Bifunctional up-converting lanthanide nanoparticles for selective in vitro imaging and inhibition of cyclin D as anti-cancer agents†
Received
26th July 2013
, Accepted 21st October 2013
First published on 18th November 2013
Abstract
Inhibition of the CDK4/cyclin D complex through the substrate recruitment site on the cyclin positive regulatory subunit is recognised as being a promising anti-cancer target. Specific peptide sequences can be used to selectively disrupt this target, but the development of peptides as anti-tumor agents in vitro/in vivo presents several obstacles. Poor cell internalization, low sensitivity towards enzymatic degradation in vivo, and ineffectiveness in monitoring via indirect screening are all issues which must be overcome. Herein, we describe the surface functionalization of lanthanide nanoparticles with cyclin D-specific peptides to prepare novel nanomaterials (UCNPs–P1) which can target the CDK4/cyclin D complex. The nanomaterials prepared (UCNPs–P1) are cell permeable and they display parallel emission spectra in vitro and in an aqueous biological environment. They can also be used in low dose concentrations under harmless NIR excitation and emission via upconversion. Uniquely, in addition to acting as a bioimaging probe, UCNPs–P1 also exhibits promising cytotoxicity towards cancer cells. In light of the aforementioned properties, the prepared functionalized nanomaterials (UCNPs–P1) offer the first real dual acting system for cyclin D imaging and simultaneous inhibition of cancer cell division.
1. Introduction
Being one of the key cell cycle regulatory proteins which are subjected to ectopic overexpression in tumor cells, cyclin D1/CDK4 has long been the focus of oncological research for cancer targeting.1–4 Its consensus sequence has been found, in many cell cycles and tumor suppressor proteins, to be the cyclin-binding motif. To date, the selective inhibition and imaging of cyclin D1 can and must be carried out separately and alternatively with the use of specific peptides, small molecules or complexes.5–7
In the development of cancer diagnostic and therapeutic agents, the rise of peptide aptamers and small interfering RNA (siRNA) molecules in research cannot be overstated. It is true that siRNAs can selectively block the cyclin subunit, but introduction of an excess amount may lead to ill-defined off-targeting and nonspecific events due to the activation of innate immune responses.8–11 In contrast, peptides have been considered as potential drugs due to their target specificity, interfering capacity and low dosage requirement in use. It has been found that the transducible forms of cyclin groove inhibitor (CGI) peptides can suppress cyclin D activity, triggering cell cycle arrest and selective apoptosis in tumor cells in vitro.12–14 However, using peptidic CGIs is inevitably fraught with some difficulties, encompassing their physical and chemical instabilities, limited cell internalization, and low effectiveness to be monitored/evaluated via indirect screening.15–17 In particular, real-time evaluation of the anti-cancer potency (such as toxicity, MTT, tumor inhibition), visualization of the therapy process in vitro (e.g. protein therapeutics/drug delivery) and in vitro/in vivo imaging for cyclin specific peptides of no luminescence properties (by labeling with a primary antibody followed by amplification with an organic dye conjugate) are still very arduous or undesirable, if not impossible. All of these factors hamper the therapeutic applications of such peptide-based medicines (Scheme 1).
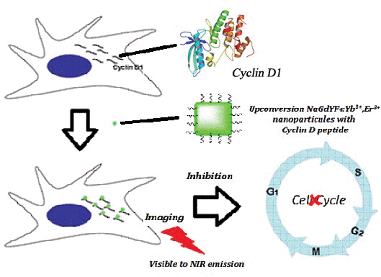 |
| Scheme 1 Schematic representation of the final goal of this project: dual functions (imaging and inhibition) of cyclin D1-targeting peptide–lanthanide nanoparticles in the cell cycle. | |
In effect, using a many-sided delivery vehicle is a panacea for all of the limitations and challenges mentioned above. One such solution is our lanthanide nanomaterials. Upon conjugation with our biocompatible lanthanide complexes, target-specific peptides can then be protected from enzymatic degradation and biological conditions;18–20 the overall water solubility can also be improved. Most importantly, it is the combination of specific imaging and selective inhibition of cyclin D1 that can now be accomplished synchronously.21–23 To the best of our knowledge, there has so far been limited development of lanthanide probes targeting specific peptides or proteins sensitized with near-infrared excitation.24–29 The only study in the literature characterizing a specific cyclin A/mRNA core peptide sequence tagged to a terbium complex entails absorption and emission in the ultra-violet region;24,28 Pazos et al. has demonstrated the intermolecular sensitization of lanthanide ions as a useful strategy for the design of cyclin A/mRNA biosensors. Despite this, the major drawback of their methodologies lies in the excitation of Trp217/mRNA as the antenna in the UV region, which is not suitable for in vitro imaging. Recently, our co-workers have developed two-photon (NIR excitation) available lanthanide bio-probes for visualizing the cyclin A in vitro.28 However, the two-photon induced emission quantum efficiencies of these complexes are still very low and no inhibition effect on the mitosis of the cancer cells can be observed. Lanthanide-based up-conversion nanomaterials are thus more promising with a decent quantum efficiency compared to the available two-photon complexes. In this regard, there are a plethora of lanthanide materials capable of energy up-conversion for in vitro imaging in the literature, but their localization profiles and in-depth studies of their potential bio-applications, such as dual-functional MRI, in vitro imaging and drug delivery, are still lacking.
Herein, we have synthesized several up-converted lanthanide nanoparticles, NaGdF4:Yb3+, Er3+ (UCNPs) with which cyclin D-specific inhibitory peptides, SAKRRLFG–NH2 (P1)21, have been coated (UCNPs–P1). In addition to protecting fragile peptides from biological decomposition, these modified nanomaterials (UCNPs–P1) can not only trigger a high selectivity of cell cycle inhibition as CGIs by the delivery of cyclin D-specific inhibition peptides and interruption of mitosis in cancer cells, but they can also offer simultaneous real-time imaging via efficient NIR excitation, thereby meeting our needs. The binding selectivities and affinities between cyclin D and our nanomaterials have been validated by multi-task experiments such as spectroscopic studies and competitive assays via western blotting. It is the versatility and selectivity of our nanomaterials that paves the way for the development of new-generation lanthanide materials as dual bio-probes, for imaging and inhibition.
2. Results and discussion
2.1 Synthesis and surface characterisation of the initial nanoparticles (UCNPs) and peptide-coated nanoparticles (UCNPs–P1) using FTIR spectroscopy
The overall synthetic approach used to prepare the peptide-coated nanoparticles (UCNPs–P1) is detailed in Scheme 2. The initial surface modification of the lanthanide nanoparticles NaGdF4:Yb3+, Er3+ (UCNPs) could be evidenced by FTIR. The corresponding IR transmission spectrum of the amine-functionalized UCNPs is shown in Fig 1(a). The board absorption band at 3427 cm−1 is due to the O–H/N–H stretching vibrations. The absorption peak at 2922 and 2850 cm−1 corresponds to the stretching vibration of the C–H bond. The IR absorption peaks at 1639 and 1437 cm−1 are attributed to the N–H bending of the amino group (NH2) and the C–N bond stretching vibration. Therefore, the IR transmission spectrum obtained supports the capping of the UCNPs with poly(ethylenimine) (PEI).30,31 The next step in the process involved the attachment of a reactive alkene handle (step b, Scheme 2). Again, this modification step was verified by the IR spectrum in Fig 1(b). The overall spectrum is similar to Fig 1(a). However, the additional absorption peaks at 1645 cm−1 correspond to the stretching vibration of the C
O bond.32 In the final step (step c, Scheme 2) of the synthesis, the N-terminal cysteine of the cyclin D-targeting peptide (P1) was reacted with the reactive alkene on the nanoparticles to give UCNPs–P1. Fig 1(c) shows the IR spectrum obtained after coating the UCNPs–C
C with P1. The small absorption peaks at around 3159 to 3537 cm−1 represent the N–H bond stretching, while the doublet absorption peak at 658 and 577 cm−1 represents the stretching vibration of the C–S bond.33
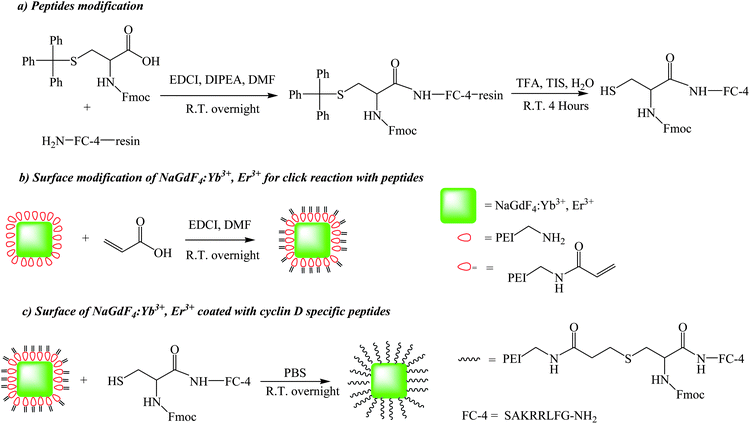 |
| Scheme 2 The synthetic schemes of the dual functional up-conversion nanoparticles (UCNPs–P1) from the NaGdF4:Yb3+, Er3+ nanoparticles (UCNPs) coated with cyclin D (P1–SAKRRLFG–NH2) specific peptides. | |
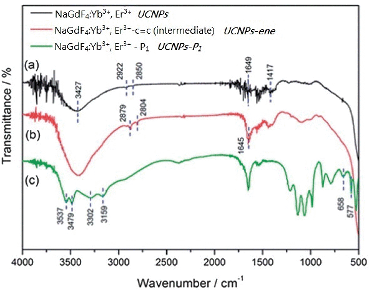 |
| Fig. 1 FTIR transmission spectrum of (a) amine-functionalized NaGdF4:Yb3+, Er3+ (UCNPs), (b) click reaction-modified NaGdF4:Yb3+, Er3+ (UCNPs-ene) and (c) cyclin D-specific peptides-coated NaGdF4:Yb3+, Er3+ (UCNPs–P1). | |
2.2 Phase and structure of the UCNPs and UCNPs–P1 nanomaterials
The shape and morphology of the initial nanoparticles (UCNPs) and the peptide-coated nanoparticles (UCNPs–P1) were investigated using TEM. In Fig 2(a), a square/cubic shape can be seen for the UCNPs. This corresponds to the cubic phase of NaGdF4, and the TEM image is similar to that previously reported for the synthesis of cubic phase NaGdF4 nanoparticles.34Fig 2(b) shows the high resolution TEM (HRTEM) image obtained from the UCNPs–P1 material. In this image, a thin layer that was not present in Fig 2(a) can be observed (indicated by the red arrow). This additional layer is due to the presence of the peptide P1 on the surface of the nanoparticles. The phase composition of the initial nanoparticles (UCNPs) and the peptide-coated nanoparticles (UCNPs–P1) was determined by powder X-ray diffraction (XRD). The initial nanoparticles (UCNPs) (Fig 3) were indexed to the standard cubic phase NaGdF4 (JSPDF#27-0697). The diffraction pattern agrees well with the standard pattern, thus the matching result indicates that the NaGdF4:Yb3+, Er3+ nanoparticles (UCNPs) are present in a pure cubic phase with no other impure phases. The XRD pattern of the peptide-coated nanoparticles (UCNPs–P1) shows no changes in the 2-theta angles (compared to the uncoated nanoparticles, UCNPs) and this implies that the coating of the peptide does not induce changes in crystal structure of the UCNPs. The aforementioned observation is also consistent with the non-crystalline nature of the peptide.
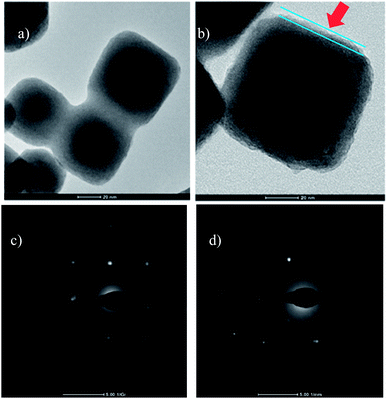 |
| Fig. 2 (a) TEM image of the initial nanoparticles (UCNPs). (b) HRTEM image of a single nanoparticle coated with the cyclin D-specific peptide (UCNPs–P1). High-magnification TEM images of the (c) UCNPs and (d) UCNPs–P1. | |
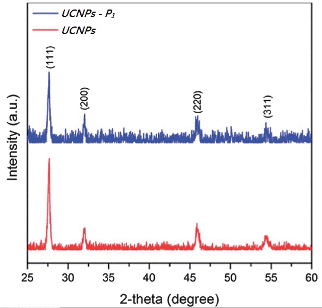 |
| Fig. 3 X-Ray diffraction patterns of the initial nanoparticles (UCNPs) and the peptide-coated nanoparticles (UCNPs–P1) indexed with a standard cubic phase NaGdF4 (JSPDF#27-0697). | |
Dynamic light scattering (DLS) allows one to investigate the diffusion properties of particles in solution, from which information on the apparent hydrodynamic diameter of the particles can be obtained. We carried out DLS measurements of both UCNPs and UCNPs–P1 in distilled water. The hydrodynamic diameter of UCNPs–P1 is shifted by around 7 nm compared with the UCNPs. Moreover, the DLS polydispersity index (PDI) and peak width at half height of the UCNPs are 1.855 and 154 nm respectively; the corresponding values for the UCNPs–P1 are −0.417 and 941.6 nm, respectively (Table S1†). This slight increase in hydrodynamic diameter could be ascribed mainly to the dwelling effect of the outer peptide coatings on the UCNPs. UCNPs have a hydrodynamic size distribution profile similar to the UCNPs–P1.
2.3 Up-conversion luminescence
With common organic fluorophores, emissive lanthanide ions transcend in terms of long emission lifetimes (effective elimination of biological autofluorescence in time-resolved spectroscopy) and characteristic hypersensitive emissions (provision of real-time information about the effect on the coordination environment by surrounding entities). In practice, cell imaging within the key biological window can even be fulfilled with the use of biocompatible luminescent up-conversion nanoparticles which offer visible to NIR emission by NIR excitation, so no autofluorescence would occur. The upconversion photophysical properties of the UCNPs and UCNPs–P1 were examined in the dispersed solution under near infrared excitation at 980 nm. The UCNPs and peptide-coated nanomaterials, UCNPs–P1, show similar up-conversion emission spectra under near-infrared excitation by using a continuous-wave (CW) 980 nm laser diode at 340 mW. Three upconversion emission peaks at 521, 541 and 650nm arise from the 2H11/2 to 4I15/2, 4S3/2 to 4I15/2, and 4F9/2 to 4I15/2 transitions, respectively. These three peaks at 521, 541 and 650 nm are ascribed to a two-photon upconversion process (Fig 4), which are the most common upconversion emissions present in nanoparticles doped with Yb3+ and Er3+ ions. When the same amount of the nanomaterial was put in the dispersed solution alone, the emission intensity was different, illustrating that the peptide linkage reduces the luminescence properties of the UCNPs.
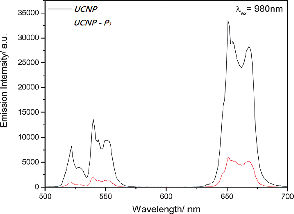 |
| Fig. 4 The up-conversion luminescence spectra of (a) NaGdF4:Yb3+, Er3+ (UCNPs) and (b) NaGdF4:Yb3+, Er3+–SAKRRLFG–NH2 (UCNPs–P1) (λex = 980 nm). | |
2.4 Analysis of the cyclin D binding affinity with NaGdF4:Yb3+, Er3+ (UCNPs) and peptide-coated NaGdF4:Yb3+, Er3+ (UCNPs–P1)
To confirm the specific binding of our nanoparticles (UCNPs–P1) towards the cellular cyclin D, a pull down assay followed by western blotting was carried out with the bare nanoparticles (UCNPs, as a control) and cyclin D-specific UCNPs–P1 (Fig 5). In general, HeLa cells were suspended with lysis buffer to release the proteins, and were then incubated with UCNPs or UCNPs–P1. Cellular cyclin D would be enriched by the nanoparticle coated with cyclin D peptides (UCNPs–P1), but not the bare UCNPs. Then non-specifically bound and free proteins were removed by washing the nanoparticles three times with lysis buffer. The black mark can only be observed by UCNPs–P1 with cyclin D (the white blanket in Fig. 5). On the contrary, the nanoparticle-enriched proteins were separated by SDS-PAGE (Sodium Dodecyl Sulfate-Polyacrylamide Gel Electrophoresis) and probed using antibodies. The results show that UCNPs–P1 did pull down the cyclin D1 protein from the cell lysis, while the bare UCNPs were significantly less potent in enriching cyclin D1. The unrelated DNA replication inhibitory protein Geminin was examined in parallel, and both UCNPs and UCNPs–P1 could not enrich the Geminin. The above experiment confirms that our UCNPs–P1 nanoparticles have a strong binding affinity to cyclin D.
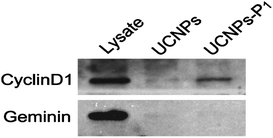 |
| Fig. 5 The pull down assay proved the binding of cyclin D1 with UCNPs–P1. HeLa cell lysate was incubated with 100 μg UCNPs or UCNPs–P1 for two hours, with the unbound proteins subsequently washed with lysis buffer. The nanoparticle-bound cyclin D1 proteins were examined using anti-cyclin D1 antibodies via western blotting. The lysate which contains the whole cellular proteins (lysate lane) was loaded as the positive control for the existence of the cyclin D1 protein, while the unrelated protein Geminin was blotted in parallel as unbound proteins (negative control). | |
2.5
In vitro imaging of cyclin D
The in vitro uptake rates of bare and peptide-coated NaGdF4:Yb3+, Er3+ nanoparticles are different, and were evaluated by ICP-MS in HeLa cells. (UCNPs −6.1925 ppt per cells and UCNPs–P1 −5.7970 ppb per cells). Given that our nanomaterials demonstrated interesting photophysical properties within the biological window, high selectivity to cyclin D, and can be up-taken easily by cancer cells, the further development of UCNPs–P1 to be in vitro imaging cyclin D probes is feasible. In vitro experiments had been conducted in cervical carcinoma HeLa cells with two-photon microscopes (Fig 6). UCNPs–P1 manifests detectable emission within the biological window (500 nm to 700 nm) via the near infrared excitation at 980 nm.
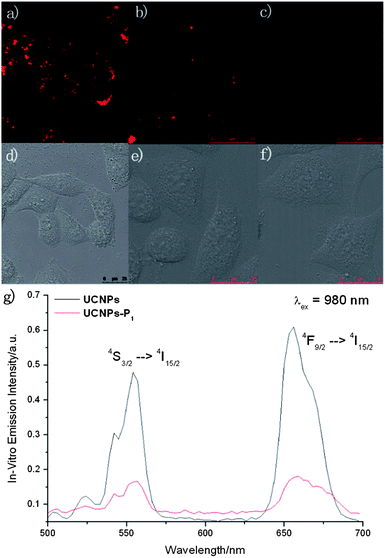 |
| Fig. 6
In vitro cell imaging of (a) NaGdF4:Yb3+, Er3+ (UCNPs), (b) NaGdF4:Yb3+, Er3+–SAKRRLFG–NH2 (UCNPs–P1) and (c) negative control, the HeLa cells were treated with siRNA before being dosed with UCNPs–P1; (d)–(f) bright field images corresponding to (a)–(c) respectively. (g) Upconversion in vitro emission spectra of the UCNPs (black) and UCNPs–P1 (red) in (a) and (b) (λex = 980 nm). | |
Under the NIR excitation, the impressive green (4S3/2 → 4I15/2) and red (Fig. 6, 4F9/2 → 4I15/2) f–f emission can be observed in the HeLa cells due to UCNPs–P1, after 3 hours of incubation. The doubts about the selectivity of these nanomaterials towards cyclin D have been overcome with two control experiments in Fig. 6via a two-photon microscope (λex = 980 nm). The lambda scan inside the two-photon microscope can be used to monitor the in vitro emission spectrum (resolution = 6 nm) in Fig. 6(f). As in the upconversion emission spectra of Fig. 4, the upconversion emission intensity of UCNPs–P1 is weaker than that of the UCNPs, which is the same as the in vitro emission spectrum inside the HeLa cells. Two erbium transitions (4S3/2 → 4I15/2 and 4F9/2 → 4I15/2) are visible in Fig. 6(g) with the excitation at 980 nm. The negative control had been carried out with imaging experiments which worked on the cells with the treatment of siRNA (to inhibit cyclin D generation, Fig. 6(c)). The upconversion emission diminished, as shown in the in vitro emission spectrum.
2.6 Inhibition of cancer Cell cycle and cell viability
Increases in the cyclin D and its partner kinase CDK level are well-observed in various kinds of cancers. As such, it is necessary to clarify the inhibitory activity of UCNPs–P1 in cancer cell lines prior to any further investigation and application. Two experiments (cell cycle flow cytometry and MTT assays) were carried out to study the capability of UCNPs–P1 to interrupt the cell division. First, flow cytometry analysis was conducted to examine the cell cycle phase distribution (G1, G2 and S phases) of the UCNPs–P1-treated HeLa cells. Treatment with UCNPs–P1 (50 or 200 μg mL−1), UCNPs (200 μg mL−1) and blank (no dosage of nanoparticles) followed, with the blank used as the experimental control. Then, cell phase distributions were calculated. As shown in Fig 7, UCNPs–P1-treated cells show an increase in the G1 phase (P < 0.01), indicating the potential for cyclin D inhibition. In contrast, the bare UCNPs show otherwise. Generally speaking, the cell phase distribution of usual cell division is ∼67%, ∼10% and ∼23% in the G1, G2 and S phases respectively. However, under the influence of UCNPs–P1, with 50/200 μg mol−1 doses in the HeLa cells, the G1 phase distribution rose significantly, while the G2 and S phases were under suppression simultaneously (Fig. 7 and S1†).
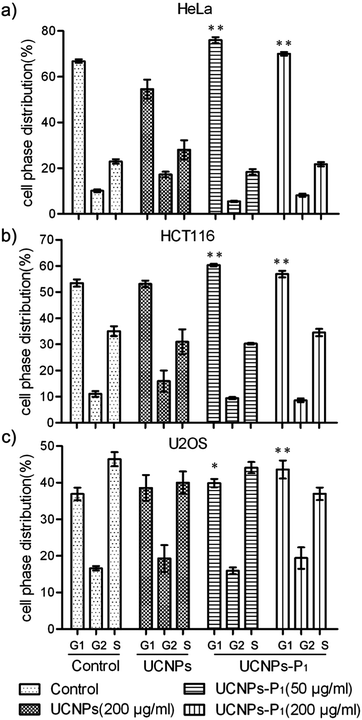 |
| Fig. 7 Flow cytometry analysis of (a) HeLa, (b) HCT116 and (c) U2OS cells treated with NaGdF4:Yb3+, Er3+ nanoparticles (UCNPs) and cyclin D-specific peptide-coated NaGdF4:Yb3+, Er3+ nanoparticles (UCNPs–P1) for 24 hours. The cell phase distribution was expressed as the percentage of G1, G2 and S. One-way analysis of variance was used in the data statistics (**P < 0.01). | |
In addition, cell viability was examined using MTT assays. Cancer cells HeLa, HCT116, U2OS and normal MRC-5 cells were treated with UCNPs or UCNPs–P1 for 24 hours. The nanoparticle-caused effects on cell viability were examined by the formation of formazan after adding MTT (3-(4,5-dimethylthiazol-2-yl)-2,5-diphenyltetrazolium bromide) and revealed by absorbance. Cell viability inhibition rates were also calculated. In Fig. 8, UCNPs–P1 obviously exerts an inhibition effect on cell viability, in comparison with the results from using UCNPs in all tested cell lines. In addition, UCNPs–P1 exerts a slight effect on the viability of normal MRC-5 cells. These results will contribute to the further application of UCNPs–P1.
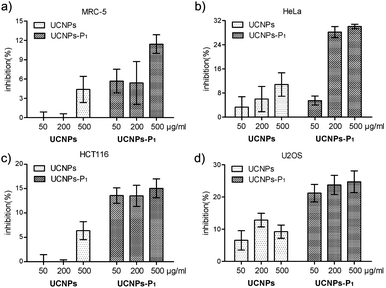 |
| Fig. 8 Cell viability inhibitory activity of cyclin D-specific peptide-coated NaGdF4:Yb3+, Er3+ nanoparticles (UCNPs–P1) as well as NaGdF4:Yb3+, Er3+ nanoparticles (UCNPs) on (a) human normal lung fibroblast cells, (b) human cervical cancer HeLa, (c) human colorectal cancer HCT116 and (d) osteosarcoma U2OS cells. | |
3. Experimental
3.1 Chemicals and materials
Ln(NO3)3·6H2O (Ln = Gd, Yb, Er) were purchased from Aldrich and dissolved in deionized water (DI-water) to form solutions with concentrations of 0.5 M and 0.1 M. Acrylic acid, Fmoc–Cys–S(Ph)3 and triisopropylsilane (TIS) were purchased from Aldrich. Ethylene glycol (EG, 99%), branched polyethylenimine (PEI, 25 kDa) and trifluoroacetic acid (TFA, 99%) were purchased from Sigma-Aldrich. NH4F (99.99%) and NaCl (99.99%) were obtained from Sinopharm Chemical Reagent Co., China. 1-(3-Dimethylaminopropyl)-3-ethylcarbodiimide hydrochloride (EDCI) and diisopropylethylamine (DIPEA, 99%) were purchased from Meryer. All of these chemicals were used as received, without further purification.
3.2 Synthesis of NaGdF4:Yb3+, Er3+ up-conversion nanoparticles (UCNPs)
Water-dispersed NaGdF4:Yb3+, Er3+ (UCNPs) were prepared by a one-step hydrothermal method. Typically, 1 mmol of NaCl and 1 mmol of Gd(NO3)3 (0.5 M), Yb(NO3)3 (0.5 M) and Er(NO3)3 (0.1 M) with a molar ratio of 78
:
20
:
2 were dissolved in 20 mL of ethylene glycol (EG) and stirred for 30 min. After capping with polyethyleneimine (PEI, branched 25 kDa), approximately 10 mL of EG containing 5.5 mmol of NH4F was then added to the above solution and stirred for another 30 min. The obtained mixture was then transferred into a 50 mL stainless Teflon-lined autoclave, which was sealed and kept at 190 °C for 24 h. After that, the autoclave was cooled to room temperature naturally, and the reaction mixture was separated through centrifugation. Ethanol and DI-water were used to wash the precipitation several times, which was then dried at 70 °C for 12 h to obtain the as-prepared samples. As a result, NaGdF4:Yb3+, Er3+ (UCNPs) with PEI as the surface modifier were prepared by a hydrothermal method. The ζ-potential for the UCNPs colloidal solution was measured to be around +23.6 mV, indicating the successful conjugation of positively charged PEI on the surface of the NPs.
3.3 Coating of cyclin D1-specific peptide on the UCNPs
50 mg bare NaGdF4 nanocrystals were added to 174 mg EDCI and 40 mg acrylic acid in DMF to react at room temperature overnight. The excess EDCI and acrylic acid was washed with DMF and methanol. The precipitate was then vacuum dried. 37.3 mg EDCI, 52.3 μL DIPEA and 40 mg Fmoc–Cys–S(Ph)3 were added to 50 mg cyclin D peptide in DMF and stirred at room temperature overnight. Excess EDCI, DIPEA and Fmoc–Cys–S(Ph)3 were washed away with DMF and methanol. The resin of modified cyclin D1 peptide was removed by reacting with TFA, TIS and DI–water in a ratio of 90
:
5
:
5 for 4 hours. Finally, the modified NaGdF4 nanocrystal and cyclin D1 peptide reacted with each other in PBS buffer at pH 7.2 at room temperature overnight. The product was washed with methanol and vacuum dried overnight.
3.4 Characterization
Powder X-ray diffraction (XRD) patterns of the as-prepared UCNPs were recorded using a Bruker AXS D8 Advance X-ray diffractometer at 40 kV and 40 mA with Cu-Kα radiation (λ = 1.5406 Å). The morphology of the as-prepared samples was characterized by using a Tecnai G2 20 S-TWIN Transmission Electron Microscope, operated at 200 kV. The Fourier transform infrared spectra were recorded by an Fourier Transform Infra-Red Spectrometer (Nicolet Avator 630). The ζ-potential measurements were performed on a Zetasizer 3000 HAS instrument. The up-conversion spectra were recorded using an FLS920P Edinburgh analytical instrument apparatus equipped with a 980 nm diode laser as the excitation source.
3.5 Cell culture
Human cervical carcinoma HeLa cells were purchased from the American Type Culture Collection (ATCC) (#CCL-185, ATCC, Manassas, VA, USA). The HeLa cells were grown in Dulbecco's Modified Eagle Medium (DMEM) supplemented with 10% fetal bovine serum (FBS), 1% penicillin and streptomycin at 37 °C and 5% CO2. To apply NaGdF4:Yb/Er for fluorescent imaging, HeLa cells were incubated in DMEM containing 100 mg mL−1 of cyclin D1-specific peptide-coated UCNPs at 37 °C for 24 hours under 5% CO2, and then washed with PBS sufficiently to remove excess NPs. In addition, human MRC-5 lung fibroblasts were grown in MEM medium, human colorectal cancer HCT116 cells were maintained in McCoy's 5A medium, while human osteosarcoma U2OS cells were maintained in RPMI 1640 medium, with both supplemented with 10% FBS.
3.6
In vitro bioimaging
To test the suitability of the obtained cyclin D1-specific peptide-coated UCNPs as bioprobes, bioimaging of HeLa cells incubated with NaGdF4:Yb/Er was performed on a commercial confocal laser scanning microscope, Leica TCS SP5, equipped with a Ti:Sapphire laser (Libra II, Coherent). The samples were excited by a 980 nm wavelength laser.
3.7 MTT cell viability assay
Cells treated with NPs for 24 hours were further incubated with MTT, 3-(4,5-dimethylthiazol-2-yl)-2,5-diphenyltetrazolium bromide (0.5 mg mL−1) for 4 hours, to produce formazan during cell metabolism. Then, formazan was thoroughly dissolved by dimethyl sulfoxide (DMSO), and the absorbances of the solutions were measured with a Bio-Rad iMark microplate reader (490 nm). Quadruplicates were performed. Data analysis and plotting were operated by the GraphPad Prism 5 software.
4. Conclusions
In conclusion, we have successfully functionalized lanthanide nanoparticles with a cyclin D-specific peptide to generate novel UCNPs–P1 nanomaterials. UCNPs–P1 display similar emission spectra in vitro and in aqueous biological environments, and they have the ability to cause cell cycle disruption via G1-phase arrest. Such an interruption correlates with a striking inhibiting effect and prominent toxicity towards cancer cells. Imaging can be achieved at low dose concentrations under harmless NIR excitation. The UCNPs–P1 nanomaterials overcome some of the major drawbacks, such as cell permeability, that are associated with developing “free” peptides as anti-cancer agents. The functionalized nanomaterial-prepared UCNPs–P1 represent the first true dual functioning system that enables both cyclin D imaging and cancer cell division inhibition. As such, they are promising systems for the development of new anti-cancer agents.
Acknowledgements
This work was funded by grants from The Hong Kong Research Grants Council (HKBU 203012), ESPRC, Hong Kong Baptist University (FRG 2/12-13/002), The Hong Kong Polytechnic University (GYL01 and GUA22) and Durham University.
Notes and references
- V. J. LiCata and A. J. Wowor, Methods Cell Biol., 2008, 84, 243 CrossRef CAS.
- M. Malumbres and M. Barbacid, Cancer Cell, 2006, 9, 2 CrossRef CAS PubMed.
- M. W. Landis, B. S. Pawlyk, T. Li, P. Sicinski and P. W. Hinds, Cancer Cell, 2006, 9, 13 CrossRef CAS PubMed.
- H. K. Reddy, R. V. Mettus, S. G. Rane, X. Graña, J. Litvin and E. P. Reddy, Cancer Res., 2005, 65, 10174 CrossRef CAS PubMed.
- C. Mclnnes, M. J. I. Andrews, D. I. Zheleva, D. P. Lane and P. M. Fisher, Curr. Med. Chem.: Anti-Cancer Agents, 2003, 3, 57 CrossRef.
- A. M. Senderowicz, Clin. Lung Cancer, 2003, 5, 158 CrossRef CAS PubMed.
- D. Cirillo, F. Pentimalli and A. Giordano, Curr. Med. Chem., 2011, 18, 2854 CrossRef CAS.
-
J. A. Birchler, RNA Interference: What is it?, in RNA Interference: Application to Drug Discovery and Challenges to Pharmaceutical Development, ed. P. H. Johnson, John Wiley & Sons, Inc., Hoboken, NJ, USA, 2011 Search PubMed.
- Y. K. Oh and T. G. Park, Adv. Drug Delivery Rev., 2009, 61, 850 CrossRef CAS PubMed.
- F. Takeshita and T. Ochiya, Cancer Sci., 2006, 97, 689 CrossRef CAS PubMed.
- A. Grünweller and R. K. Hartmann, Curr. Med. Chem., 2005, 12, 3143 CrossRef.
- Q. T. Nguyena, E. S. Olson, T. A. Aguilera, T. Jiang, M. Scadenge, L. G. Elliesf and R. Y. Tsien, Proc. Natl. Acad. Sci. U. S. A., 2010, 107, 4317 CrossRef PubMed.
- T. Shiraishi and P. E. Nielsen, Nat. Protoc., 2006, 1, 633 CrossRef CAS PubMed.
- S. E. Andaloussi, P. Guterstam and A. Langel, Nat. Protoc., 2007, 2, 2043 CrossRef PubMed.
- D. I. Zheleva, C. Mclnnes, A. L. Gavine, N. Z. Zhelev, P. M. Fisher and D. P. Lane, J. Pept. Res., 2002, 60, 257 CrossRef CAS.
- G. Kontopidis, M. J. I. Andrews, C. McInnes, A. Cowan, H. Powers, L. Innes, A. Plater, G. Griffiths, D. Paterson, D. I. Zheleva, D. P. Lane, S. Green, M. D. Walkinshaw and P. M. Fischer, Structure, 2003, 11, 1537 CrossRef CAS PubMed.
- M. J. Andrews, G. Kontopidis, C. McInnes, A. Plater, L. Innes, A. Cowan, P. Jewsbury and P. M. Fischer, ChemBioChem, 2006, 7, 1909 CrossRef CAS PubMed.
- S. J. Butler and D. Parker, Chem. Soc. Rev., 2013, 42, 1652 RSC.
- J. C. G. Bünzli and S. V. Eliseeva, Chem. Sci., 2013, 4, 1939 RSC.
- F. Piccinelli, A. Melchior, A. Speghini, M. Monari, M. Tolazzi and M. Bettinelli, Polyhedron, 2013, 57, 30 CrossRef CAS PubMed.
- M. E. Burkard, A. Santamaria and P. V. Jallepalli, ACS Chem. Biol., 2012, 7, 978 CrossRef CAS PubMed.
- L. Garuti, M. Roberti and G. Bottegoni, Curr. Med. Chem., 2012, 19, 3937 CrossRef CAS.
- M. Nihal, N. Stutz, T. Schmit, N. Ahmad and G. S. Wood, Cell Cycle, 2011, 10, 1303 CrossRef CAS.
- C. Penas, E. Pazos, J. L. Mascareñas, M. Eugenio and M. Vázquez, J. Am. Chem. Soc., 2013, 135, 3812 CrossRef CAS PubMed.
- M. S. Tremblay, Q. Zhu, A. A. Martí, J. Dyer, M. Halim, S. Jockusch, N. J. Turro and D. Sames, Org. Lett., 2006, 8, 2723 CrossRef CAS PubMed.
- T. Terai, K. Kikuchi, S. Y. Iwasawa, T. Kawabe, Y. Hirata, Y. Urano and T. Nagano, J. Am. Chem. Soc., 2006, 128, 6938 CrossRef CAS PubMed.
- C. S. Bonnet, M. Devocelle and T. Gunnlaugsson, Org. Biomol. Chem., 2012, 10, 126 CAS.
- E. Pazos, D. Torrecilla, M. V. López, L. Castedo, J. L. Mascareňas, A. Vidal and M. E. Vázquez, J. Am. Chem. Soc., 2008, 130, 9652 CrossRef CAS PubMed.
- H.-K. Kong, F.-L. Chadbourne, G.-L. Law, H. Li, H.-L. Tam, S.-L. Cobb, C. K. Lau, C.-S. Lee and K.-L. Wong, Chem. Commun., 2011, 47, 8052 RSC.
- H. T. Wong, M. K. Tsang, C. F. Chan, K. L. Wong, B. Fei and J. H. Hao, Nanoscale, 2013, 5, 3465 RSC.
- F. Wang, D. K. Chatterjee1, Z. Q. Li, Y. Zhang, X. P. Fan and M. Q. Wang, Nanotechnology, 2006, 17, 5786 CrossRef CAS.
-
E. Pretsch, P. Bühlmann and C. Affolter, Structure Determination of Organic Compounds, Springer, Berlin Heidelberg, 2000, p. 250 Search PubMed.
-
E. Pretsch, P. Bühlmann and C. Affolter, Structure Determination of Organic Compounds, Springer, Berlin Heidelberg, 2000, p. 268 Search PubMed.
- Y. Park, J. H. Kim, K. T. Lee, K. S. Jeon, H.-B. Na, J. H. Yu, H. M. Kim, N. Lee, S. H. Choi, S. Baik, H. Kim, S. P. Park, B.-J. Park, Y.-W. Kim, S. H. Lee, S.-Y. Yoon, I.-C. Song, W. K. Moon, Y. D. Suh and T. Hyeon, Adv. Mater., 2009, 21, 4467 CrossRef CAS.
Footnote |
† Electronic supplementary information (ESI) available. See DOI: 10.1039/c3tb21034k |
|
This journal is © The Royal Society of Chemistry 2014 |
Click here to see how this site uses Cookies. View our privacy policy here.