DOI:
10.1039/D0AN00389A
(Minireview)
Analyst, 2020,
145, 4388-4397
Light-responsive nanozymes for biosensing
Received
24th February 2020
, Accepted 23rd April 2020
First published on 23rd April 2020
Abstract
Using light as an external stimulus plays a key role not only in modulating activities of nanozymes, but also in constructing efficient biosensing systems. This mini-review highlights recent advances in light-responsive nanozyme-based biosensing systems. First, we introduce the light stimulation for regulating nanozymes’ activities. Then, several strategies are presented to construct efficient photo-responsive nanozyme-based detection systems by using metal-based nanozymes, carbon-based nanozymes, and MOF-based nanozymes, respectively. Moreover, the detection mechanisms of current biosensors are discussed. Finally, we discuss the current challenges and future perspectives of this research area.
1. Introduction
Nanozymes, as emerging artificial enzymes, not only exhibit enzyme-like catalytic properties, but also have their intrinsic advantages over natural enzymes (such as low cost, high stability, and large-scale production). Furthermore, they are superior to conventional enzyme mimics in multi-functionalities and in activities that can be modulated.1–8 The latter allow us to modulate the activities of nanozymes via various strategies. Among them, light stimulation is of great interest because of its high efficiency and spatial controllability.9–14 Moreover, light stimulation is also a fascinating regulation of natural enzymes,15–17 such as DNA photolyase,18 fatty acid photodecarboxylase,19 and protochlorophyllide oxidoreductase.20 In this regard, the introduction of light-responsive functionalities will be a promising way to design new enzyme mimics (including nanozymes) for further applications.
Nanomaterials with light harnessing capacity can utilize solar energy in numerous fields,9,21–23 including photovoltaic industry,24 fuel generation,25,26 environmental protection,27etc. Most of these photoactive nanomaterials have the photo-induced electron transfer (PET) ability,28,29 which is a fundamental process of photocatalytic reactions. Therefore, modulating the interactions between substrates and active electrons can produce on-demand effects.30–32 The currently developed light-responsive nanozymes can be mainly classified into two types: for type I, the photocatalytic properties originate from the nanomaterials themselves, which could generate reactive oxygen species (ROS) by absorbing light and subsequently oxidizing enzymatic substrates; for type II, the photocatalytic activities are entirely from functional photoactive molecules which are modified on the nanomaterials. For instance, photoisomerization,33 photo-induced pH changes,34 and photothermal effects35 can impact the enzyme-like activities of non-photoactive nanozymes. By taking advantages of the two types of photo-responsive nanozymes, lots of applications, including biosensing, degradation of organic pollutants,36–38 modification of DNA,39,40 and anti-bacteria,41–44 have been developed. With the rapid development of this area, two recent comprehensive reviews45,46 on light-activated nanozymes have been published, whereas none of them focused on the strategies to construct photoactive nanozyme-based biosensors.
To highlight the strategy of using light-activated nanozymes for building biosensing systems, in this mini-review, we first introduced the activity modulation of nanozymes under light stimulation. Then, several types of nanozymes with light-induced enzyme-like activities were systematically presented for developing the photoactive sensing systems. Subsequently, the mechanisms of detection towards the above-mentioned sensing systems were summarized. Finally, we discuss the current challenges of the photoactive nanozymes and further propose several perspectives of light-responsive biosensing systems for future applications.
2. Light stimulation for modulating the activities of nanozymes
As mentioned above, there are two types of light-responsive nanozymes. For type I, ROS, such as superoxide anion (˙O2−), hydroxyl radical (˙OH), singlet oxygen (1O2), and photo-generated holes (h+), is involved during the photocatalytic reactions. The main advantage of photocatalytic nanozymes is their ability to catalyze challenging reactions that hardly proceed without light. However, the disadvantage of such nanozymes is the low efficiency under the sunlight, and thus a powerful light source such as a Xe lamp is needed. For type II, since these nanozymes themselves are non-photocatalytically active, photoactive molecules are needed to facilitate the photocatalysis. Unlike photocatalytic reactions, minimal side reactions are an advantage of this type of nanozymes because of the generation of less ROS, which is suitable for the construction of reversible reaction systems. Meanwhile, the limited amount and types of photoactive molecules are the main drawbacks of this kind of nanozymes. These two types of light-stimulated modulations including photocatalytic and non-photocatalytic are discussed in this section.
2.1. Photocatalytic modulation
Improving the production of ROS is a fundamental method for accelerating photocatalytic reactions.47–49 To this end, improving the light absorption is a promising strategy to enhance the ROS yield. For example, although carbon-based photosensitizers50 are one kind of attractive photoactive nanozymes, low efficiency and lack of rational design were the bottlenecks for the construction of highly efficient photo-oxidative nanozymes. To rationally design carbon-based photoactive nanozymes, in 2018, the Wu group synthesized a series of nitrogen-doped carbon dots (C-dots) and studied the correlation between photo-oxidation activity and phosphorescence quantum yield.41 As shown in Fig. 1A, the C-dots exhibited enzyme-like activity under light irradiation and the photo-generated ROS was used for antimicrobial chemotherapy. Further experimental results showed that C-dots exhibit better performance for the activation of oxygen than other carbon-based nanomaterials as well as dyes of phloxine B (PB) and rose bengal (RB) (Fig. 1B). To provide an insight into the production mechanism of singlet oxygen, Fig. 1C shows the correlation between fluorescence and phosphorescence of four types of C-dots. In addition, the oxidation efficiency of 3,3′,5,5′-tetramethylbenzidine (TMB) indicated that the C-dots had a positive correlation between the phosphorescence quantum yield and the photosensitizing ability (Fig. 1D).
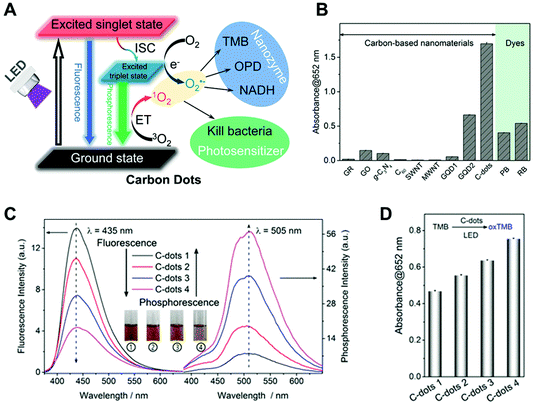 |
| Fig. 1 (A) Schematic illustration of photo-sensitized oxygen activation with C-dot nanozymes for photodynamic antimicrobial chemotherapy. (B) Comparison of the oxygen activation performance of various carbon-based nanomaterials and two molecular photosensitizers (PB and RB) for TMB oxidation under light. All the materials were of 5 mg L−1. (C) Fluorescence and phosphorescence (in the PVA matrix) spectra of four types of C-dots. Inset shows the solution of C-dots at different temperatures of synthesis. (D) TMB photo-oxidation efficiencies of the four C-dots. Reproduced from ref. 41 with permission from the American Chemical Society, copyright 2018. | |
In addition, the long excited-state lifetime is another important factor of light-responsive nanozymes for increasing the yield of ROS. In this regard, the Liu group used Mn(II) to communicate with C-dots, which promoted the catalysis of C-dots for TMB oxidation at neutral pH in the presence of singlet oxygen produced via photosensitization (Fig. 2A).51 Mn(II) can be oxidized to Mn(III) by the photo-generated singlet oxygen, enhancing the electron transfer upon light irradiation. Furthermore, compared with several other metal ions, only Mn(II) could enhance the oxidation of TMB in a physiological buffer (Fig. 2B). This Mn-mediated oxidation method solved a long-standing problem for the nanozymes in lacking the activity at physiological pH.
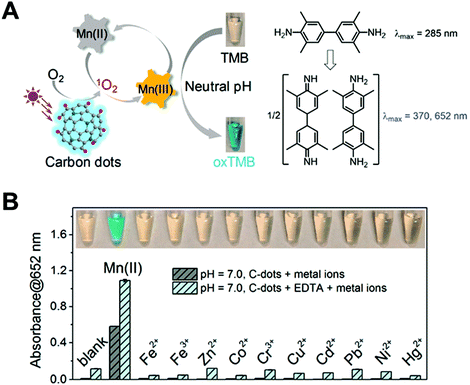 |
| Fig. 2 (A) Schematic illustration of Mn(II) enhancing the photo-oxidase activity of C-dots at neutral pH. (B) Among the tested metals (1 mM each), without or with 1 mM EDTA, only Mn(II) enhanced oxidation. Reproduced from ref. 51 with permission from the American Chemical Society, copyright 2019. | |
Hydroxyl radical is another kind of ROS in photocatalytic reactions. For example, the Xia group found gold nanoparticles (Au NPs) exhibited peroxidase-like activity under visible-light irradiation.52 Based on the unique localized surface plasmon resonance (LSPR) property of Au NPs, they revealed that upon LSPR excitation, hot carriers (electrons and holes) were activated on the surface of Au NPs. The injection of hot electrons into the H2O2 molecule could produce more hydroxyl radicals, leading to an enhanced enzyme-like performance. Similarly, superoxide anion can be generated by the activation of oxygen through the injection of hot electrons. Apart from Au NPs, many photocatalytic nanozymes including g-C3N4,53 Au–Pd-Bi2WO6,54 and β-In2S3
55 rely on the photo-generated superoxide anion for the oxidation of substrates.
2.2. Non-photocatalytic modulation
Unlike the photocatalytic regulation, non-photocatalytic modulation affects nanozymes’ activity in the absence of ROS. As shown in Table 1, photoisomerization,56 photo-induced pH change,57 and photothermal effect58 are the currently developed strategies for activity regulation. In 2017, the Qu group reported that a photoactive molecule of azobenzene (Azo) with the ability of light-driven isomerization reversibly regulated the catalase-like activity of Au nanoparticles (NPs) (Fig. 3A).59 They used Azo-modified mesoporous silica as a host to encapsulate Au NPs and cyclodextrin (CD) (Au–Si-Azo). The trans conformations of Azo in Au–Si-Azo led to catalytic site blockage by CD, and subsequently inhibited the catalytic activity. As shown in Fig. 3B, under the irradiation of UV light, azo undergoes the isomerization from trans to cis conformation, which enhanced the enzyme-like activity of Au NPs. However, after the visible light irradiation, transformation of azo from cis to trans conformation took place, CD was unable to be released and the catalase-like activity of Au NPs was inhibited (Fig. 3C). Inspired by this, the Qu group further developed an azo-modified Pd nanozyme and controlled its catalytic activity by the light-induced isomerization.60 Likewise, the Prins group reported that the hydrolysis activity of Au NPs can be reversibly regulated by switching the light source.61 The Au NPs were functionalized with a monolayer of C9-thiol-based molecules to have a higher affinity towards the trans isomer of the photoactive molecule. Therefore, the photoactive molecule with the trans conformation inhibited the absorption of the substrate 2-hydroxypropyl-4-nitrophenylphosphate (HPNPP) and thus decreased the hydrolysis activity. Upon irradiation at 365 nm, the trans isomer of photoactive molecule transformed into cis isomer, lowering their affinity on the surface of Au NPs. Meanwhile, the transphosphorylation rate of HPNPP was upregulated.
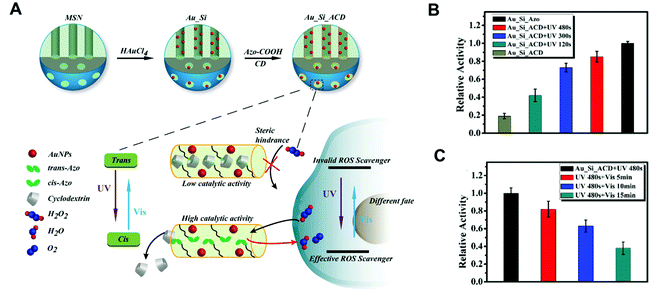 |
| Fig. 3 (A) Synthetic procedure for Au_Si_ACD. The UV and visible light reversibly regulate the catalytic activity of Au_Si_ACD by the trans–cis photoisomerization of Azo molecules to control the host–guest interactions between Azo and CD. The new nanozymes can act as a controllable ROS scavenger in cells with different catalytic activities. (B) Au_Si_ACD was irradiated with UV light for 0, 120, 300 and 480 s and then incubated with H2O2 for 60 min. The catalase-like activity was normalized to that of Au_Si_Azo sample. (C) After irradiation with UV light for 480 s, Au_Si_ACD was exposed under visible light for 5, 10 and 15 min. The catalase-like activity was normalized. Reproduced from ref. 59 with permission from John Wiley and Sons, copyright 2017. | |
Table 1 Non-photocatalytic modulations of light-responsive nanozymes
Modulator |
Mechanism |
Nanozymes |
Activity |
Ref. |
Azobenzene |
Photoisomerization |
Au–Si-Azo |
Catalase-like activity |
59
|
Azobenzene |
Photoisomerization |
CASP |
Bioorthogonal catalysis |
60
|
Azobenzene |
Photoisomerization |
Au NP |
Transphosphorylation |
61
|
2-Nitrobenzaldehyde |
Photoacid effect |
Nanoceria |
Oxidase-like activity |
63
|
2-Nitrobenzaldehyde |
Photoacid effect |
MoS2 |
Gram-selective antimicrobial |
64
|
Au NPs |
Photothermal effect |
Au@HCNs |
ROS production |
65
|
Besides modifying with photo-isomerized molecules, the combination with a photoacid molecule is another way to fabricate photo-responsive nanozymes. A photoacid molecule can induce pH change during the light irradiation, further regulating the pH-dependent activity of nanozymes. In this regard, a typical photoacid molecule of 2-nitrobenzaldehyde (2-NBA)62 was applied to activate the enzyme-like activity of nanoceria63 nanozyme (Fig. 4A) and increase the antimicrobial64 activity of MoS2 nanozyme (Fig. 4B). In addition, nanozymes’ activity could also be modulated by the photothermal effect. For instance, Au NPs could improve the production of photo-generated ROS by increasing the photothermal effect, which benefited cancer therapy (Fig. 4C).65
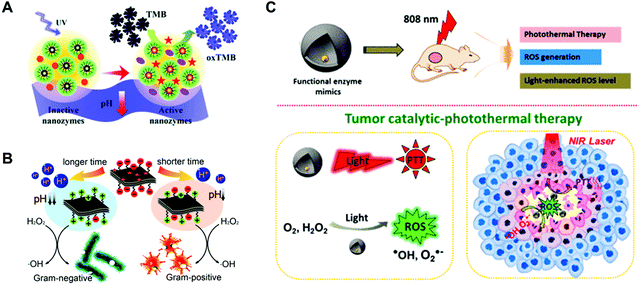 |
| Fig. 4 Schematic illustrations of controllable oxidase-like activity of dextran-decorated nanoceria based on the photo-induced proton release (A), light-modulated nanozyme of MoS2 for a Gram-selective antimicrobial (B), and the catalytic–photothermal tumor therapy with yolk–shell Gold@Carbon nanozymes (C). (A) Reproduced from ref. 63 with permission from the Royal Society of Chemistry, copyright 2018. (B) Reproduced from ref. 64 with permission from the American Chemical Society, copyright 2018. (C) Reproduced from ref. 65 with permission from the American Chemical Society, copyright 2018. | |
3. Construction of biosensing systems using light-responsive nanozymes
Using a light-controllable system for biosensing is a promising analytical method in recent years.66–69 To date, various photoactive materials have been applied for the detection of many analytes, such as ions,70 thiols,71 and amino acids.72 These photo-responsive systems have several advantages. First, the pre-mixture of an inactive sensor and an analyte will not affect the performance of their active state, thus avoiding the overestimation of detection. For instance, the Hecht group has developed a methodology for the detection of amines using specific diarylethenes (DAEs), which can be activated not only on the defined areas by using light irradiation, but also in the desired point of time.73 Therefore, the influence of local concentration can be avoided. Second, the light-controllable detection system possesses high sensitivity and low background since the separation of signal excitation and detection, such as the analytical method of photoelectrochemistry.74 Third, the photoactive system is of great advantage for the detection upon cellular context due to the ready conversion from an inert to active state, the short half-life time, and the self-quenching ability. For instance, the Spitale group introduced a novel strategy for measuring the RNA solvent accessibility called light-activated structural examination of RNA (LASER). Due to the exquisite sensitivity of LASER probing, the understanding of the transition structure of RNA is more accurate and faster.75 With this in mind, in this section, the light-responsive nanozymes for the detection of biomolecules were systematically summarized and discussed based on the different types of nanozymes, including metal-, carbon-, and MOF-based ones.
3.1. Using metal-based nanozymes
3.1.1. Metal halides.
In 2014, chitosan (CS)-modified silver halide NPs (AgX, X = Cl, Br, I) with light-responsive oxidase-like activities were developed by the Wang group for cancer cell detection.76 A 500 W Xe lamp with a cut-off filter (λ ≥ 420 nm) was applied as the irradiation source. CS-AgX NPs could oxidize three colorless enzymatic substrates (i.e., 3,3′,5,5′-tetramethylbenzidine (TMB), o-phenylenediamine (OPD), and 2,2′-azino-bis(3-ethylbenzothiazoline-6-sulfonic acid (ABTS)) to their colored products upon irradiation (Fig. 5A). Kinetic studies of oxidizing TMB suggested a ping-pong catalytic mechanism of CS-AgX NPs. Moreover, the excellent oxidase-like activity was retained over a broad pH range (3.0–7.0) (Fig. 5B). In addition, mechanistic studies indicated that the photo-generated ˙O2− and holes were the reactive intermediates for oxidizing TMB. Subsequently, CS-AgI NPs were modified with folic acid (FA) via the formation of an amide bond between FA and CS. The as-prepared FA-CS-AgI was employed for cancer cell detections as folate receptors were usually overexpressed on cancer cell membranes (Fig. 5C). Therefore, different types of cancer cells were detected according to their different numbers of folate receptors (Fig. 5D).
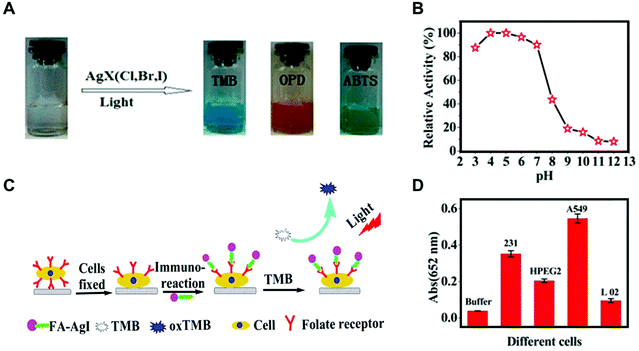 |
| Fig. 5 (A) Color evolution of TMB, OPD, and ABTS oxidation by visible light-stimulated CS-AgX (X = Cl, Br, I). (B) Relative catalytic activity of the CS-AgI NPs under visible light irradiation (λ ≥ 420 nm) at a range of different solution pH values. (C) Proposed process of cancer cell detection by using FA-CS-AgI. (D) Response of different cells using FA-CS-AgI to TMB under visible light irradiation. The error bars represent the standard deviation of five measurements. Reproduced from ref. 76 with permission from the American Chemical Society, copyright 2014. | |
3.1.2. Metal.
Because of the unique optical property of noble-metal NPs, several noble metal-based light-responsive sensing systems were explored. In 2015, the Wang group used bovine serum albumin (BSA) to stabilize Au nanoclusters to control their size-dependent optical properties.77 With the help of BSA, the obtained BSA-Au NCs exhibited excellent oxidase-like activities under visible light irradiation. Since trypsin can digest BSA and decrease the catalytic activity of BSA-Au-NCs, a facile colorimetric sensing system for trypsin was developed (Fig. 6A). The photoactive trypsin sensing platform showed excellent selectivity and a limit detection of 0.6 μg mL−1 (Fig. 6B and 6C). Inspired by this, the Wu group developed an ascorbic acid (AA)-regulated Ag3PO4 nanozyme for multiple biological detections.78 Ag+ on the surface of Ag3PO4 NPs can be reduced by AA to form a Ag0/Ag3PO4 heterostructure, which in turn enhanced its oxidase-like activities due to the effect of surface plasmon resonance (SPR). Therefore, AA and AA-related biomolecules were detected using this photo-responsive sensing system.
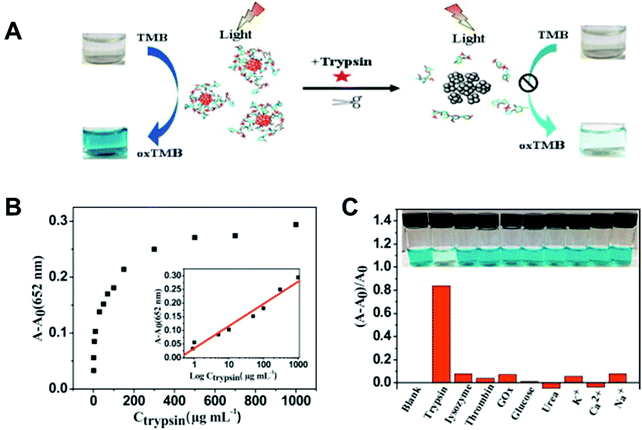 |
| Fig. 6 (A) Schematic representation of the colorimetric sensing mechanism for trypsin based on the enzyme-like activity of BSA–Au NCs. (B) Change in absorption and the linear relationship (inset shows the curve) between (A − A0) of oxTMB at 652 nm and the concentration of trypsin. (C) The effect of different substances on the colorimetric detection of trypsin. Reproduced from ref. 77 with permission from Elsevier, copyright 2015. | |
3.1.3. Metal oxides.
Besides the noble metal nanomaterials, metal oxide nanomaterials such as TiO2 NPs could be used for photoactive sensing as well. In 2015, the Wang group reported that coordinating CA on TiO2 NPs79 extended their absorption range from ultraviolet to visible, which activated the oxidase-like activity of TiO2 NPs under visible light stimulation (Fig. 7A). Upon light irradiation, TiO2-CA NPs exhibited a typical Michaelis–Menten kinetics, and the activity could be easily controlled by switching “on/off” state of light. In this way, CA correlated biomolecules including alkaline phosphatase (ALP) and ALP inhibitor were effectively detected. Later, another photoactive peroxidase mimic was constructed by using dihydroxyphenylalanine (DOPA) to coordinate with TiO2 NPs80 (Fig. 7B). The DOPA-TiO2 nanozyme produced more reactive species than the non-coordinated TiO2 NPs under visible light irradiation. Subsequently, since tyrosinase (TYR) could catalyze tyrosine to generate DOPA, the TiO2 NP-based photoactive sensor was applied for TYR detection.
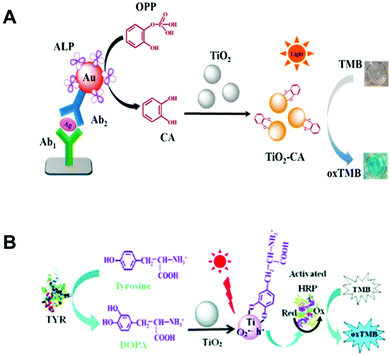 |
| Fig. 7 (A) Proposed immunodetection process for mouse IgG by coupling the in situ-generated photo-responsive nanozyme of CA−TiO2. (B) Schematic illustration of the colorimetric sensing mechanism for TYR based on the photoactive sensor. (A) Reproduced from ref. 79 with permission from the American Chemical Society, copyright 2015. (B) Reproduced from ref. 80 with permission from the Royal Society of Chemistry, copyright 2017. | |
3.2. Using carbon-based nanozymes
3.2.1. GO.
Another typical photoactive nanozyme was a carbon-based nanomaterial. In 2014, chitosan functional graphene oxide (CS-GO)81 was shown to exhibit oxidase-like activity under visible light irradiation (Fig. 8A). Kinetics and mechanistic studies indicated that the CS-GO nanozyme possessed higher affinity towards TMB than HRP and the photogenerated holes (h+) represented the active species in TMB oxidation. Interestingly, the photo-responsive activity of CS-GO can be inhibited through the aggregation of GO, which was induced by the interaction between CS and concanavalin A (Con A). Meanwhile, glucose could compete against CS for interacting with Con A, which led to the recovery of the enzyme-like activity. Therefore, a selective and sensitive photo-stimulated sensing system was developed by CS-GO nanozymes for the detection of glucose (Fig. 8B).
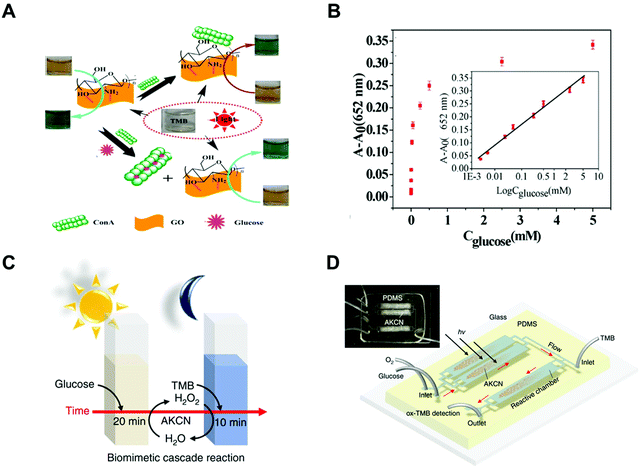 |
| Fig. 8 (A) Fabrication process and detection principle of the system to detect glucose. (B) Linear relationship between (A − A0) of oxTMB at 652 nm and the concentration of glucose. (C) Scheme of the cascade reaction with continuous O2-purging in a batch mode. (D) Scheme of the cascade reaction in a microfluidic device and the actual device image (inset). (A and B) Reproduced from ref. 81 with permission from the American Chemical Society, copyright 2014. (C and D) Reproduced from ref. 83 with permission from Springer Nature, copyright 2019. | |
3.2.2. C3N4.
To further functionalize the carbon-based nanozymes, a photoactive sensing system by combining acid phosphatase (ACP) with C3N4 was established.82 ACP can hydrolyze pyrophosphate (PPi) to liberate copper ion (Cu2+), which subsequently triggers C3N4 nanosheets to generate the enzyme-like activity under light irradiation. Switching “on/off” state of light can easily control the activity of Cu2+/C3N4. Moreover, ACP can be effectively detected via this photoactive sensing system.
Besides, another carbon-based photoactive sensing system has been developed by the Choi group in 2019.83 The C3N4 nanozyme modified with KOH and KCl possessed excellent glucose oxidase (GOx)-like activity under the light stimulation, which could catalyze glucose to produce H2O2 (Fig. 8C). With the help of in situ generated H2O2, the C3N4 nanozyme oxidized TMB via the HRP-like activity under dark conditions. By taking such advantages of the C3N4 nanozyme, a cascade photoactive biosensor was developed for the detection of glucose. Moreover, using a microfluidic device could highly accelerate such biosensing processes, enabling the real-time monitoring of glucose with a detection limit of 0.8 μM in 30 s (Fig. 8D).
3.3 Using MOF-based nanozymes
To overcome the limited absorbance of photoactive nanomaterials under visible light, recently, a photo-sensitized MOF (PSMOF) has been reported as an oxidase mimic for biomedical applications by our group (Fig. 9A).84 An organic dye of Ru(bpy)32+ with a stronger visible-light absorption85 was used as a PS linker for the construction of PSMOF. The PSMOF showed excellent oxidase-like activity under light irradiation, and the enzyme-like activity could be controlled by switching light on and off. Mechanistic studies indicated that the dissolved oxygen could be activated by the PSMOF under light irradiation to form ROS including ˙O2− and ˙OH. Based on the production of ROS, the PSMOF nanozyme was used to detect the reducing molecule of glutathione in both normal and cancer cells (Fig. 9B).
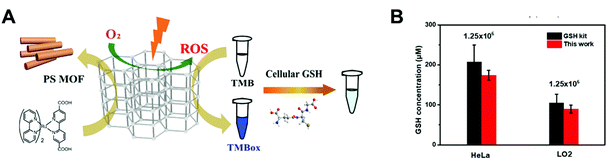 |
| Fig. 9 (A) Schematic illustration of the cellular GSH detection by using a PSMOF-based photoactive sensor. (B) Qualitative evaluation of cellular GSH levels with the developed sensor and a GSH kit. The number marked on the histogram is the cell density. Each error bar shows the standard deviation of three independent measurements. Reproduced from ref. 84 with permission from the American Chemical Society, copyright 2019. | |
4. Detection mechanisms
As mentioned above, most of the photoactive sensing systems were based on the following two sensing mechanisms. One was that the analytes affect the activity of the photo-responsive nanozymes by non-ROS-involved interactions. Therefore, the detection is mainly based on the effects of analytes on the photoactivity of nanozymes. For example, ACP could catalyze the hydrolysis of PPi to liberate Cu2+ and the released Cu2+ in turn enhances the photo-responsive enzyme-like activity of C3N4 nanozyme. In this way, ACP could be detected by monitoring such variations of enzyme-like activity and the detection limit was 8.8 × 10−3 U L−1.82 On the other hand, due to the correlation between photoactivity and light absorption ability, analytes with the ability of affecting the light absorbance of nanozymes could be detected. For instance, BSA-stabilized Au NCs endowed themselves with the ability of light absorption and exhibited enzyme-like activities under visible light irradiation. As the TYR could decrease the light absorption ability of BSA–Au NCs through the digestion of BSA, TYR could be detected by measuring the reduction of their enzyme-like activities.80
The other principle of sensing is based on ROS-mediated interactions, meaning the analytes need to be reacted with photo-generated ROS. Due to the strong oxidizing ability of ROS, biomolecules with reductive activity could be detected such as glutathione (GSH). However, the selectivity of this reaction is limited. For example, the reductive molecules of ascorbic acid (AA) and cysteine could interfere the detection of GSH.84 Therefore, constructing more specific ROS-involved photosensing systems is of great interest. Recently, glucose could be oxidized to generate hydrogen peroxide by the modified C3N4 nanozyme under light irradiation, demonstrating the glucose oxidase-like activity of C3N4 nanozymes. Since the modified C3N4 nanozyme also exhibited peroxidase-like activity, glucose could be detected by exploring the C3N4 nanozyme-catalyzed GOx- and peroxidase-like cascade reactions.83
5. Conclusions and future perspectives
In this mini-review, we summarize the recent progress in photoactive nanozyme-based biosensors, including enzyme-like activity regulation, types of photo-responsive biosensors, and mechanism of detection. Although these effective and controllable biosensors had been demonstrated for multiple biomolecule detections, most of these studies were proof-of-concept. Therefore, there is still plenty of room for future development. Several possible opportunities for future improvements have been given as follows. (1) More reaction types of photoactive nanozymes are needed. To date, almost all photoactive nanozyme-based biosensors rely on the oxidase- and peroxidase-like activities, limiting the range of analytes of interest. Since light stimulation is extensively applied in chemical synthesis and biomedical applications, searching for new kinds of photoactive enzyme-like activities for constructing biosensing systems is reasonable and attractive. (2) Using photoactive nanozyme-based sensors for in vivo detection. Nanozyme-based in vitro sensing systems had been successfully developed, while none of the photo-responsive nanozymes were applied for in vivo detection yet. (3) Sensing systems with high selectivity need to be further improved for detection. Substrate specificity is a long-standing challenge for designing nanozymes. Therefore, developing photoactive nanozymes with high specificity for analytes is a promising direction for the construction of biosensors. (4) Developing controllable photoactive sensing systems is attractive for portable detection. The most fascinating feature of light stimulation is that it is controllable, and is useful to control the detection process. However, building controllable detection systems by using photo-responsive nanozymes are limited and difficult. (5) Exploring more nanomaterials with photoactive enzyme-like activity for the development of biosensing systems. The photo-responsive nanozymes are far less than traditional nanozymes and photocatalytic nanomaterials both in quantities and types. We expect that this review will benefit the modulation of light-activated nanozymes and inspire the exploration of more functional photoactive biosensors in future.
Conflicts of interest
There are no conflicts to declare.
Acknowledgements
This work was supported by the National Natural Science Foundation of China (21722503 and 21874067), the China Postdoctoral Science Foundation (2019TQ0144 and 2019M661786), the PAPD Program, the Open Funds of the State Key Laboratory of Analytical Chemistry for Life Science (SKLACLS1704), the Open Funds of the State Key Laboratory of Coordination Chemistry (SKLCC1819), and the Fundamental Research Funds for the Central Universities (14380145).
References
- L. Gao, J. Zhuang, L. Nie, J. Zhang, Y. Zhang, N. Gu, T. Wang, J. Feng, D. Yang, S. Perrett and X. Yan, Nat. Nanotechnol., 2007, 2, 577–583 CrossRef CAS PubMed.
- H. Wei and E. Wang, Chem. Soc. Rev., 2013, 42, 6060–6093 RSC.
- J. Wu, X. Wang, Q. Wang, Z. Lou, S. Li, Y. Zhu, L. Qin and H. Wei, Chem. Soc. Rev., 2019, 48, 1004–1076 RSC.
- D. Jiang, D. Ni, Z. T. Rosenkrans, P. Huang, X. Yan and W. Cai, Chem. Soc. Rev., 2019, 48, 3683–3704 RSC.
- Y. Huang, J. Ren and X. Qu, Chem. Rev., 2019, 119, 4357–4412 CrossRef CAS PubMed.
- J. W. Liu and Y. Lu, J. Am. Chem. Soc., 2003, 125, 6642–6643 CrossRef CAS PubMed.
- Y. Song, K. Qu, C. Zhao, J. Ren and X. Qu, Adv. Mater., 2010, 22, 2206–2210 CrossRef CAS PubMed.
- Y. Lin, J. Ren and X. Qu, Acc. Chem. Res., 2014, 47, 1097–1105 CrossRef CAS PubMed.
- J. C. Colmenares and R. Luque, Chem. Soc. Rev., 2014, 43, 765–778 RSC.
- M. A. Fox and M. T. Dulay, Chem. Rev., 1993, 93, 341–357 CrossRef CAS.
- M. Anpo and M. Takeuchi, J. Catal., 2003, 216, 505–516 CrossRef CAS.
- H. Yan, J. Yang, G. Ma, G. Wu, X. Zong, Z. Lei, J. Shi and C. Li, J. Catal., 2009, 266, 165–168 CrossRef CAS.
- Y. Fu, D. Sun, Y. Chen, R. Huang, Z. Ding, X. Fu and Z. Li, Angew. Chem., Int. Ed., 2012, 51, 3364–3367 CrossRef CAS PubMed.
- B. Jeong and A. Gutowska, Trends Biotechnol., 2002, 20, 305–311 CrossRef CAS PubMed.
- H. Kuhn, Nature, 1980, 283, 587–589 CrossRef CAS PubMed.
- C. Tommos and G. T. Babcock, Acc. Chem. Res., 1998, 31, 18–25 CrossRef CAS.
- B. Schierling, A.-J. Noel, W. Wende, L. T. Hien, E. Volkov, E. Kubareva, T. Oretskaya, M. Kokkinidis, A. Roempp, B. Spengler and A. Pingoud, Proc. Natl. Acad. Sci. U. S. A., 2010, 107, 1361–1366 CrossRef CAS PubMed.
- T. Carell, L. T. Burgdorf, L. M. Kundu and M. Cichon, Curr. Opin. Chem. Biol., 2001, 5, 491–498 CrossRef CAS PubMed.
- J. Xu, Y. Hu, J. Fan, M. Arkin, D. Li, Y. Peng, W. Xu, X. Lin and Q. Wu, Angew. Chem., Int. Ed., 2019, 58, 8474–8478 CrossRef CAS PubMed.
- D. J. Heyes, C. N. Hunter, I. H. M. van Stokkum, R. van Grondelle and M. L. Groot, Nat. Struct. Biol., 2003, 10, 491–492 CrossRef CAS PubMed.
- N. M. Idris, M. K. G. Jayakumar, A. Bansal and Y. Zhang, Chem. Soc. Rev., 2015, 44, 1449–1478 RSC.
- S. Sarina, H. Zhu, E. Jaatinen, Q. Xiao, H. Liu, J. Jia, C. Chen and J. Zhao, J. Am. Chem. Soc., 2013, 135, 5793–5801 CrossRef CAS PubMed.
- F. Wang, C. Li, H. Chen, R. Jiang, L.-D. Sun, Q. Li, J. Wang, J. C. Yu and C.-H. Yan, J. Am. Chem. Soc., 2013, 135, 5588–5601 CrossRef CAS PubMed.
- J. A. Gow and C. D. Manning, IEEE Proc.: Electr. Power Appl., 1999, 146, 193–200 CrossRef.
- Q. Xiang, B. Cheng and J. Yu, Angew. Chem., Int. Ed., 2015, 54, 11350–11366 CrossRef CAS PubMed.
- Y. Ma, X. Wang, Y. Jia, X. Chen, H. Han and C. Li, Chem. Rev., 2014, 114, 9987–10043 CrossRef CAS PubMed.
- D. Chatterjee and S. Dasgupta, J. Photochem. Photobiol., C, 2005, 6, 186–205 CrossRef CAS.
- X. Liu, L. Jiang, J. Li, L. Wang, Y. Yu, Q. Zhou, X. Lv, W. Gong, Y. Lu and J. Wang, J. Am. Chem. Soc., 2014, 136, 13094–13097 CrossRef CAS PubMed.
- H. Y. Zou, P. F. Gao, M. X. Gao and C. Z. Huang, Analyst, 2015, 140, 4121–4129 RSC.
- G. Liu, G. Zhao, W. Zhou, Y. Liu, H. Pang, H. Zhang, D. Hao, X. Meng, P. Li, T. Kako and J. Ye, Adv. Funct. Mater., 2016, 26, 6822–6829 CrossRef CAS.
- G. Liu, J. Pan, L. Yin, J. T. S. Irvine, F. Li, J. Tan, P. Wormald and H.-M. Cheng, Adv. Funct. Mater., 2012, 22, 3233–3238 CrossRef CAS.
- N. Waiskopf, Y. Ben-Shahar, M. Galchenko, I. Carmel, G. Moshitzky, H. Soreq and U. Banin, Nano Lett., 2016, 16, 4266–4273 CrossRef CAS PubMed.
- M. Quick, A. L. Dobryakov, M. Gerecke, C. Richter, F. Berndt, I. N. Ioffe, A. A. Granovsky, R. Mahrwald, N. P. Ernsting and S. A. Kovalenko, J. Phys. Chem. B, 2014, 118, 8756–8771 CrossRef CAS PubMed.
- M. Irie, J. Am. Chem. Soc., 1983, 105, 2078–2079 CrossRef CAS.
- C. Wang, Q. Zhang, X. Wang, H. Chang, S. Zhang, Y. Tang, J. Xu, R. Qi and Y. Cheng, Angew. Chem., Int. Ed., 2017, 56, 6767–6772 CrossRef CAS PubMed.
- C. Li, Y. Wang, C. Li, S. Xu, X. Hou and P. Wu, ACS Appl. Mater. Interfaces, 2019, 11, 20770–20777 CrossRef CAS PubMed.
- C. Li, Q. Xu, S. Xu, X. Zhang, X. Hou and P. Wu, RSC Adv., 2017, 7, 16204–16209 RSC.
- Y. Zheng, Z. Yu, H. Ou, A. M. Asiri, Y. Chen and X. Wang, Adv. Funct. Mater., 2018, 28, 1705407 CrossRef.
- M. Sun, L. Xu, A. Qu, P. Zhao, T. Hao, W. Ma, C. Hao, X. Wen, F. M. Colombari, A. F. de Moura, N. A. Kotov, C. Xu and H. Kuang, Nat. Chem., 2018, 10, 821–830 CrossRef CAS PubMed.
- J. Zhang, S. Wu, L. Ma, P. Wu and J. Liu, Nano Res., 2020, 13, 455–460 CrossRef CAS.
- J. Zhang, X. Lu, D. Tang, S. Wu, X. Hou, J. Liu and P. Wu, ACS Appl. Mater. Interfaces, 2018, 10, 40808–40814 CrossRef CAS PubMed.
- M. J. Meziani, X. Dong, L. Zhu, L. P. Jones, G. E. LeCroy, F. Yang, S. Wang, P. Wang, Y. Zhao, L. Yang, R. A. Tripp and Y.-P. Sun, ACS Appl. Mater. Interfaces, 2016, 8, 10761–10766 CrossRef CAS PubMed.
- X. Xie, C. Mao, X. Liu, Y. Zhang, Z. Cui, X. Yang, K. W. K. Yeung, H. Pan, P. K. Chu and S. Wu, ACS Appl. Mater. Interfaces, 2017, 9, 26417–26428 CrossRef CAS PubMed.
- C. M. Courtney, S. M. Goodman, J. A. McDaniel, N. E. Madinger, A. Chatterjee and P. Nagpal, Nat. Mater., 2016, 15, 529–534 CrossRef CAS PubMed.
- J. Zhang and J. Liu, Nanoscale, 2020, 12, 2914–2923 RSC.
- S. Wu, J. Zhang and P. Wu, Anal. Methods, 2019, 11, 5081–5088 RSC.
- W. Bi, X. Li, L. Zhang, T. Jin, L. Zhang, Q. Zhang, Y. Luo, C. Wu and Y. Xie, Nat. Commun., 2015, 6, 8647 CrossRef CAS PubMed.
- J. Li, X. Liu, L. Tan, Y. Liang, Z. Cui, X. Yang, S. Zhu, Z. Li, Y. Zheng, K. W. K. Yeung, X. Wang and S. Wu, Small Methods, 2019, 3, 1900048 CrossRef.
- P. Zhang, T. Wang, X. Chang and J. Gong, Acc. Chem. Res., 2016, 49, 911–921 CrossRef CAS PubMed.
- F. D'Souza and O. Ito, Chem. Soc. Rev., 2012, 41, 86–96 RSC.
- J. Zhang, S. Wu, X. Lu, P. Wu and J. Liu, Nano Lett., 2019, 19, 3214–3220 CrossRef CAS PubMed.
- C. Wang, Y. Shi, Y.-Y. Dan, X.-G. Nie, J. Li and X.-H. Xia, Chem. – Eur. J., 2017, 23, 6717–6723 CrossRef CAS PubMed.
- F. Su, S. C. Mathew, G. Lipner, X. Fu, M. Antonietti, S. Blechert and X. Wang, J. Am. Chem. Soc., 2010, 132, 16299–16301 CrossRef CAS PubMed.
- R. Wang, B. Li, Y. Xiao, X. Tao, X. Su and X. Dong, J. Catal., 2018, 364, 154–165 CrossRef CAS.
- X. Sun, X. Luo, X. Zhang, J. Xie, S. Jin, H. Wang, X. Zheng, X. Wu and Y. Xie, J. Am. Chem. Soc., 2019, 141, 3797–3801 CrossRef CAS PubMed.
- H. M. D. Bandara and S. C. Burdette, Chem. Soc. Rev., 2012, 41, 1809–1825 RSC.
- J. Tamogami, T. Kikukawa, T. Nara, K. Shimono, M. Demura and N. Kamo, Biochemistry, 2012, 51, 9290–9301 CrossRef CAS PubMed.
- H. K. Moon, S. H. Lee and H. C. Choi, ACS Nano, 2009, 3, 3707–3713 CrossRef CAS PubMed.
- F. Wang, E. Ju, Y. Guan, J. Ren and X. Qu, Small, 2017, 13, 1603051 CrossRef PubMed.
- F. Wang, Y. Zhang, Z. Du, J. Ren and X. Qu, Nat. Commun., 2018, 9, 1209 CrossRef PubMed.
- S. Neri, S. G. Martin, C. Pezzato and L. J. Prins, J. Am. Chem. Soc., 2017, 139, 1794–1797 CrossRef CAS PubMed.
- S. Laimgruber, T. Schmierer, P. Gilch, K. Kiewisch and J. Neugebauer, Phys. Chem. Chem. Phys., 2008, 10, 3872–3882 RSC.
- X. Wang, A. Gong, W. Luo, H. Wang, C. Lin, X. Y. Liu and Y. Lin, Chem. Commun., 2018, 54, 8641–8644 RSC.
- J. Niu, Y. Sun, F. Wang, C. Zhao, J. Ren and X. Qu, Chem. Mater., 2018, 30, 7027–7033 CrossRef CAS.
- L. Fan, X. Xu, C. Zhu, J. Han, L. Gao, J. Xi and R. Guo, ACS Appl. Mater. Interfaces, 2018, 10, 4502–4511 CrossRef CAS PubMed.
- F. Yesilkoy, R. A. Terborg, J. Pello, A. A. Belushkin, Y. Jahani, V. Pruneri and H. Altug, Light: Sci. Appl., 2018, 7, 17152 CrossRef PubMed.
- V. K. Johns, P. K. Patel, S. Hassett, P. Calvo-Marzal, Y. Qin and K. Y. Chumbimuni-Torres, Anal. Chem., 2014, 86, 6184–6187 CrossRef CAS PubMed.
- J. Kim, S. Lee, K. Jung, W. C. Oh, N. Kim, S. Son, Y. Jo, H.-B. Kwon and W. D. Heo, Nat. Commun., 2019, 10, 211 CrossRef PubMed.
- Y. Jin, Adv. Mater., 2012, 24, 5153–5165 CrossRef CAS PubMed.
- Y. Shiraishi, K. Adachi, M. Itoh and T. Hirai, Org. Lett., 2009, 11, 3482–3485 CrossRef CAS PubMed.
- F. Nourmohammadian, T. Wu and N. R. Branda, Chem. Commun., 2011, 47, 10954–10956 RSC.
- N. Shao, J. Y. Jin, S. M. Cheung, R. H. Yang, W. H. Chan and T. Mo, Angew. Chem., Int. Ed., 2006, 45, 4944–4948 CrossRef CAS PubMed.
- V. Valderrey, A. Bonasera, S. Fredrich and S. Hecht, Angew. Chem., Int. Ed., 2017, 56, 1914–1918 CrossRef CAS PubMed.
- W.-W. Zhao, J.-J. Xu and H.-Y. Chen, Chem. Soc. Rev., 2015, 44, 729–741 RSC.
- C. Feng, D. Chan, J. Joseph, M. Muuronen, W. H. Coldren, N. Dai, I. R. Correa Jr., F. Furche, C. M. Hadad and R. C. Spitale, Nat. Chem. Biol., 2018, 14, 276–283 CrossRef CAS PubMed.
- G.-L. Wang, X.-F. Xu, L. Qiu, Y.-M. Dong, Z.-J. Li and C. Zhang, ACS Appl. Mater. Interfaces, 2014, 6, 6434–6442 CrossRef CAS PubMed.
- G.-L. Wang, L.-Y. Jin, Y.-M. Dong, X.-M. Wu and Z.-J. Li, Biosens. Bioelectron., 2015, 64, 523–529 CrossRef CAS PubMed.
- D. Wu, N. Hu, J. Liu, G. Fan, X. Li, J. Sun, C. Dai, Y. Suo, G. Li and Y. Wu, Talanta, 2018, 190, 103–109 CrossRef CAS PubMed.
- L.-Y. Jin, Y.-M. Dong, X.-M. Wu, G.-X. Cao and G.-L. Wang, Anal. Chem., 2015, 87, 10429–10436 CrossRef CAS PubMed.
- G.-L. Wang, X.-Q. Li, G.-X. Cao, F. Yuan, Y. Dong and Z. Li, Chem. Commun., 2017, 53, 11165–11168 RSC.
- G.-L. Wang, X. Xu, X. Wu, G. Cao, Y. Dong and Z. Li, J. Phys. Chem. C, 2014, 118, 28109–28117 CrossRef CAS.
- Y. Guo, X. Li, Y. Dong and G.-L. Wang, ACS Sustainable Chem. Eng., 2019, 7, 7572–7579 CrossRef CAS.
- P. Zhang, D. Sun, A. Cho, S. Weon, S. Lee, J. Lee, J. W. Han, D.-P. Kim and W. Choi, Nat. Commun., 2019, 10, 940 CrossRef PubMed.
- Y. Liu, M. Zhou, W. Cao, X. Wang, Q. Wang, S. Li and H. Wei, Anal. Chem., 2019, 91, 8170–8175 CrossRef CAS PubMed.
- H. S. White, W. G. Becker and A. J. Bard, J. Phys. Chem., 1984, 88, 1840–1846 CrossRef CAS.
|
This journal is © The Royal Society of Chemistry 2020 |
Click here to see how this site uses Cookies. View our privacy policy here.