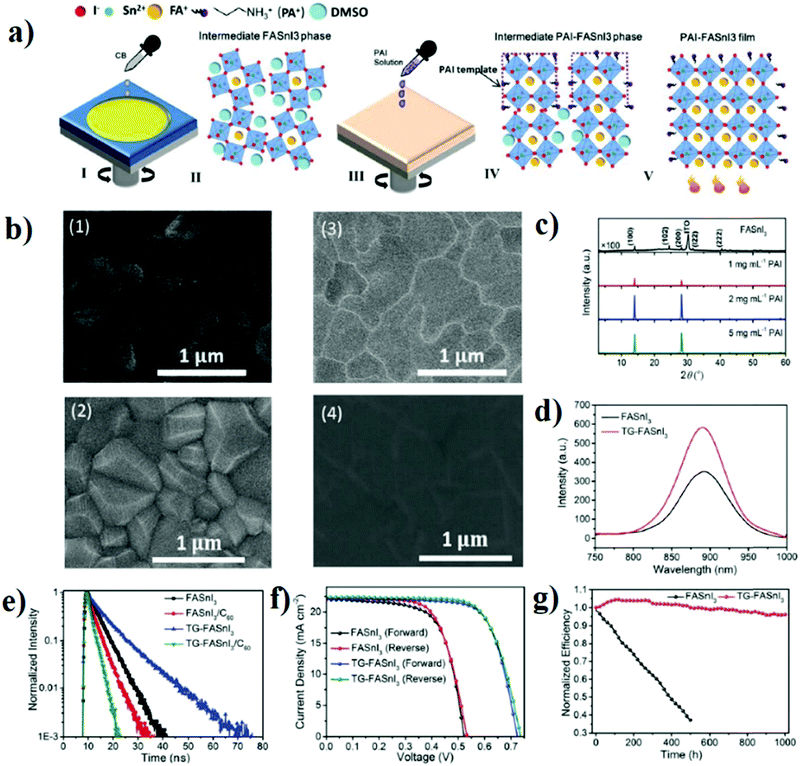 |
| Fig. 5 (a) Proposed mechanism of action of TG-FASnI3. (b) SEM images of FASnI3 (1) before and (2) after annealing; TG-FASnI3 (3) before and (4) after annealing. (c) XRD patterns of FASnI3 and TG-FASnI3. (d) PL spectra of FASnI3 and TG-FASnI3. (e) TRPL of FASnI3 and TG-FASnI3. (f) J–V curves of FASnI3 and TG-FASnI3 in forward and reverse scanning. (g) Stability test of FASnI3 and TG-FASnI3 under simulated AM 1.5 G (100 mW cm−2) operating at MPPT.64 Reproduced with permission, Copyright 2020, The Royal Society of Chemistry. | |
Finally, one of the bottlenecks that tin-based perovskites face is the fast crystallization leading to low film quality compared to that of lead-based perovskites, which leads to a high defect density in the range of 1015–1016, while in lead-based perovskites, it is 1013–1014 cm−3. This high defect density is one of the main reasons behind the low efficiency of tin-based perovskites.87
4. Sn(II) oxidation
Oxidation of Sn(II) is one of the bottlenecks to achieve good efficiency in Sn-based perovskites solar cells. Sn(II) is more vulnerable to oxidation which is electronically and chemically favorable.88 It has been revealed through theoretical calculations that tin oxidation is favorable on the pristine surface and unfavorable in the bulk. Resultantly, bulk Sn(IV) changes into Sn(II), which releases two holes to the valence band and by p-doping the perovskite,68 this has been experimentally proved that tin vacancies lead to p-doping which limits the diffusion length.15,89
Although Sn and Pb are present in the same group of the periodic table, it has been found that the Sn 5s2 orbital has higher energy as compared to the 6s2 orbital of Pb which increases its contribution to the valence band edge. The electrons of 6s2 in Pb show an inert pair effect due to lanthanide contraction but 5s2 lacks this phenomenon which ultimately makes Sn electrons loosely bound and easy to lose.90
To stop the oxidation of tin and to improve the stability of the perovskite films and devices, different SnX2 species have been employed in excess to stop p-doping. For the first time, Kumar and co-workers showed that the introduction of SnF2 in inorganic tin halide CsSnI3 reduces the Sn vacancies.91 Later on, they have also reported that by the introduction of SnF2 in FASnI3 films, the oxidation of Sn(II) has been effectively reduced, and there is an increase in the Jsc values of the device.92 SnF2 has also been introduced in CsSnBr3 films, and it has been found that it suppresses the oxidation of Sn(II).93 In the most recent work on Sn halide perovskite-based devices, SnF2 has been used as an important dopant as it suppresses the oxidation of Sn(II), improves film morphology, and also improves Jsc.94 Other SnX2 species like SnCl2, SnBr2 and SnI2 improve the film quality, device performances95 energy alignments91 and also surface passivation.96
Hydrazine and its derivatives were used by many researchers as a reducing material in Sn-based perovskites. Hydrazine in the vapor phase has been used in MASnI3 and CsSnI3 film formation and for device fabrication.97 The ratio of Sn(IV) to Sn(II) has been reduced by using hydrazine vapors. It has been found that hydrazine molecules release electrons which suppress the oxidation of Sn(II).98 By introducing phenylhydrazine hydrochloride salt (PHCl) in the FASnI3 film, Wang and co-workers reported 11.4% efficiency. It has been noticed that incorporating the molecules in the crystal lattice improves the device performance.99 Similarly, by adding 8 mol% trihydrazine dihydriodide (THDH) to FASnI3 solution, the oxidation of Sn(II) was suppressed, and the champion device efficiency was reported to be 8.50%. Another derivate of hydrazine 4-fluorobenzohydrazide (FBH) was introduced in FASnI3, and it significantly enhanced the efficiency as compared to that of the control device. The champion device efficiency was reported to be 9.03% by using FBH molecules.100 Recently, Wang and co-workers achieved a record efficiency of 13.4% (12.4% certified) through the introduction of synergistic effects between PH cations and halide anions.62
T. Wang and co-workers introduced gallic acid (GA) as an antioxidant along with SnCl2 for FASnI3 films. By exploring the underlying chemistry of GA and the tin precursor, they reported 9.03% efficiency.101 Kayesh and co-workers used 5-ammonium valeric acid (5-AVAI) to enhance the oxidation stability of FASnI3 perovskite films. 5-AVAI not only suppresses the Sn(II) oxidation but also enhances the crystallinity by making a hydrogen bond with SnI6. The devices show improved stability with 7% efficiency.102
The primary precursor used for tin halide perovskites is SnI2. It is oxidized by air. To make it purer, Sn metal powder is used. Low purity has a high level of doping which ultimately decreases the efficiency. Huang and co-workers used tin powder to purify tin sources from Sn(IV) moieties, which has increased the device performance parameters.103 Recently, Nakamura and co-workers have reported a different technique to make Sn(IV) free devices. They have produced Sn metal nanoparticles from SnF2 over SnBr2 and SnCl2 by using 1,4-bis(trimethylsilyl)-2,3,5,6-tetramethyl-1,4-dihydropyrazine (TM-DHP) molecules. These Sn metal nanoparticles can reduce SnI4 to SnI2 to make it a Sn(IV) free system. By using this technique, they have reported more than 11.2% certified efficiency.104
5. Device structure and energy alignment
In device operation, when light is shone on the device, due to the low bandgap, the electrons from the valence band of the perovskite halide materials shift to the conduction band and leave the hole vacancies in the valence band. These generated electrons and holes diffuse in the ETM and HTM and are finally collected at the cathode and anode. These ETM and HTM materials should be efficient enough to transport the electrons and holes across the boundary. This phenomenon is only possible when the highest occupied molecular orbitals (HOMO) and lowest unoccupied molecular orbitals (LUMO) of the hole transport material and electron transport layer are well aligned with the conduction and the valence band of the perovskite material. For better performance and stability of the devices, hole transport materials and electron transport layers are chosen accordingly.105
Conventional HTMs in Sn-based perovskites were designed originally for Pb-based perovskites and then tried for Sn-based devices after showing a good performance. However, the positions of energy bands in Sn-based perovskites are slightly different from those of Pb-based perovskites. This leads to mismatching in the alignment of the energy bands of the absorber material with charge transfer layers on both sides of the Sn-based absorber film.106 For instance, the conventional HTM used in inverted devices poly(3,4-ethylenedioxythiophene):poly(styrenesulfonate) (PEDOT:PSS) has a work function of about −5.0 eV (higher than FASnI3) and 2,2′,7,7′-tetrakis[N,N-di(p-methoxyphenylamine)]-9,9-spirobifluorene (spiro-OMeTAD) has an even more higher HOMO level that is −5.22 eV. Due to these poor energy alignments, Sn-based PSCs are unable to achieve their theoretical predicted efficiency.
Initially, Noel and co-workers have reported Sn-based perovskite halide-based regular devices with efficiencies of greater than 6% under simulated full sunlight using MASnI3 as a perovskite material. Later on, it was found that spiro-OMeTAD, which is used as the hole transport material in regular structure devices, needs dopants like lithium bis(trifluoromethanesulfonyl)imide (Li-TFSI) and 4-tert-butylpyridine (tBP), which enhance its hole mobility. These dopants have a harmful effect on the perovskite layer107 and in addition to that, they increase the rate of charge recombination by forming bonds between TiO2 and Sn (Fig. 6).108
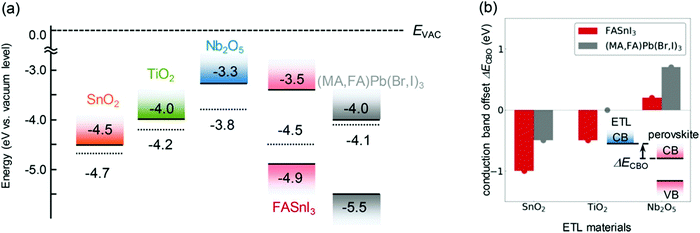 |
| Fig. 6 (a) Band profiles of each ETL material and perovskite material obtained experimentally from the ionization potential, the electron affinity, and the work function. The dotted lines indicate the Fermi energy, and the vacuum level (EVAC) is plotted as zero. (b) Conduction band offset (CBO) at the ETL/perovskite layer interface when using each of the oxides. The CBO (ΔECBO) is defined as the energy difference between the electron affinity of the ETL material and that of the perovskite material based on the perovskite material.109 Reproduced with permission Copyright 2020, American Chemical Society. | |
After these attempts, the first inverted device reported 3.30% efficiency, which was fabricated by using CsSnI3 as the perovskite material, NiOx as the hole transport layer, and PCBM and Al as ETLs. By using PEDOT:PSS as the HTL, Liao and co-workers reported 6.22% efficiency.50 Later on, Liu and co-workers tuned the energy levels of PEDOT:PSS by using polyethylene glycol (PEG). Fullerene molecules are used as ETMs in inverted devices. To know which derivative has the best energy alignment with Sn perovskites. C60, PCBM, and ICBA were used as ETLs for FA0.9PEA0.1SnI3. Among all, ICBA with work functions of −3.73 and −5.73 has perfect energy alignment with the perovskite and has a higher Voc value as compared to others. Due to the dopant issues in spiro-OMETAD, Ke and co-workers reported dopant-free organic molecules such as tetrakis-triphenylamine (TPE) as the HTM for Sn-based PSCs, and they reported 7.23% champion device efficiency.110 Similarly, due to the shortcomings of PEDOT:PSS, Cao and co-workers introduced copper thiocyanate (CuSCN) as the HTM for the FASnI3 based device and acquired 7.34% efficiency.111 The conventional electron transport layer in a Pb-based perovskite solar cell has an energy mismatch with that of a Sn halide-based perovskites solar cell. Yokoyama and co-workers used Nb2O5 as the electron transport material in place of TiO2 in a regular inverted device. It has been found that it decreased the conduction offset and interfacial charge recombinations.109
This study reveals that the conduction band of Nb2O5 is closest to zero for FASnI3 perovskite materials; this increases the Voc of Sn PSCs as compared to that of TiO2 and SnO2. To gain a good energy alignment, Cong and co-workers introduced an organic molecule poly[tetraphenylethene3,3′-(((2,2-diphenylethene-1,1-diyl)bis(4,1-phenylene))bis(oxy)) bis (N,N-dimethylpropan-1-amine)tetraphenylethene] (PTN-Br) in FASnI3 to modify grain boundaries. It has the highest occupied molecular orbital (HOMO) energy level of −5.41 eV, due to which it forms an energy alignment with the Sn halide perovskites solar cell.112 Precise measurements of the energy levels of tin-based perovskites were provided by Shuxia and co-workers.106 These values are beneficial for making a new cascade route for electrons and holes.
6. Conclusion and outlook
Sn-based PSCs carry enough optoelectronic properties to achieve the maximum theoretical efficiency of a single junction solar cell according to the QS limit. We believe that it will also be a key component in the multi-junction solar cell industry. So far, an efficiency of 14.6% has been achieved, which is 10% less than where it should be compared to those of Pb-based PSCs. However, to achieve such ambitious goals, Sn-based PSCs must overcome four bottlenecks, namely: (1) pure starting chemicals and non-oxidising solvents, (2) better film formation dynamics, (3) Sn(II) oxidation suppression and (4) better energy band alignment. To overcome these limitations, we need to focus on finding new, stable, and effective solvents for Sn-based PSCs. We also need to understand the Sn chemistry inside the precursor, and through film formation steps, customize new contact layers that have better matching with the energy bands of Sn-based compositions, and stabilizing Sn(II) through all the device making steps. Then we could provide a high protection and isolation level to devices under operation using modern encapsulation techniques.113,114
Conflicts of interest
There are no conflicts of interest to declare.
Acknowledgements
M. A. and Z. I. thank DAAD and Egyptian missions for PhD scholarship. The authors acknowledge financial support from the Natural Science Foundation of China (No. 51903181). G. L. thanks China Scholarship Council (CSC) for financial support (Grant No. 201906150131). Authors thanks the Distinguished Scientist Fellowship Program (DSFP) at KSU for financial support.
References
- A. Kojima, K. Teshima, Y. Shirai and T. Miyasaka, Organometal Halide Perovskites as Visible-Light Sensitizers for Photovoltaic Cells, J. Am. Chem. Soc., 2009, 131, 6050–6051 CrossRef CAS PubMed.
- NREL, Best Research-Cell Efficiencies, https://www.nrel.gov/pv/assets/pdfs/best-research-cell-efficiencies-190416.pdf, accessed 18 June 2019.
- A. K. Jena, A. Kulkarni and T. Miyasaka, Halide Perovskite Photovoltaics: Background, Status, and Future Prospects, Chem. Rev., 2019, 119, 3036–3103 CrossRef CAS PubMed.
- J. Gong, M. Flatken, A. Abate, J.-P. Correa-Baena, I. Mora-Seró, M. Saliba and Y. Zhou, The Bloom of Perovskite Optoelectronics: Fundamental Science Matters, ACS Energy Lett., 2019, 4, 861–865 CrossRef CAS.
- W.-J. Yin, T. Shi and Y. Yan, Unique Properties of Halide Perovskites as Possible Origins of the Superior Solar Cell Performance, Adv. Mater., 2014, 26, 4653–4658 CrossRef CAS PubMed.
- Z. Li, T. R. Klein, D. H. Kim, M. Yang, J. J. Berry, M. F. A. M. van Hest and K. Zhu, Scalable fabrication of perovskite solar cells, Nat. Rev. Mater., 2018, 3, 18017 CrossRef CAS.
- S. Albrecht and B. Rech, Perovskite solar cells: On top of commercial photovoltaics, Nat. Energy, 2017, 2, 16196 CrossRef.
- L. Meng, J. You and Y. Yang, Addressing the stability issue of perovskite solar cells for commercial applications, Nat. Commun., 2018, 9, 5265 CrossRef CAS PubMed.
- M. Lira-Cantú, Perovskite solar cells: Stability lies at interfaces, Nat. Energy, 2017, 2, 1–3 Search PubMed.
- R. Wang, M. Mujahid, Y. Duan, Z. K. Wang, J. Xue and Y. Yang, A Review of Perovskites Solar Cell Stability, Adv. Funct. Mater., 2019, 1808843, 1–25 Search PubMed.
- A. Abate and Perovskite Solar, Cells Go Lead Free, Joule, 2017, 1, 659–664 CrossRef CAS.
- Q. Jiang, Y. Zhao, X. Zhang, X. Yang, Y. Chen, Z. Chu, Q. Ye, X. Li, Z. Yin and J. You, Surface passivation of perovskite film for efficient solar cells, Nat. Photonics, 2019, 1–25 Search PubMed.
- O. US EPA, Lead Laws and Regulations.
- F. Giustino and H. J. Snaith, Toward Lead-Free Perovskite Solar Cells, ACS Energy Lett., 2016, 1(6), 1233–1240 CrossRef CAS.
- N. K. Noel, S. D. Stranks, A. Abate, C. Wehrenfennig, S. Guarnera, A. A. Haghighirad, A. Sadhanala, G. E. Eperon, S. K. Pathak, M. B. Johnston, A. Petrozza, L. M. Herz and H. J. Snaith, Lead-free organic-inorganic tin halide perovskites for photovoltaic applications, Energy Environ. Sci., 2014, 7, 3061–3068 RSC.
- F. Hao, C. C. Stoumpos, D. H. Cao, R. P. H. Chang and M. G. Kanatzidis, Lead-free solid-state organic–inorganic halide perovskite solar cells, Nat. Photonics, 2014, 8, 489–494 CrossRef CAS.
- W. Li, Z. Wang, F. Deschler, S. Gao, R. H. Friend and A. K. Cheetham, Chemically diverse and multifunctional hybrid organic–inorganic perovskites, Nat. Rev. Mater., 2017, 2, 16099 CrossRef.
- L. Mao, C. C. Stoumpos and M. G. Kanatzidis, Two-Dimensional Hybrid Halide Perovskites: Principles and Promises, J. Am. Chem. Soc., 2019, 141, 1171–1190 CrossRef CAS PubMed.
- D. B. Mitzi, Synthesis, Crystal Structure, and Optical and Thermal Properties of (C4H9NH3)2MI4 (M = Ge, Sn, Pb), Chem. Mater., 1996, 8(3), 791–800 CrossRef CAS.
- A. Babayigit, D. Duy Thanh, A. Ethirajan, J. Manca, M. Muller, H.-G. Boyen and B. Conings, Assessing the toxicity of Pb- and Sn-based perovskite solar cells in model organism Danio rerio, Sci. Rep., 2016, 61(6), 1–11 Search PubMed.
- J. Li, H.-L. Cao, W.-B. Jiao, Q. Wang, M. Wei, I. Cantone, J. Lü and A. Abate, Biological impact of lead from halide perovskites reveals the risk of introducing a safe threshold, Nat. Commun., 2020, 11, 1–5 CAS.
- T. Wu, X. Liu, X. Luo, X. Lin, D. Cui, Y. Wang, H. Segawa, Y. Zhang and L. Han, Lead-free tin perovskite solar cells, Joule, 2021, 5, 863–886 CrossRef CAS.
- I. Poli, G. W. Kim, E. L. Wong, A. Treglia, G. Folpini and A. Petrozza, High External Photoluminescence Quantum Yield in Tin Halide Perovskite Thin Films, ACS Energy Lett., 2021, 6, 609–611 CrossRef CAS PubMed.
- M. Lyu, J.-H. Yun, P. Chen, M. Hao and L. Wang, Addressing Toxicity of Lead: Progress and Applications of Low-Toxic Metal Halide Perovskites and Their Derivatives, Adv. Energy Mater., 2017, 7, 1602512 CrossRef.
- S. Shao, W. Talsma, M. Pitaro, J. Dong, S. Kahmann, A. J. Rommens, G. Portale and M. A. Loi, Field-Effect Transistors Based on Formamidinium Tin Triiodide Perovskite, Adv. Funct. Mater., 2021, 31, 2008478 CrossRef CAS.
- A. Filippetti, S. Kahmann, C. Caddeo, A. Mattoni, M. Saba, A. Bosin and M. A. Loi, Fundamentals of tin iodide perovskites: a promising route to highly efficient, lead-free solar cells, J. Mater. Chem. A, 2021, 9, 11812–11826 RSC.
- D. E. Scaife, P. F. Weller and W. G. Fisher, Crystal preparation and properties of cesium tin(II) trihalides, J. Solid State Chem., 1974, 9, 308–314 CrossRef CAS.
- D. E. Parry, M. J. Tricker and J. D. Donaldson, The electronic structure of CsSnBr3 and related trihalides; Studies using XPS and band theory, J. Solid State Chem., 1979, 28, 401–408 CrossRef CAS.
- S. J. Clark, C. D. Flint and J. D. Donaldson, Luminescence and electrical conductivity of CsSnBr3, and related phases, J. Phys. Chem. Solids, 1981, 42, 133–135 CrossRef CAS.
- K. Yamada, S. Nose, T. Umehara, T. Okuda and S. Ichiba, 81Br NQR and 119Sn Mössbauer Study for MSnBr3 (M = Cs and CH3NH3), Bull. Chem. Soc. Jpn., 1988, 61, 4265–4268 CrossRef CAS.
- K. Yamada, T. Matsui, T. Tsuritani, T. Okuda and S. Ichiba,
127I-NQR, 119Sn Mössbauer Effect, and Electrical Conductivity of MSnI3 (M = K, NH4, Rb, Cs, and CH3NH3), Z. Naturforsch., A: Phys. Sci., 1990, 45, 307–312 CrossRef CAS.
- K. Yamada, Y. Kuranaga, K. Ueda, S. Goto, T. Okuda and Y. Furukawa, Phase Transition and Electric Conductivity of ASnCl3 (A = Cs and CH3NH3), Bull. Chem. Soc. Jpn., 1998, 71, 127–134 CrossRef CAS.
- D. B. Mitzi, Templating and structural engineering in organic–inorganic perovskites, J. Chem. Soc., Dalton Trans., 2001, 0, 1–12 RSC.
- D. B. Mitzi, C. A. Feild, Z. Schlesinger and R. B. Laibowitz, Transport, Optical, and Magnetic Properties of the Conducting Halide Perovskite CH3NH3SnI3, J. Solid State Chem., 1995, 114, 159–163 CrossRef CAS.
- D. B. Mitzi, C. A. Feild, W. T. A. Harrison and A. M. Guloy, Conducting tin halides with a layered organic-based perovskite structure, Nature, 1994, 369, 467–469 CrossRef CAS.
- D. B. Mitzi, S. Wang, C. A. Feild, C. A. Chess and A. M. Guloy, Conducting Layered Organic-inorganic Halides Containing-Oriented
Perovskite Sheets, Science, 1995, 267, 1473–1476 CrossRef CAS PubMed.
- A. Dodabalapur, L. Torsi, H. E. Katz, A. Callegari and J. M. Shaw, Organic Transistors: Two-Dimensional Transport and Improved Electrical Characteristics, Science, 1995, 268, 270–271 CrossRef CAS PubMed.
- D. B. Mitzi, C. D. Dimitrakopoulos and L. L. Kosbar, Structurally Tailored Organic–Inorganic Perovskites: Optical Properties and Solution-Processed Channel Materials for Thin-Film Transistors, Chem. Mater., 2001, 13(10), 3728–3740 CrossRef CAS.
- I. Chung, B. Lee, J. He, R. P. H. Chang and M. G. Kanatzidis, All-solid-state dye-sensitized solar cells with high efficiency, Nature, 2012, 485, 486–489 CrossRef CAS PubMed.
- Z. Chen, J. J. Wang, Y. Ren, C. Yu and K. Shum, Schottky solar cells based on CsSnI3 thin-films, Appl. Phys. Lett., 2012, 101, 093901 CrossRef.
- G. Murtaza, S. Muhammad, S. Naeem, M. Khalid and A. Manzar, Physical Properties of CsSnM3 (M = Cl, Br, I): A First Principle Study, Acta Phys. Pol., A, 2013, 71(15), 102–107 Search PubMed.
- L. Huang and W. R. L. Lambrecht, Electronic band structure, phonons, and exciton binding energies of halide perovskites CsSnCl3, CsSnBr3, and CsSnI3, Phys. Rev. B: Condens. Matter Mater. Phys., 2013, 88, 165203 CrossRef.
- C. C. Stoumpos, C. D. Malliakas and M. G. Kanatzidis, Semiconducting Tin and Lead Iodide Perovskites with Organic Cations: Phase Transitions, High Mobilities, and Near-Infrared Photoluminescent Properties, Inorg. Chem., 2015, 52(15), 9019–9038 CrossRef PubMed.
- F. Brivio, A. B. Walker and A. Walsh, Structural and electronic properties of hybrid perovskites for high-efficiency thin-film photovoltaics from first-principles, APL Mater., 2013, 1, 042111 CrossRef.
- J.-H. Im, I.-H. Jang, N. Pellet, M. Grätzel and N.-G. Park, Growth of CH3NH3PbI3 cuboids with controlled size for high-efficiency perovskite solar cells, Nat. Nanotechnol., 2014, 9, 927–932 CrossRef CAS PubMed.
- Z. Ren, A. Ng, Q. Shen, H. C. Gokkaya, J. Wang, L. Yang, W.-K. Yiu, G. Bai, A. B. Djurišić, W. W. Leung, J. Hao, W. K. Chan and C. Surya, Thermal Assisted Oxygen Annealing for High Efficiency Planar CH3NH3PbI3 Perovskite Solar Cells, Sci. Rep., 2015, 4, 6752 CrossRef PubMed.
- M. A. Green, A. Ho-Baillie and H. J. Snaith, The emergence of perovskite solar cells, Nat. Photonics, 2014, 8, 506–514 CrossRef CAS.
- S. J. Lee, S. S. Shin, Y. C. Kim, D. Kim, T. K. Ahn, J. H. Noh, J. Seo and S. Il Seok, Fabrication of Efficient Formamidinium Tin Iodide Perovskite Solar Cells through SnF2–Pyrazine Complex, J. Am. Chem. Soc., 2016, 138, 3974–3977 CrossRef CAS PubMed.
- J. Pascual, M. Flatken, R. Felix, G. Li, S.-H. Turren-Curz, M. H. Aldamasy, C. Hartmann, M. Li, D. Di Girolamo, G. Nasti, E. Hüsam, R. G. Wilks, A. Dallmann, M. Bär, A. Hoell and A. Abate, Fluoride Chemistry in Tin Halide Perovskites, Angew. Chem., 2021, 60, 2–11 CrossRef.
- W. Liao, D. Zhao, Y. Yu, C. R. Grice, C. Wang, A. J. Cimaroli, P. Schulz, W. Meng, K. Zhu, R.-G. Xiong and Y. Yan, Lead-Free Inverted Planar Formamidinium Tin Triiodide Perovskite Solar Cells Achieving Power Conversion Efficiencies up to 6.22%, Adv. Mater., 2016, 28, 9333–9340 CrossRef CAS PubMed.
- Z. Zhao, F. Gu, Y. Li, W. Sun, S. Ye, H. Rao, Z. Liu, Z. Bian and C. Huang, Mixed-Organic-Cation Tin Iodide for Lead-Free Perovskite Solar Cells with an Efficiency of 8.12%, Adv. Sci., 2017, 4, 1700204 CrossRef PubMed.
- Y. Liao, H. Liu, W. Zhou, D. Yang, Y. Shang, Z. Shi, B. Li, X. Jiang, L. Zhang, L. N. Quan, R. Quintero-Bermudez, B. R. Sutherland, Q. Mi, E. H. Sargent and Z. Ning, Highly Oriented Low-Dimensional Tin Halide Perovskites with Enhanced Stability and Photovoltaic Performance, J. Am. Chem. Soc., 2017, 139, 6693–6699 CrossRef CAS PubMed.
- W. Ke, C. C. Stoumpos, M. Zhu, L. Mao, I. Spanopoulos, J. Liu, O. Y. Kontsevoi, M. Chen, D. Sarma, Y. Zhang, M. R. Wasielewski and M. G. Kanatzidis, Enhanced photovoltaic performance and stability with a new type of hollow 3D perovskite {en}FASnI3, Sci. Adv., 2017, 3, e1701293 CrossRef PubMed.
- W. Ke, C. C. Stoumpos, I. Spanopoulos, L. Mao, M. Chen, M. R. Wasielewski and M. G. Kanatzidis, Efficient Lead-Free Solar Cells Based on Hollow {en}MASnI3 Perovskites, J. Am. Chem. Soc., 2017, 139, 14800–14806 CrossRef CAS PubMed.
- S. Shao, J. Liu, G. Portale, H.-H. Fang, G. R. Blake, G. H. ten Brink, L. J. A. Koster and M. A. Loi, Highly Reproducible Sn-Based Hybrid Perovskite Solar Cells with 9% Efficiency, Adv. Energy Mater., 2018, 8, 1702019 CrossRef.
- C. Ran, J. Xi, W. Gao, F. Yuan, T. Lei, B. Jiao, X. Hou and Z. Wu, Bilateral Interface Engineering toward Efficient 2D−3D Bulk Heterojunction Tin Halide Lead-Free Perovskite Solar Cells, ACS Energy Lett., 2018, 3(3), 713–721 CrossRef CAS.
- J. Liu, M. Ozaki, S. Yakumaru, T. Handa, R. Nishikubo, Y. Kanemitsu, A. Saeki, Y. Murata, R. Murdey and A. Wakamiya, Lead-Free Solar Cells based on Tin Halide Perovskite Films with High Coverage and Improved Aggregation, Angew. Chem., Int. Ed., 2018, 57, 13221–13225 CrossRef CAS PubMed.
- E. Jokar, C.-H. Chien, A. Fathi, M. Rameez, Y.-H. Chang and E. W.-G. Diau, Slow surface passivation and crystal relaxation with additives to improve device performance and durability for tin-based perovskite solar cells, Energy Environ. Sci., 2018, 11, 2353–2362 RSC.
- E. Jokar, C.-H. Chien, C.-M. Tsai, A. Fathi and E. W.-G. Diau, Robust Tin-Based Perovskite Solar Cells with Hybrid Organic Cations to Attain Efficiency Approaching 10%, Adv. Mater., 2019, 31, 1804835 CrossRef PubMed.
- F. Wang, X. Jiang, H. Chen, Y. Shang, H. Liu, J. Wei, W. Zhou, H. He, W. Liu and Z. Ning, 2D-Quasi-2D-3D Hierarchy Structure for Tin Perovskite Solar Cells with Enhanced Efficiency and Stability, Joule, 2018, 2, 2732–2743 CrossRef CAS.
- K. Nishimura, M. A. Kamarudin, D. Hirotani, K. Hamada, Q. Shen, S. Iikubo, T. Minemoto, K. Yoshino and S. Hayase, Lead-free tin-halide perovskite solar cells with 13% efficiency, Nano Energy, 2020, 74, 104858 CrossRef CAS.
- C. Wang, Y. Zhang, F. Gu, Z. Zhao, H. Li, H. Jiang, Z. Bian and Z. Liu, Illumination Durability and High-Efficiency Sn-Based Perovskite Solar Cell under Coordinated Control of Phenylhydrazine and Halogen Ions, Matter, 2021, 4, 709–721 CrossRef CAS.
- X. Jiang, H. Li, Q. Zhou, Q. Wei, M. Wei, L. Jiang, Z. Wang, Z. Peng, F. Wang, Z. Zang, K. Xu, Y. Hou, S. Teale, W. Zhou, R. Si, X. Gao, E. H. Sargent and Z. Ning, One-Step Synthesis of SnI2·(DMSO)x Adducts for High-Performance Tin Perovskite Solar Cells, J. Am. Chem. Soc., 2021, 143, 10970–10976 CrossRef CAS PubMed.
- X. Liu, T. Wu, J. Y. Chen, X. Meng, X. He, T. Noda, H. Chen, X. Yang, H. Segawa, Y. Wang and L. Han, Templated growth of FASnI3 crystals for efficient tin perovskite solar cells, Energy Environ. Sci., 2020, 13, 2896–2902 RSC.
- G. Nasti and A. Abate, Adv. Energy Mater., 2020, 10, 1902467 CrossRef CAS.
- D. He, L. Shen, Y. Bai and L. Wang, Rational strategies toward efficient and stable lead-free tin halide perovskite solar cells, Mater. Chem. Front., 2021, 5, 4107–4127 RSC.
- T. Leijtens, R. Prasanna, A. Gold-Parker, M. F. Toney and M. D. McGehee, Mechanism of Tin Oxidation and Stabilization by Lead Substitution in Tin Halide Perovskites, ACS Energy Lett., 2017, 2, 2159–2165 CrossRef CAS.
- D. Ricciarelli, D. Meggiolaro, F. Ambrosio and F. De Angelis, Instability of tin iodide perovskites: bulk p-doping versus surface tin oxidation, ACS Energy Lett., 2020, 5, 2787–2795 CrossRef CAS.
- M. Ozaki, Y. Katsuki, J. Liu, T. Handa, R. Nishikubo, S. Yakumaru, Y. Hashikawa, Y. Murata, T. Saito, Y. Shimakawa, Y. Kanemitsu, A. Saeki and A. Wakamiya, Solvent-coordinated tin halide complexes as purified precursors for tin-based perovskites, ACS Omega, 2017, 2, 7016–7021 CrossRef CAS PubMed.
- Y. Kanemitsu, A. Wakamiya, T. Nakamura, T. Handa and R. Murdey, Materials chemistry approach for efficient lead-free tin halide perovskite solar cells, ACS Appl. Electron. Mater., 2020, 2, 3794–3804 CrossRef.
- N. K. Tailor, M. Abdi-Jalebi, V. Gupta, H. Hu, M. I. Dar, G. Li and S. Satapathi, J. Mater. Chem. A, 2020, 8, 21356–21386 RSC.
- F. Huang, M. Li, P. Siffalovic, G. Cao and J. Tian, Energy Environ. Sci., 2019, 12, 518–549 RSC.
- M. Jung, S. G. Ji, G. Kim and S. Il Seok, Chem. Soc. Rev., 2019, 48, 2011–2038 RSC.
- M. I. Saidaminov, I. Spanopoulos, J. Abed, W. Ke, J. Wicks, M. G. Kanatzidis and E. H. Sargent, ACS Energy Lett., 2020, 5, 1153–1155 CrossRef CAS.
- J. Pascual, G. Nasti, M. H. Aldamasy, J. A. Smith, M. Flatken, N. Phung, D. Di Girolamo, S.-H. Turren-Cruz, M. Li, A. Dallmann, R. Avolio and A. Abate, Origin of Sn(II) oxidation in tin halide perovskites, Mater. Adv., 2020, 1, 1066–1070 RSC.
- A. Monga, S. Bagchi and A. Sharma, New J. Chem., 2018, 42, 1551–1576 RSC.
- K. Mislow, T. Simmons, J. T. Melillo and A. L. Ternay, The Hydrogen Chloride-Catalyzed Racemization of Sulfoxides, J. Am. Chem. Soc., 1964, 86, 1452–1453 CrossRef CAS.
- D. Di Girolamo, J. Pascual, M. H. Aldamasy, Z. Iqbal, G. Li, E. Radicchi, M. Li, S. H. Turren-Cruz, G. Nasti, A. Dallmann, F. De Angelis and A. Abate, Solvents for Processing Stable Tin Halide Perovskites, ACS Energy Lett., 2021, 959–968 CrossRef CAS.
- G. Grancini, S. Marras, M. Prato, C. Giannini, C. Quarti, F. De Angelis, M. De Bastiani, G. E. Eperon, H. J. Snaith, L. Manna and A. Petrozza, The impact of the crystallization processes on the structural and optical properties of hybrid perovskite films for photovoltaics, J. Phys. Chem. Lett., 2014, 5, 3836–3842 CrossRef CAS PubMed.
- Y. Yang, H. Lu, S. Feng, L. Yang, H. Dong, J. Wang, C. Tian, L. Li, H. Lu, J. Jeong, S. M. Zakeeruddin, Y. Liu, M. Grätzel and A. Hagfeldt, Modulation of perovskite crystallization processes towards highly efficient and stable perovskite solar cells with MXene quantum dot-modified SnO2, Energy Environ. Sci., 2021, 14, 3447–3454 RSC.
- Y. Xu, M. Wang, Y. Lei, Z. Ci and Z. Jin, Adv. Energy Mater., 2020, 10, 2002558 CrossRef CAS.
- N. Alhazmi, E. Pineda, J. Rawle, J. R. Howse and A. D. F. Dunbar, Perovskite Crystallization Dynamics during Spin-Casting: An in Situ Wide-Angle X-ray Scattering Study, ACS Appl. Energy Mater., 2020, 3, 6155–6164 CrossRef CAS PubMed.
- L. Kuai, J. Li, Y. Li, Y. Wang, P. Li, Y. Qin, T. Song, Y. Yang, Z. Chen, X. Gao and B. Sun, Revealing Crystallization Dynamics and the Compositional Control Mechanism of 2D Perovskite Film Growth by in Situ Synchrotron-Based GIXRD, ACS Energy Lett., 2020, 5, 8–16 CrossRef CAS.
- J. Dong, S. Shao, S. Kahmann, A. J. Rommens, D. Hermida-Merino, G. H. ten Brink, M. A. Loi and G. Portale, Mechanism of Crystal Formation in Ruddlesden–Popper Sn-Based Perovskites, Adv. Funct. Mater., 2020, 30, 2001294 CrossRef CAS.
- A. Krishna, S. Gottis, M. K. Nazeeruddin and F. Sauvage, Mixed Dimensional 2D/3D Hybrid Perovskite Absorbers: The Future of Perovskite Solar Cells?, Adv. Funct. Mater., 2019, 29, 1–20 CrossRef.
- D. H. Cao, C. C. Stoumpos, T. Yokoyama, J. L. Logsdon, T. Bin Song, O. K. Farha, M. R. Wasielewski, J. T. Hupp and M. G. Kanatzidis, Thin Films and Solar Cells Based on Semiconducting Two-Dimensional Ruddlesden-Popper (CH3(CH2)3NH3)2(CH3NH3)n−1SnnI3n+1 Perovskites, ACS Energy Lett., 2017, 2, 982–990 CrossRef CAS.
- L. Lanzetta, N. Aristidou and S. A. Haque, J. Phys. Chem. Lett., 2020, 11, 574–585 CrossRef CAS PubMed.
- M. Awais, R. L. Kirsch, V. Yeddu and M. I. Saidaminov, ACS Mater. Lett., 2021, 3, 299–307 CrossRef CAS.
- D. Meggiolaro, D. Ricciarelli, A. A. Alasmari, F. A. S. Alasmary and F. De Angelis, Tin versus Lead Redox Chemistry Modulates Charge Trapping and Self-Doping in Tin/Lead Iodide Perovskites, J. Phys. Chem. Lett., 2020, 11, 3546–3556 CrossRef CAS PubMed.
- R. S. Drago, J. Phys. Chem., 1958, 62, 353–357 CrossRef CAS.
- M. H. Kumar, S. Dharani, W. L. Leong, P. P. Boix, R. R. Prabhakar, T. Baikie, C. Shi, H. Ding, R. Ramesh, M. Asta, M. Graetzel, S. G. Mhaisalkar and N. Mathews, Lead-free halide perovskite solar cells with high photocurrents realized through vacancy modulation, Adv. Mater., 2014, 26, 7122–7127 CrossRef CAS PubMed.
- T. M. Koh, T. Krishnamoorthy, N. Yantara, C. Shi, W. L. Leong, P. P. Boix, A. C. Grimsdale, S. G. Mhaisalkar and N. Mathews, Formamidinium tin-based perovskite with low Eg for photovoltaic applications, J. Mater. Chem. A, 2015, 3, 14996–15000 RSC.
- C. Hartmann, S. Gupta, T. Bendikov, X. Kozina, T. Kunze, R. Félix, G. Hodes, R. G. Wilks, D. Cahen and M. Bär, Impact of SnF2 Addition on the Chemical and Electronic Surface Structure of CsSnBr3, ACS Appl. Mater. Interfaces, 2020, 12, 12353–12361 CrossRef CAS PubMed.
- P. Zhu, C. Chen, S. Gu, R. Lin and J. Zhu, CsSnI3 Solar Cells via an Evaporation-Assisted Solution Method, Sol. RRL, 2018, 2, 1700224 CrossRef.
- X. Liu, Y. Wang, T. Wu, X. He, X. Meng, J. Barbaud, H. Chen, H. Segawa, X. Yang and L. Han, Efficient and stable tin perovskite solar cells enabled by amorphous-polycrystalline structure, Nat. Commun., 2020, 11, 1–7 Search PubMed.
- J. H. Heo, J. Kim, H. Kim, S. H. Moon, S. H. Im and K. H. Hong, Roles of SnX2 (X = F, Cl, Br) Additives in Tin-Based Halide Perovskites toward Highly Efficient and Stable Lead-Free Perovskite Solar Cells, J. Phys. Chem. Lett., 2018, 9, 6024–6031 CrossRef CAS PubMed.
- T. Bin Song, T. Yokoyama, S. Aramaki and M. G. Kanatzidis, Performance enhancement of lead-free tin-based perovskite solar cells with reducing atmosphere-assisted dispersible additive, ACS Energy Lett., 2017, 2, 897–903 CrossRef.
- T. Wang and F. Yan, Chem. – Asian J., 2020, 15, 1524–1535 CrossRef CAS PubMed.
- C. Wang, F. Gu, Z. Zhao, H. Rao, Y. Qiu, Z. Cai, G. Zhan, X. Li, B. Sun, X. Yu, B. Zhao, Z. Liu, Z. Bian and C. Huang, Self-Repairing Tin-Based Perovskite Solar Cells with a Breakthrough Efficiency Over 11%, Adv. Mater., 2020, 32(31), 1907623 CrossRef CAS PubMed.
- X. He, T. Wu, X. Liu, Y. Wang, X. Meng, J. Wu, T. Noda, X. Yang, Y. Moritomo, H. Segawa and L. Han, Highly efficient tin perovskite solar cells achieved in a wide oxygen concentration range, J. Mater. Chem. A, 2020, 8, 2760–2768 RSC.
- T. Wang, Q. Tai, X. Guo, J. Cao, C. K. Liu, N. Wang, D. Shen, Y. Zhu, C. S. Lee and F. Yan, Highly Air-Stable Tin-Based Perovskite Solar Cells through Grain-Surface Protection by Gallic Acid, ACS Energy Lett., 2020, 5, 1741–1749 CrossRef CAS.
- M. E. Kayesh, T. H. Chowdhury, K. Matsuishi, R. Kaneko, S. Kazaoui, J. J. Lee, T. Noda and A. Islam, Enhanced Photovoltaic Performance of FASnI3-Based Perovskite Solar Cells with Hydrazinium Chloride Coadditive, ACS Energy Lett., 2018, 3, 1584–1589 CrossRef CAS.
- F. Gu, S. Ye, Z. Zhao, H. Rao, Z. Liu, Z. Bian and C. Huang, Improving Performance of Lead-Free Formamidinium Tin Triiodide Perovskite Solar Cells by Tin Source Purification (Solar RRL 10/2018), Sol. RRL, 2018, 2, 1870217 CrossRef.
- T. Nakamura, S. Yakumaru, M. A. Truong, K. Kim, J. Liu, S. Hu, K. Otsuka, R. Hashimoto, R. Murdey, T. Sasamori, H. Do Kim, H. Ohkita, T. Handa, Y. Kanemitsu and A. Wakamiya, Sn(IV)-free tin perovskite films realized by in situ Sn(0) nanoparticle treatment of the precursor solution, Nat. Commun., 2020, 11, 1–8 Search PubMed.
- N. Sun, W. Gao, H. Dong, Y. Liu, X. Liu, Z. Wu, L. Song, C. Ran and Y. Chen, Architecture of p-i-n Sn-Based Perovskite Solar Cells: Characteristics, Advances, and Perspectives, ACS Energy Lett., 2021, 6, 2863–2875 CrossRef CAS.
- S. Tao, I. Schmidt, G. Brocks, J. Jiang, I. Tranca, K. Meerholz and S. Olthof, Absolute energy level positions in tin- and lead-based halide perovskites, Nat. Commun., 2019, 10, 1–10 CrossRef CAS PubMed.
- N. Wang, Y. Zhou, M. G. Ju, H. F. Garces, T. Ding, S. Pang, X. C. Zeng, N. P. Padture and X. W. Sun, Heterojunction-Depleted Lead-Free Perovskite Solar Cells with Coarse-Grained B-γ-CsSnI3 Thin Films, Adv. Energy Mater., 2016, 6, 1601130 CrossRef.
- K. Hamada, R. Tanaka, M. A. Kamarudin, Q. Shen, S. Iikubo, T. Minemoto, K. Yoshino, T. Toyoda, T. Ma, D. W. Kang and S. Hayase, Enhanced Device Performance with Passivation of the TiO2 Surface Using a Carboxylic Acid Fullerene Monolayer for a SnPb Perovskite Solar Cell with a Normal Planar Structure, ACS Appl. Mater. Interfaces, 2020, 12, 17776–17782 CrossRef CAS PubMed.
- T. Yokoyama, Y. Nishitani, Y. Miyamoto, S. Kusumoto, R. Uchida, T. Matsui, K. Kawano, T. Sekiguchi and Y. Kaneko, Improving the Open-Circuit Voltage of Sn-Based Perovskite Solar Cells by Band Alignment at the Electron Transport Layer/Perovskite Layer Interface, ACS Appl. Mater. Interfaces, 2020, 12, 27131–27139 CrossRef CAS PubMed.
- W. Ke, P. Priyanka, S. Vegiraju, C. C. Stoumpos, I. Spanopoulos, C. M. M. Soe, T. J. Marks, M. C. Chen and M. G. Kanatzidis, Dopant-Free Tetrakis-Triphenylamine Hole Transporting Material for Efficient Tin-Based Perovskite Solar Cells, J. Am. Chem. Soc., 2018, 140, 388–393 CrossRef CAS PubMed.
- J. Cao, Q. Tai, P. You, G. Tang, T. Wang, N. Wang and F. Yan, Enhanced performance of tin-based perovskite solar cells induced by an ammonium hypophosphite additive, J. Mater. Chem. A, 2019, 7, 26580–26585 RSC.
- C. Liu, J. Tu, X. Hu, Z. Huang, X. Meng, J. Yang, X. Duan, L. Tan, Z. Li and Y. Chen, Enhanced Hole Transportation for Inverted Tin-Based Perovskite Solar Cells with High Performance and Stability, Adv. Funct. Mater., 2019, 29, 1808059 CrossRef.
- S. Emami, J. Martins, D. Ivanou and A. Mendes, Advanced hermetic encapsulation of perovskite solar cells: the route to commercialization, J. Mater. Chem. A, 2020, 8, 2654–2662 RSC.
- J. Li, R. Xia, W. Qi, X. Zhou, J. Cheng, Y. Chen, G. Hou, Y. Ding, Y. Li, Y. Zhao and X. Zhang, Encapsulation of perovskite solar cells for enhanced stability: Structures, materials and characterization, J. Power Sources, 2021, 485, 229313 CrossRef CAS.
-
F. Alta and E. S. Asu, Best Research-Cell Efficiencies, 2020, p. 2020.
|
This journal is © the Owner Societies 2021 |