DOI:
10.1039/D2TC04759D
(Communication)
J. Mater. Chem. C, 2023,
11, 113-117
Boosting organic room-temperature phosphorescence performance through joint luminescence sensitization†
Received
9th November 2022
, Accepted 25th November 2022
First published on 29th November 2022
Abstract
Organic ultralong room-temperature phosphorescence (OURTP) has broken through the traditional understanding of luminance from molecular aggregated structures of π-conjugated materials. However, it is still challenging to develop highly efficient OURTP molecules owing to their spin-forbidden intersystem crossing. Herein, we present a rational design to boost OURTP performance through luminescence sensitization involving multi-step energy transfer. With the weak OURTP molecule 9-phenyl-9H-carbazole as the host and metal complexes/thermally activated delayed fluorescence guests as triplet exciton activators, the bicomponent materials exhibit extraordinary OURTP performance with a lifetime of up to 455 ms and quantum efficiency of up to 14.5% as well as color-resolved emission under ambient conditions. Detailed photophysical characterization verified that the guests with facile intersystem crossing could promote the population of triplet excitons via luminescence sensitization, and the triplet excitons were stabilized by H-aggregation of the host, thus boosting OURTP performance.
Introduction
Organic ultralong room-temperature phosphorescence (OURTP) has received extensive attention owing to its characteristics of long lifetime and full utilization of excited state energy1–3 and shows great potential in triplet-state-involved organic optoelectronic applications, such as data security,4,5 biological imaging,6,7 and afterglow organic light-emitting diodes.8,9 Recently, the luminescence mechanism of OURTP materials has been well investigated.10–12 As OURTP is a type of room-temperature phosphorescence (RTP) due to the radiative decay of triplet excited states (Tn), two important prerequisites should be fulfilled to achieve efficient OURTP from purely organic molecules.13,14 Firstly, it is crucial to transform the photoexcited singlet excitons of the first singlet state (S1) into triplet excitons effectively via facile S1 → Tn intersystem crossing (ISC), and many recent advances have focused on promoting ISC by enhancing spin–orbit coupling (SOC) with the use of heteroatoms,15,16 heavy atoms,17,18 or heteroatom-mediated resonance structures.13,19 Secondly, triplet excitons should be stabilized to suppress both the radiative and non-radiative relaxation rates for long-lived OURTP emission. However, owing to the spin-forbidden ISC nature of organic luminescent materials, efficient OURTP is still rarely observed, which greatly limits the expansion of this material system.
Multi-component host–guest architecture-based OURTP has been reported using co-crystallization and other methods,20–22 and almost all reported luminescence mechanisms still rely on the conventional wisdom that the host only plays passive roles in suppressing non-radiative decay or acts as an oxygen barrier to avoid triplet quenching. Recently, Adachi et al. innovatively exploited the strategy of photoinduced charge transfer to fully utilize the excited state energy in a host–guest doping system, realizing long persistent luminescence that lasted for more than one hour under a nitrogen atmosphere.1,23 Despite these attractive works,24–26 investigation on the detailed photophysics has rarely been reported, particularly involving multi-step energy transfer processes concerning the host species.
As is well known, luminescence sensitization can be achieved via intramolecular energy transfer upon excitation of the sensitizer instead of using direct excitation of the weak absorption bands of the host lattice.27,28 Considering that efficient ISC in traditional metal complexes or thermally activated delayed fluorescence (TADF) have been widely recognized,29,30 we hypothesize that luminescence sensitization may also be applicable to populate the T1 state in host–guest doped OURTP systems without the participation of the weak S1 → Tn ISC of the host itself. Additionally, the trace amounts of guest molecules would not disrupt the crystal structure, which is necessary to stabilize the triplet exciton, but rather boost the OURTP performance by acting as triplet exciton activators (Fig. 1). In contrast, the intermolecular heavy atom effect would not only promote the S1 → Tn ISC but also boost the T1 → S0 ISC, which is disadvantageous to maintaining long OURTP lifetime.
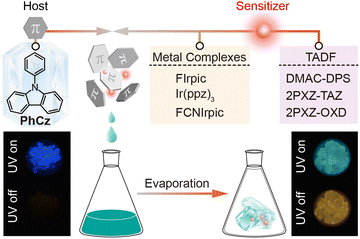 |
| Fig. 1 Schematic illustration of boosting organic room-temperature phosphorescence performance through luminescence sensitization. | |
Results and discussion
Proof of concept
9-Phenyl-9H-carbazole (PhCz) crystal is a weak but H-aggregation-dominated OURTP material with an ISC rate (kISC) of only 8.7 × 106 s−1 at room temperature (Fig. S1 and Table S1, S2, ESI†).17,31 The absorption and photoluminescence (PL) spectra of PhCz in dichloromethane (DCM) solution are similar to those of the carbazole moiety. Additionally, the energy gap (Eg) of PhCz was deduced to be ca. 3.54 eV while the triplet energy (ET) calculated from the highest-energy vibronic sub-band of the low temperature (77 K) phosphorescence spectrum was determined to be ca. 3.05 eV (Fig. S2, ESI†). It thus qualifies for application as a host for FIrpic (ET = 2.65 eV),32 Ir(ppz)3 (ET = 2.79 eV),33 FCNIrpic (ET = 2.71 eV),34 DMAC-DPS (Eg = 2.63 eV),35 2PXZ-TAZ (Eg = 2.59 eV), and 2PXZ-OXD (Eg = 2.42 eV) (Scheme S1, ESI†).36 When PhCz is in the crystal form, weak yellow OURTP appears with a lifetime of 240 ms (Fig. S3, ESI†). Beforehand, a simple contrast experiment was designed to demonstrate the important role of H-aggregation in stabilizing the triplet exciton to prolong the lifetime of OURTP.2,12 When PhCz was cooled naturally to room temperature after heating to its melting point (Tm) (∼97 °C) in a quartz tube, the OURTP disappeared as expected because heat gradually destroyed H-aggregation to yield the amorphous state in the heat cycle (Fig. S4, ESI†).
As a proof of concept, we first prepared FIrpic@PhCz from a mixture of PhCz and FIrpic (99
:
1 mass ratio) by removing the solvent under ambient conditions. X-Ray powder diffraction (XRPD) analysis reveals that the doped crystals showed no peak position shift compared to that of pure PhCz crystal and verifies that low-concentration dopant retained the H-aggregation structure of the host matrix, which is necessary to stabilize the triplet exciton (Fig. 2a).37 The PL spectra of the FIrpic@PhCz crystalline doped material exhibited a bimodal emission at about 481 and 499 nm belonging to the characteristic emission of FIrpic (Fig. 2b and Fig. S5, ESI†).32 The photosensitizer-dominated emission clearly shows facile energy transfer from the host to the luminescent guest. More interestingly, a yellowish OURTP that lasted ∼2 s was observed by the naked eye from FIrpic@PhCz after removing the 365 nm excitation source under ambient conditions (Fig. 2b). The OURTP spectrum of FIrpic@PhCz confirmed the yellowish afterglow as a result of the time-resolved emission bands in the range of 400–750 nm (Fig. 3a). We ascribed the emission peak at 502 nm to the RTP of the sensitizer, and its RTP nature was further confirmed by its emission lifetime of 36 ms (Fig. S6a, ESI†). During this, PhCz acted as a host matrix, significantly minimizing the quenching of the long-lifetime triplet excitons of the guest. The OURTP spectra of these samples between 500 and 750 nm were the OURTP emission of PhCz crystal, as its characteristic peaks were 548 and 596 nm (Fig. S3, ESI†). Additionally, the OURTP lifetime was found to significantly increase up to 365 ms (596 nm) compared to that of pure PhCz crystal, which only shows an OURTP lifetime of 240 ms (Table 1 and Fig. S6a, ESI†).17
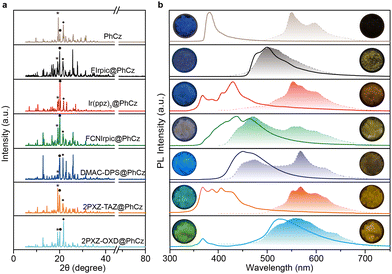 |
| Fig. 2 (a) XRPD patterns and (b) steady-state PL (solid lines)/OURTP spectra (dotted lines) excited by 295 nm under ambient conditions for FIrpic@PhCz, Ir(ppz)3@PhCz, FCNIrpic@PhCz, DMAC-DPS@PhCz, 2PXZ-TAZ@PhCz and 2PXZ-OXD@PhCz compared to that of pure PhCz crystal, and the insets in (b) show the photographs under (left) and after (right) 365 nm excitation. | |
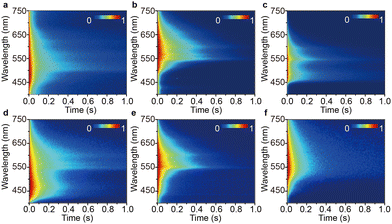 |
| Fig. 3 Transient PL decay images of (a) FIrpic@PhCz, (b) Ir(ppz)3@PhCz, (c) FCNIrpic@PhCz, (d) DMAC-DPS@PhCz, (e) 2PXZ-TAZ@PhCz and (f) 2PXZ-OXD@PhCz excited at 295 nm under ambient conditions. | |
Table 1 PL properties of pure PhCz, FIrpic@PhCz, Ir(ppz)3@PhCz, FCNIrpic@PhCz, DMAC-DPS@PhCz, 2PXZ-TAZ@PhCz and 2PXZ-OXD@PhCz excited by 295 nm under ambient conditions
Compound |
Steady-state PL |
Delayed PL |
λ (nm) |
η
f (%) |
λ
RTP/OURTP (nm) |
τ
(ms) |
η
OURTP
(%) |
η
OURTP
(%) |
To ensure consistency of results, all OURTP lifetimes were recorded after photoactivation and obtained using the time-dependent intensity profile method.
Quantum yields were obtained in air.
Quantum yields were obtained in nitrogen atmosphere.
|
PhCz
|
380 |
40.1 |
548/596 |
240/230 |
1.5 |
1.6 |
FIrpic@PhCz |
481/499 |
1.3 |
502/548/596 |
36/319/365 |
1.3 |
4.7 |
Ir(ppz)3@PhCz |
367/386/407/429 |
5.6 |
550/565/596 |
254/277/255 |
0.8 |
3.8 |
FCNIrpic@PhCz |
436/463 |
4.4 |
472/558/596 |
87/329/335 |
0.9 |
4.1 |
DMAC-DPS@PhCz |
450 |
17.9 |
470/569/615 |
18/365/455 |
10.1 |
11.0 |
2PXZ-TAZ@PhCz |
368/387/406 |
18.6 |
550/569/597 |
255/257/304 |
4.7 |
5.4 |
2PXZ-OXD@PhCz |
368/526 |
6.1 |
526/558/630 |
67/234/351 |
14.5 |
16.0 |
Large-scale applications
We then investigated the PL of the other metal complex-doped PhCz, namely Ir(ppz)3@PhCz and FCNIrpic@PhCz, under ambient conditions. All physically doped crystals showed yellowish OURTP, which was also confirmed by the time-resolved emission bands ranging from 400 to 750 nm (Fig. 2b). The emission band features longer emission lifetimes of 277 ms for Ir(ppz)3@PhCz (565 nm) and 329 ms for FCNIrpic@PhCz (550 nm) compared to pure PhCz crystal (Fig. 3b, c and Fig. S6b, c, ESI†). However, the efficiencies were only 0.8% for Ir(ppz)3@PhCz and 0.9% for FCNIrpic@PhCz, which were lower than that of pure PhCz crystal (1.8%) (Table 1). Evidently, metal complex-doped PhCz was more likely to be quenched by oxygen under ambient conditions.38
In a further set of experiments, TADF-doped PhCz samples (1
:
99 mass ratio) denoted as DMAC-DPS@PhCz, 2PXZ-TAZ@PhCz and 2PXZ-OXD@PhCz were prepared without affecting the original crystal structure of PhCz, which is reasonable as no position shift of the characteristic peaks was observed in the XRPD patterns (Fig. 2a). A set of emissions from 300 to 800 nm were observed, and the emission peaks at 365 and 387 nm belong to the residual fluorescence emission of the PhCz crystal, while the emission range from 400 to 550 nm is the fluorescence emission of the TADF guests, which can be confirmed by comparing their emission peaks in solution and thin film (Fig. 2b and Fig. S3, S5, ESI†).17,34 As expected, yellowish OURTP was observed after switching off the 365 nm excitation source under ambient conditions, which was also confirmed by the time-resolved emission bands in the range of 400–800 nm (Fig. 3d–f). Additionally, the phosphorescence peaks range from 400 to 500 nm and can be ascribed to the RTP of the sensitizer; their phosphorescent nature was further confirmed by their lifetime being shorter than 100 ms (Fig. S6d–f, ESI†). The OURTP spectra of these samples between 500 and 700 nm exhibited the afterglow emission of the PhCz crystal confirmed by its characteristic peak.17 Notably, the OURTP lifetime and efficiency were found to significantly increase to 455 ms and 14.5%, respectively, compared to those of the pure PhCz crystal (Table 1).
Photophysical processes
To uncover the mechanism of these phenomena, DMAC-DPS@PhCz was taken as a typical example. At room temperature, strong fluorescence of DMAC-DPS can be observed in the steady-state PL spectrum under 295 nm excitation. However, in the delayed spectrum, the strong phosphorescence peaks at 470 nm of DMAC-DPS as well as OURTP peaks at 569 and 615 nm of PhCz host appeared with emission lifetimes of 18, 365 and 455 ms, respectively (Fig. 4a and Fig. S6, ESI†), and the phosphorescence lifetime of the DMAC-DPS sensitizer in the crystal is much shorter than the OURTP lifetime of the PhCz host.14,17 Additionally, a high afterglow efficiency of 10.1% was obtained, which is much higher than that of the pure PhCz crystal (1.8%). Evidently, the facile energy transformation not only occurred from the PhCz host to the sensitizer guest but also occurred from the sensitizer guest to the PhCz host. More interestingly, in the time-dependent intensity profiles of the phosphorescence/OURTP emissions under ambient conditions, the luminescence intensity of the phosphorescence peak at 470 nm of DMAC-DPS decreases sharply in the first second, and so does the OURTP peak at 569 nm of the PhCz crystal, albeit much more slowly. However, the OURTP peak at 615 nm of the PhCz crystal increased slowly, and both the intensity and lifetime can be enhanced using continuous 365 nm UV light excitation, leading to strong OURTP emission that can be easily observed by the naked eye under ambient conditions. The OURTP can be facilely activated to a stable state using a 365 nm hand-held UV lamp for less than 2 s (Fig. 4b), which is not observed with the pure PhCz crystal. Further, we found that the intensity of OURTP can reach different levels at different photoactivation times. When the excitation time of a xenon arc lamp (Edinburgh FLS980) increased from 0.01 s to 5 s, the photoactivation intensity for OURTP increased correspondingly (Fig. 4c).
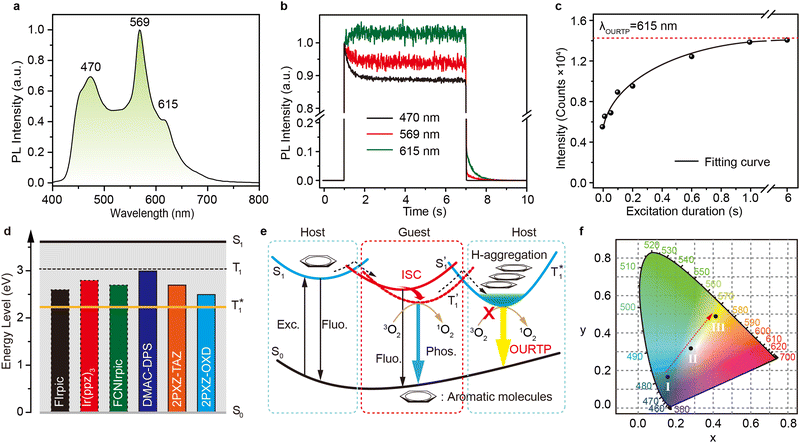 |
| Fig. 4 (a) OURTP spectrum with a delay time of 10 ms and (b) time-dependent intensity profiles of the phosphorescence (470 nm)/OURTP (569 and 615 nm) emissions of DMAC-DPS@PhCz excited by 295 nm under ambient conditions. (c) Influence of excitation duration on OURTP emission (615 nm) of DMAC-DPS@PhCz under ambient conditions; the sample was irradiated by 295 nm UV light for 0.01, 0.02, 0.06, 0.10, 0.60, 1.00 and 6.00 s. (d) Luminous energy of FIrpic, Ir(ppz)3, FCNIrpic, DMAC-DPS, 2PXZ-TAZ and 2PXZ-OXD and (e) proposed mechanism of luminescence sensitization-boosted OURTP in host–guest systems. (f) DMAC-DPS@PhCz shows color-tunable emission with Commission Internationale de l’Eclairage (CIE) 1931 coordinates of (0.16, 0.17), (0.28, 0.32) and (0.42, 0.50) with different delay time of (I) 0, (II) 10 and (III) 40 ms under 295 nm excitation, respectively. | |
To evaluate the effect on luminescence sensitization, we carefully analyzed the energy levels of PhCz and DMAC-DPS (Fig. 4d); the Eg and ET were deduced to be 3.54 eV and 3.05 eV, respectively, which are much higher than the Eg of DMAC-DPS (2.63 eV). When DMAC-DPS is doped into a host matrix with low concentration, the energy can easily transfer from the S1 of PhCz to the S1 of TADF sensitizers through efficient Förster resonance energy transfer.39 Besides, the large energy difference between PhCz and DMAC-DPS would prevent the energy return. Thus, benefiting from the efficient ISC process of the sensitizer, singlet excitons convert into triplet excitons easily. Meanwhile, the T1 (2.55 eV) of DMAC-DPS is higher than the stabilized
(2.25 eV) of PhCz; triplet excitons would be trapped by
to enhance OURTP, thus overcoming the disadvantage of the PhCz host with a weak S1 → Tn ISC process (Fig. 4d and e).12
We also evaluated the electrical properties of another sensitizer, as shown in Fig. 4d; the Eg/ET values were reported to be 2.59 eV for 2PXZ-TAZ, 2.42 eV for 2PXZ-OXD, 2.65 eV for FIrpic, 2.79 eV for Ir(ppz)3 and 2.71 eV for FCNIrpic, which is lower than the Eg/ET but higher than the
of PhCz. When metal complex/TADF-doped PhCz was excited by UV light, the energy easily transferred from the S1 of PhCz to the metal complex/TADF sensitizers through efficient Förster resonance energy transfer and exhibited sensitizer-dominated luminescence. Through the efficient ISC process of the metal complex/TADF sensitizer, singlet excitons are easily converted into triplet excitons. Thereafter, the triplet excitons were trapped by the
state of the PhCz crystal for enhanced OURTP. Notably, among the different emission lifetimes of the various excited energy levels, DMAC-DPS@PhCz exhibits color-tunable emission changes from blue to yellow over time, which is highly desirable for practical applications in optoelectronics, information encryption, anti-counterfeiting and sensing (Fig. 4f).
Effects of oxygen on OURTP
Judging from the detailed energy synergy of the luminescence sensitization system, triplet excitons in the sensitizers and PhCz crystal were more likely to be quenched by oxygen under ambient conditions (Fig. 4b and Table 1).38 To confirm this, dry nitrogen atmosphere-based time-dependent intensity profiles were recorded. As presented in Fig. S7 (ESI†), the phosphorescence intensity of the DMAC-DPS peak at 470 nm decreased more slowly in the nitrogen atmosphere as the triplet exciton is insensitive to nitrogen. However, the OURTP emission peak at 615 nm remains almost unchanged in the nitrogen atmosphere, which indicated that the H-aggregation-dominated PhCz crystal can not only protect triplet excitons from oxygen quenching but also effectively stabilize the triplet states, resulting in considerably prolonged excited triplet states under ambient conditions.
Conclusions
By integrating the weak OURTP host PhCz and sensitizer guest into a host–guest doped system, a series of efficient OURTP materials was successfully developed with improved OURTP performance. Detailed photophysical characterization revealed that luminescence sensitization involving multi-step energy transfer and sensitizers with effective ISC ability are two essential factors to obtain efficient host–guest doped OURTP materials, which may provide inspiration for developing OURTP materials based on normal conjugated hosts with a weak S1 → Tn ISC process, making this an extremely versatile system for developing a broad variety of highly efficient OURTP materials.
Conflicts of interest
There are no conflicts to declare.
Acknowledgements
This study was supported by the Natural Science Foundation of Jiangsu Province (BK20210935), Natural Science Foundation of the Jiangsu Higher Education Institutions of China (20KJB140012 and 22KJB150030), Starting Research Fund of Nanjing Vocational University of Industry Technology (YK20-14-03) and Open Research Fund of State Key Laboratory of Organic Electronics and Information Displays.
Notes and references
- K. Jinnai, R. Kabe, Z. Lin and C. Adachi, Nat. Mater., 2022, 21, 338–344 CrossRef CAS PubMed.
- Z. An, C. Zheng, Y. Tao, R. Chen, H. Shi, T. Chen, Z. Wang, H. Li, R. Deng and X. Liu, Nat. Mater., 2015, 14, 685–690 CrossRef CAS.
- C. Chen and B. Liu, Nat. Commun., 2019, 10, 1–15 CrossRef.
- H. Peng, G. Xie, Y. Cao, L. Zhang, X. Yan, X. Zhang, S. Miao, Y. Tao, H. Li and C. Zheng, Sci. Adv., 2022, 8, eabk2925 CrossRef CAS PubMed.
- J. Ren, Y. Tian, Y. Wang, J. Yang, M. Fang and Z. Li, J. Mater. Chem. C, 2022, 10, 13741–13746 RSC.
- J. Liu, Y. Sun, G. Wang, X. Chen, J. Li, X. Wang, Y. Zou, B. Wang and K. Zhang, Adv. Opt. Mater., 2022, 2201502 CrossRef CAS.
- J. Yang, X. Zhen, B. Wang, X. Gao, Z. Ren, J. Wang, Y. Xie, J. Li, Q. Peng and K. Pu, Nat. Commun., 2018, 9, 1–10 CrossRef.
- G. Xie, J. Wang, X. Xue, H. Li, N. Guo, H. Li, D. Wang, M. Li, W. Huang and R. Chen, Appl. Phys. Rev., 2022, 9, 031410 CAS.
- R. Kabe, N. Notsuka, K. Yoshida and C. Adachi, Adv. Mater., 2016, 28, 655–660 CrossRef CAS PubMed.
- Y. Xie, Y. Ge, Q. Peng, C. Li, Q. Li and Z. Li, Adv. Mater., 2017, 29, 1606829 CrossRef.
- W. Zhao, Z. He and B. Z. Tang, Nat. Rev. Mater., 2020, 5, 869–885 CrossRef CAS.
- J. Yuan, S. Wang, Y. Ji, R. Chen, Q. Zhu, Y. Wang, C. Zheng, Y. Tao, Q. Fan and W. Huang, Mater. Horiz., 2019, 6, 1259–1264 RSC.
- Y. Tao, R. Chen, H. Li, J. Yuan, Y. Wan, H. Jiang, C. Chen, Y. Si, C. Zheng and B. Yang, Adv. Mater., 2018, 30, 1803856 CrossRef.
- S. Xu, R. Chen, C. Zheng and W. Huang, Adv. Mater., 2016, 28, 9920–9940 CrossRef CAS.
- P. She, J. Duan, J. Lu, Y. Qin, F. Li, C. Liu, S. Liu, Y. Ma and Q. Zhao, Adv. Opt. Mater., 2022, 10, 2102706 CrossRef CAS.
- W. Zhao, Z. He, J. W. Lam, Q. Peng, H. Ma, Z. Shuai, G. Bai, J. Hao and B. Z. Tang, Chemistry, 2016, 1, 592–602 CrossRef CAS.
- J. Yuan, R. Chen, X. Tang, Y. Tao, S. Xu, L. Jin, C. Chen, X. Zhou, C. Zheng and W. Huang, Chem. Sci., 2019, 10, 5031–5038 RSC.
- X. Wang, H. Shi, H. Ma, W. Ye, L. Song, J. Zan, X. Yao, X. Ou, G. Yang and Z. Zhao, Nat. Photonics, 2021, 15, 187–192 CrossRef CAS.
- Y. Tao, C. Liu, Y. Xiang, Z. Wang, X. Xue, P. Li, H. Li, G. Xie, W. Huang and R. Chen, J. Am. Chem. Soc., 2022, 144, 6946–6953 CrossRef CAS PubMed.
- J. Wei, B. Liang, R. Duan, Z. Cheng, C. Li, T. Zhou, Y. Yi and Y. Wang, Angew. Chem., Int. Ed., 2016, 55, 15589–15593 CrossRef CAS PubMed.
- D. Li, F. Lu, J. Wang, W. Hu, X.-M. Cao, X. Ma and H. Tian, J. Am. Chem. Soc., 2018, 140, 1916–1923 CrossRef CAS.
- X. Yan, H. Peng, Y. Xiang, J. Wang, L. Yu, Y. Tao, H. Li, W. Huang and R. Chen, Small, 2022, 18, 2104073 CrossRef CAS.
- R. Kabe and C. Adachi, Nature, 2017, 550, 384–387 CrossRef CAS PubMed.
- G. Huang, Z. Deng, J. Pang, J. Li, S. Ni, J. A. Li, C. Zhou, H. Li, B. Xu and L. Dang, Adv. Opt. Mater., 2021, 9, 2101337 CrossRef CAS.
- T. Zhang, X. Ma, H. Wu, L. Zhu, Y. Zhao and H. Tian, Angew. Chem., Int. Ed., 2020, 59, 11206–11216 CrossRef CAS PubMed.
- X. Zheng, Y. Huang, W. Lv, J. Fan, Q. Ling and Z. Lin, Angew. Chem., Int. Ed., 2022, 61, e202207104 CAS.
- D. L. Dexter, J. Chem. Phys., 1953, 21, 836–850 CrossRef CAS.
- A. Khrebtov, E. Fedorenko, L. Lim and V. Reutov, Opt. Spectrosc., 2018, 124, 68–71 CrossRef CAS.
- C. Cebrián and M. Mauro, Beilstein J. Org. Chem., 2018, 14, 1459–1481 CrossRef.
- H. Uoyama, K. Goushi, K. Shizu, H. Nomura and C. Adachi, Nature, 2012, 492, 234–238 CrossRef CAS.
- K. Zhang, Q. Sun, Z. Zhang, L. Tang, Z. Xie, Z. Chi, S. Xue, H. Zhang and W. Yang, Chem. Commun., 2018, 54, 5225–5228 RSC.
- E. Baranoff and B. F. Curchod, Dalton Trans., 2015, 44, 8318–8329 RSC.
- E. J. Nam, J. H. Kim, B.-O. Kim, S. M. Kim, N. G. Park, Y. S. Kim, Y. K. Kim and Y. Ha, Bull. Chem. Soc. Jpn., 2004, 77, 751–755 CrossRef CAS.
- S. O. Jeon, S. E. Jang, H. S. Son and J. Y. Lee, Adv. Mater., 2011, 23, 1436–1441 CrossRef CAS PubMed.
- Q. Zhang, B. Li, S. Huang, H. Nomura, H. Tanaka and C. Adachi, Nat. Photon., 2014, 8, 326–332 CrossRef CAS.
- J. Lee, K. Shizu, H. Tanaka, H. Nomura, T. Yasuda and C. Adachi, J. Mater. Chem. C, 2013, 1, 4599–4604 RSC.
- S. d’Agostino, F. Grepioni, D. Braga and B. Ventura, Cryst. Growth Des., 2015, 15, 2039–2045 CrossRef.
- H. Gao, B. Ding, C. Wang and X. Ma, J. Mater. Chem. C, 2021, 9, 16581–16586 RSC.
- R. M. Clegg, Lab. Tech. Biochem. Mol. Biol., 2009, 33, 1–57 Search PubMed.
|
This journal is © The Royal Society of Chemistry 2023 |
Click here to see how this site uses Cookies. View our privacy policy here.