DOI:
10.1039/D2TC03318F
(Review Article)
J. Mater. Chem. C, 2023,
11, 97-112
Flexible photoplethysmographic sensing devices for intelligent medical treatment
Received
7th August 2022
, Accepted 20th November 2022
First published on 28th November 2022
Abstract
The beat frequency, flow rate, and oxygen content of human pulsating blood are important health indicators. For early diagnosis of diseases and real-time monitoring of cardiovascular health in specific practical scenarios, noninvasive methods are used that reduce pain and the risk of infection, but they are not conducive to wound healing. In this field, blood oxygen saturation in humans is obtained based on the use of the medium absorption principle of Lambert Beer's law. Owing to the absorption of light by different substances in the blood, the pulse rate constitutes an important index that is used to assess the status of the cardiovascular system. This study summarizes the research outcomes of sensor devices used for blood oxygen and pulse rate signal monitoring, based on the photoplethysmographic (PPG) test principle, and the main technical means and intelligent application scenarios for improving the shape adaptability, test stability, and biological compatibility of these devices. Nowadays, fabrication technologies are capable of realizing real-time stability in complex practical applications. Based on the research results and the related development status in the field of flexible electronics, some enlightening prospects for the future development of flexible PPG sensors are presented.
1. Introduction
Over the past several decades, the recording of physiological parameters of the human body using noninvasive methods has been the focus of research in the field of flexible wearable electronics.1–7 Medical research results have shown that the high prevalence and mortality of cardiovascular and cerebrovascular diseases seriously threaten human health.8–12 As many as 15 million patients die worldwide owing to cardiovascular diseases every year.13 As the disease develops very rapidly, treatment becomes difficult.14–17 Blood oxygen saturation measured based on the pulse rate is a critical biomarker of the health statuses of the human heart and cerebrovascular system.18–23 For patients with coronary heart disease, hypertension, and respiratory diseases, real-time monitoring of blood oxygen saturation and pulse rate is crucial to avoid medical emergencies.24,25 Meanwhile, for those who live or work in extreme environments for a long time, such as pilots and astronauts, monitoring of blood oxygen saturation also introduces some application requirements.26–38 Wearable flexible electronic devices exhibit good ductility,39–41 shape adaptability42–44 and biocompatibility.10,33,45–50 In this study, some results have been obtained by flexible photoelectric detection methods that allowed the acquisition of photoplethysmography (PPG) signals, which are capable of reflecting human blood oxygen saturation.
Photoelectric volumetric measurement is the most representative noninvasive blood oxygen monitoring method.51–60 This method uses the absorption rate of human vascular tissue at different wavelengths to characterize the corresponding physiological parameters. This constitutes a potential application of flexible photoelectric sensors in the field of auxiliary medical treatment.61,62 The flexible blood oxygen probe is mainly composed of the following parts: flexible and wearable substrate,63–67 light sources based on different emission wavelengths,68–72 and photodetectors73–78 that can quickly respond to the optoelectric signal in the blood vessels in tissues. According to the differences in the distribution of light sources and photodetectors, the flexible blood oxygen probe operation based on the photoelectric principle can be classified into two important modes, namely the transmission and the reflection modes. Compared with the sensors based on reflection mode, the requirements for the concentration and thickness of the object of the device are much lower than those of the transmission mode. Additionally, all functional modules of the reflection devices are in the same plane, which improves the wearability of the device and the stability of real-time signal monitoring.79–83 Among the current research results, considering the stability of signal extraction and the overall power consumption of devices, most flexible PPG signal sensors adopt the reflection mode signal extraction method. A flexible wearable device capable of real-time monitoring of human microphysiological signals must have good shape adaptability, biological compatibility, good wearability, and stability of real-time monitoring signals.84–89
Moreover, considering the sustainability of energy consumption during the device's operation, a self-powered flexible monitoring system will be realized if both mechanical and light energies generated from the human body or natural environment can be transformed into the energy required to drive the PPG sensor.90–101 According, the collection and transformation of green energy is of great research significance from the perspective of environmental friendliness and energy conservation.102–107 There is a certain demand for the development of flexible triboelectric nanogenerators with high conversion efficiency which can be effectively integrated with optoelectronic devices and flexible solar cells based on the photovoltaic effect.108–115 In this regard, studies have contributed, but there is still room for performance optimization.
Additionally, during clinical treatment of patients, such as surgery and interventional therapy, the PPG signal is very important for the real-time monitoring of patient's recovery to ensure wound healing and the recovery of heart and brain blood supply.13,80,116–121 In cardiac and brain surgeries, the oxygen content in the heart or brain can be monitored and the postoperative wound can be recovered by collecting PPG signals.122 Based on the preparation and study of noninvasive monitoring at the present stage, a microcatheter PPG signal sensor has been designed to realize stable operation when placed on the surfaces of the mouse heart and brain, given the improvement in the device's material performance stability and the optimization of the device in terms of biological compatibility and minimal size.123–128 These works provide an excellent possibility to apply implantable blood oxygen pulse detectors in human cardiovascular interventional therapy. Based on this consideration, it is necessary to realize the stability of device performance and real-time monitoring from the perspective of the biocompatibility and implantability of the device.
In this study, the specific content of the article is predicted as follows: first, from the perspective of the application and development of flexible photoelectric detection based on PPG signal monitoring, this study compares representative research results and summarizes the current research status in combination with the developmental needs of flexible wearable optoelectronic devices applied in the medical field (Fig. 1).42,44,54,59,61,99,122,123,126 Subsequently, a more detailed discussion is presented from the aspects of materials, functional structure, working principle and cutting-edge applications. Finally, considering the current material synthesis technology and the research status of flexible manufacturing methods, the future development of flexible blood oxygen saturation detection is envisaged to progress from the perspectives of bionics, multifunctional sensing integration, and biocompatibility.
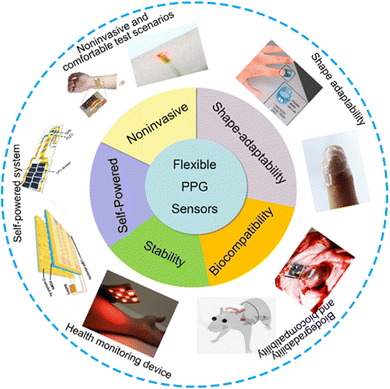 |
| Fig. 1 The device characteristics of using flexible PPG signal to monitor human blood oxygen signal are summarized. the advantages and important performance indexes of flexible PPG signal sensor. Noninvasive and comfortable test scenarios: reproduced with permission.61 Copyright 2020, Oxford University Press. Reproduced with permission.54 Copyright 2017, American Chemical Society. Shape adaptability image: reproduced with permission.44 Copyright 2014, Springer Nature. Reproduced with permission.59 Copyright 2017, John Wiley and Sons. Biodegradability and biocompatibility image: reproduced with permission.126 Copyright 2021, American Association for the Advancement of Science. Reproduced with permission.123 Copyright 2018, Spring Nature. Stability image: reproduced with permission.42 Copyright 2018, PNAS. Self-powered image: reproduced with permission.99 Copyright 2019 MDPI, Reproduced with permission.122 Copyright 2021, Spring Nature. | |
2. Wearable and flexible smart device
Photoelectric detection for obtaining the number ratio of oxygenated blood cells is a representative noninvasive monitoring method. As a high-performance flexible wearable device, it needs to have the following characteristics; good character adaptability, scalability, biological compatibility, high stability, and low-power consumption.78–83,90–100 For real-time monitoring of the blood oxygen saturation signal of the human body in complex exercise states and the physiological environment, the device must have good environmental adaptability to ensure that the monitored electrical signal can accurately represent the human health status in real time for early diagnosis and intervention of cardiovascular diseases. To achieve the required performance of the flexible devices, extensive work has been conducted on (among others) the design of the interconnection of devices into a graphic structure that can withstand external tensile strain to realize the ductility of devices and use two-dimensional materials with performance advantages to improve the sensing performance of devices.24–36 Based on this, integrating the sensors with other energy conversion devices to realize the self-power supply function is a new attempt in flexible energy-intersive systems. The aforementioned contents are discussed and highlighted in detail in this section. In Table 1, we compared the reported representative flexible PPG sensors from the perspectives of basic performance of photodetectors, the power and wavelength of the light source, material types of device and mechanical stability.
Table 1 Main functional type and structural composition of flexible wearable PPG sensors and responsive optoelectronic device
Device composition |
Wavelength of the light source (nm) |
Materials of the photodetector |
Method for realizing shape adaptability |
Flexibility and ductility |
Ref. |
Single wavelength LED with PD |
620 nm |
Silicon based |
Flexible encapsulation technology |
The device can withstand a bending curvature of 2.7 mm |
54
|
OLED&OPD arrays (2 × 2 pixels) based on multi-wavelengths |
612 and 725 nm |
PC71BM/TMB:BB/PEDOT:PSS |
Flexible encapsulation technology with flexible device based on organic materials |
The device can be slightly bent |
42
|
Multi wavelength lighting source with single IPD |
850 and 620 nm |
Silicon based |
Island Bridge stress protection structure& Flexible encapsulation technology |
The device can withstand certain tensile strain |
59
|
79
|
99
|
Muti wavelength LEDs with IPD |
640 and 950 nm 515 and 620 and 850 nm |
Silicon based |
Stretchable graphical interconnection and encapsulation material |
The device can withstand 30% and 40% tensile strain respectively |
54
|
|
|
|
|
|
61
|
Multi wavelength LEDs with flexible GQD PD. |
630 and 940 nm |
Graphene and PbS colloidal quantum dot |
Flexibility of colloidal QD thin film and the flexible encapsulation technology |
The device can withstand a bending curvature of 16 mm for 2000 times |
41
|
Organic phototransistor(OPT) with single wavelength LED |
810 nm |
DPP-DTT/PCBM |
Flexible encapsulation technology and the intrinsic flexibility of organic materials |
The device can withstand certain bending |
66
|
Muti wavelength LEDs with flexible IPD based on radial junction structure |
520 and 655 and 800 nm |
a-SiGe radial junction over the SiNW |
Certain stress bearing capacity of radial junction of the PD |
The device can withstand a bending curvature of 25 mm for 1000 times |
75
|
Flexible organic PD with single wavelength LED |
Red |
P3HT:ICBA/PEIE |
Flexibility of the OPD materials |
The device can withstand certain bending |
77
|
Flexible polymer PD with mult wavelength LEDs |
660 and 850 nm |
PEDOT:PSS/PMBBDT/Y6 |
Flexibility of the polymer photodetector |
The device can be bent to be well attached to the finger surface |
76
|
Flexible QD-LED and QD PD based on wrinkled structure |
618 and 520 and 453 nm |
PbS quantum dot |
Ductility of intrinsic mechanical structure (wrinkle) of optoelectronic devices |
QD-LED and PD can withstand 70% and 30% tensile strain respectively |
81
|
Flexible OPD with multi wavelength LEDs |
520 and 610 nm |
BmPyPB/C70:TAPC/HAT-CN |
Intrinsic flexibility of OPD and OLED materials |
The device can be well attached to the skin surface |
123
|
All organic optoelectronic sensor |
532 and 626 nm |
PTB7:PC71BM |
Intrinsic flexibility of OPD and OLED materials. |
The device can be well attached to the finger surface |
44
|
Flexible OPD with multi wavelength PLED |
517 and 609 nm |
P3HT:PCBM |
Intrinsic flexibility of OPD and PLED |
PLED can withdraw 100% compression and 100% tensile strain and OPD can withdraw 40% compression |
53
|
μ-IPD with multi wavelength μ-ILEDs |
625 and 540 nm |
Silicon |
Flexible encapsulation technology with graphical stretchable interconnect |
The device can bend on the surface of organs and tissues |
126
|
Commercial silicon PD with multi wavelength ILEDs |
645 and 950 nm |
Silicon |
The encapsulation process with biological silica gel catheter to realize flexibility |
The device can withdraw a bending curvature of 27 mm |
128
|
Flexible OPD with single wavelength OLED |
Near IR |
PIPCP:PC61BM |
|
The device can be stretch to 200% of the original length |
50
|
2.1 Shape adaptability optimization of the device through the mechanic's design
For outstanding wearability and real-time stability of flexible PPG sensors, shape adaptability is pursued for the device structural design, especially on surfaces with unstable morphologies, such as human finger joints or body muscles, which can achieve good adhesion onto the skin surface.14 However, it is difficult to avoid any discomfort during long-term real-time monitoring. Considering the elasticity and ductility of the human skin, the device needs to have a large bending curvature and extensibility within a certain range to achieve good shape adaptability on the object‘s surface. In recent years, several attempts have been expended on the structural designs of devices to improve their shape adaptability. The flexible, ultrathin structure composed of an organic polymer substrate and the stretchable graphical interconnection device structure are representative methods used to accomplish this task. The outcomes of these attempts are demonstrated in the following sections, and the key points of the research are discussed in detail.
2.1.1 Flexible device based on ultra-thin structure.
Compared with traditional rigid devices, flexible wearable devices have good adhesion onto irregular surfaces, such as human muscles or joints. Therefore, during real-time monitoring, flexible wearable devices cause less discomfort in long-term use. Polydimethylsiloxane, polystyrene, and other organic polymers are often used as flexible packaging materials; in particular, cured elastomeric films have good elasticity, biocompatibility, and insulation properties.8–13,15–19,52–60 Meanwhile, if the thickness of the device's functional body and packaging layer is ultra-thin (1 μm), the overall flexibility of the device is improved to achieve a large bending curvature. Fig. 2a and b shows representative flexible PPG sensors based on blood oxygen signal detection and luminescent visualization.53 A flexible ultra-thin PPG signal sensor based on red and green, dual-wavelength, organic light-emitting diodes (OLEDs), is proposed. OLEDs are prepared on a parylene substrate with a thickness of only 1 μm. The thickness of the ITO electrode is only 70 nm, thus ensuring the ultra-thin size and adhesion properties of the device. Compared with organic materials, inorganic materials have higher luminous efficiency and better photoelectric properties, especially in the near-infrared light-emitting band with deep penetration depth of human skin.53 Although inorganic light-emitting diodes are mostly based on rigid materials and structures, However, by reducing its thickness and using flexible encapsulation technology, the corresponding devices can still be flexible and wearable to meet the needs of daily monitoring. Fig. 2c proposes an all inorganic PPG sensor based on single light source.54 In this study, a flexible PPG signal sensor (620 nm) is reported, which uses an inorganic light-emitting diode (LED) light source based on a red light band and micro IPD (μ-IPD) photoelectric signal detection of GaAs-based materials; the device is transferred to the metal graphic interconnection structure through the PDMS stamp transfer process, which is attached to the polyimide (PI) substrate by SU-8 photoresist, and finally encapsulated with ecoflex material with good ductility, so that the overall thickness of the device is 300 μm. The device still maintains good performance at bending curvature of 3 mm.54 In addition, the photoelectric performance of the device still has no clear attenuation after bending 1000 times at this bending curvature. In Fig. 2d, a 2 μm thick PI material is used as the flexible substrate of a dual-wavelength LED light source and a photodetector (PD) silicon detector, which can achieve good adhesion on the fingers.59 Feng's group proposed the use of three wavelengths (515 nm, 620 nm, 850 nm) based on red, near-infrared, and green light flexible PPG signal sensors for inorganic optoelectronic devices (Fig. 2e).61 In this study, nano-diamond grinding technology was used to thin the light source and PD to reduce the overall thickness of the device. The device was encapsulated with 3 M material, which has with good biocompatibility and a thickness of only 30 μm. Good biocompatibility and shape adhesion were realized.61
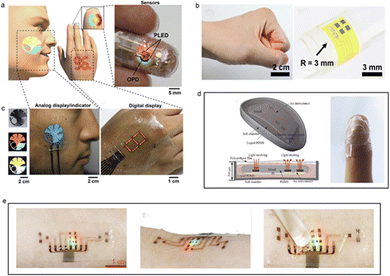 |
| Fig. 2 List of research results of wearability of devices using ultra-thin organic substrates. (a and b) Flexible PPG sensor (1 μm) based on dual wavelength ultra-thin OLED, OPD and ultra-thin parylene substrate. Reproduced with permission.53 Copyright 2016, American Association for the Advancement of Science, (c) flexible device (300 μm) based on ultra-thin PI substrate and red LED based on GaAs materials. Reproduced with permission.54 Copyright American Chemical Society. (d) Using PI Schematic diagram of all inorganic dual wavelength blood oxygen probe encapsulated in material (500 μm). Reproduced with permission.59 Copyright 2017, John Wiley and Sons. (e) Of three wavelength all inorganic flexible blood oxygen sensor encapsulated on 3 M stretchable biocompatible substrate (30 μm). Reproduced with permission.61 Copyright 2020, Oxford University Press. | |
2.1.2 Stretchable PPG sensors.
Human skin is an elastic and extensible biomaterial. When the human body undergoes complex motion, the human skin also expands and distorts, which is more evident in certain areas, such as wrist joints.68 Considering the above situation, it is difficult for a flexible device to meet the demands of practical applications. In recent years, several exploratory studies have been conducted to improve the ductility of flexible electronic devices, and several representative mechanical models have been proposed.72–78 Among them, the island bridge structure, which changes the local elastic modulus of the material to realize stress protection with a graphical structure prepared with conductive materials, is the most common method. These devices have been extensively used in the mechanical isolation of flexible electronic devices. In the cases of flexible PPG sensors with blood oxygen detection functions, some phased achievements have been expended in recent years in the realization of device extensibility. Fig. 3 shows representative achievements. In these studies, two crucial graphical stress concentration interconnection structures (serpentine and watch chain) were proposed. Under large applied tensile strain, the device maintains a good electrical signal output. On the premise of achieving the same ductility as the human epidermis, the accurate monitoring of blood oxygen signals can be realized.
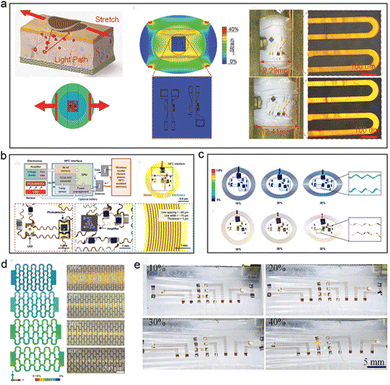 |
| Fig. 3 Implementation method of stretchable flexible PPG signal sensor. (a) It is a stretchable device with graphical interconnection and functional part of the device and graphical packaging of island bridge structure. Reproduced with permission.59 Copyright 2017, John Wiley and Sons. (b) The utility model relates to a stretchable device for stress protection by using a snake wire stretchable interconnection. Reproduced with permission.60 Copyright 2016, American Association for the Advancement of Science. (c) The simulation results of the stress distribution of the device under 10–30% applied tensile strain. (d) A stretchable device based on 3 M high ductility substrate with watch chain graphical interconnection (e) The physical diagram of the device corresponding to (d). Reproduced with permission.61 Copyright 2020, Oxford University Press. | |
An inorganic flexible PPG sensor based on an island bridge structure was proposed by Feng et al. (Fig. 3a).59 In this study, the main functional sensing parts were encapsulated with thicker organic materials, while the thickness of the encapsulation materials in the surroundings was much smaller than those in the central areas.59 This island bridge structure induced a stress protection effect in the middle parts of the photoelectric sensor. From the mechanical simulation results, when a specific amount of tensile strain was applied to the device, the stress was mainly concentrated in the non-functional component area.
In 2016, Rogers et al.60 proposed a stretchable, battery-free, wireless human body signal characterization photoelectric system (as shown in Fig. 3b and c), which realized good shape adaptability on the surface of human skin and monitored the PPG signal under deformation conditions, such as tension and bending.60 This study used an NFC interface system with a wireless battery-free structure to charge the device, and red LED, near-infrared LED and silicon PD to form a dual-wavelength test system that can monitor the response of blood tissue to photoelectric information. The interconnection of optoelectronic devices is designed into a serpentine-shape, graphical mechanical structure (width: 60–90 μm, and thickness 5 μm) that can withstand the external tensile strain, and the device is encapsulated with PI material to realize insulation protection and flexible design.60 According to the results of finite element simulation, when the device is subjected to external tensile strain, the main stress is distributed in the serpentine graphical interconnection area, which realizes stress protection for optoelectronic devices such as LEDs and PDs to avoid damage to their structure by external stress. In this case of Rogers's group, the device can withstand more than 30% of the applied tensile strain and realize the requirements of dual shape adaptability, such as bendability and extensibility.60
Feng et al.61 proposed a skin-like optoelectronic system based on watch-chain graphical interconnection structure in 2020 (Fig. 3d and e).61 Compared with the devices with a serpentine graphics structure, the interconnection structure composed of watch-chain graphics has greater ductility, and its maximum tensile range is increased to 60%. Meanwhile, this device can maintain precise sensing performance at external tensile strains <40% with lower power consumption compared with serpentine-like interconnection. This optoelectronic system consisted of LEDs with three wavelengths (850 nm, 620 nm, and 515 nm), four silicon-based photodetectors (400–1100 nm), and a flexible circuit that is used in signal processing and wireless transmission.61 The device accurately measured the human diastolic systolic blood pressure and blood oxygen saturation, which can be used for invasive real-time monitoring.
2.1.3 Flexible PPG sensors based on stretchable optoelectronic devices.
In the preface chapters, many technical means to realize the flexibility of devices are introduced in details. The main experimental methods can be summarized as designing the corresponding mechanical concentration structures to realize the mechanical protection of rigid optoelectronic devices, so as to avoid the damage of external stress to the performance of devices while realizing the expansibility. Moreover, the change of external stress environments is uncontrollable in the practical application scenario.96 On the purpose of realizing the shape adaptability of the device and the anti-interference of external force signal, the proposal and development of photoelectric devices with certain ductility are especially significant. In addition to the intrinsic flexibility of organic optoelectronic materials, there are several effective methods which are capability of introducing ductile structures on the device surface. Fig. 4 demonstrates two types of flexible PPG sensors based on full stretchable optoelectronic devices. In Fig. 4a–c. Kim. et al.81 prepared a full stretchable quantum dot LED based on wrinkled structure by using the difference of elastic modulus between photoelectric thin film (TiO2/colloidal quantum dot/TFB/PEDOT/graphene) and the pre stretched ecoflex elastic substrate.81 The LED has good ductility and stable luminescence performance. Under the external tensile stress of 70%, the device can still achieve the luminous intensity of more than 1000 dc m2. Furthermore, the photoelectric performance of the device remains outstanding under the repeated tensile stress. Then, the stretchable PD based on PbS QD was prepared by the same experimental method. The device can withstand 40% tensile strain with the best responsivity of 0.13 A W−1.81 The full stretchable PPG sensor formed by combining the above two wrinkle-based optoelectronic devices has excellent wearability and working stability. Compared with the PPG sensor based on commercial rigid LED, the noise of PPG signal has been significantly improved. In the study of ultraflexible photonic skin, Someya et al.53 proposed a flexible polymer LED based on crumpled structure (Fig. 4d–f).53 The Luminance of the device can still maintain above 95% of the initial value after 1000 cycles of 60% external tensile strain. The stretchable PLED will be a promising choice for the light source design of PPG sensor. Therefore, on the basis of the comprehensive consideration of shape adaptability and performance stability of the device. It is of great research value to optimize the basic performance of optoelectronic devices from the intrinsic mechanical structure.53
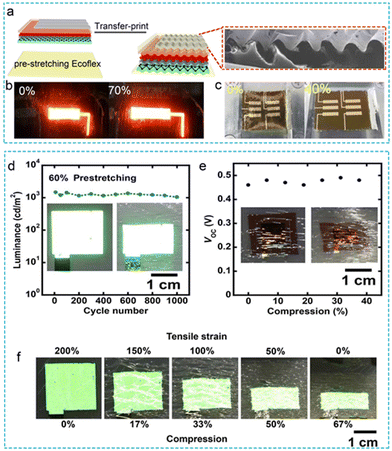 |
| Fig. 4 Stretchable flexible optoelectronic device applied to PPG sensor. (a) Fabrication of wrinkled structure by pre stretching process and transfer printing. (b and c) The physical diagram of the stretchable QD-LED and QD-PD. Reproduced with permission.81 Copyright 2017, American Chemical Society. (d and e) The Photoelectric properties of PLED and OPD in deformation state. (f) The process of winkle formation on PLED and the physical diagram of the device returning to the plane state. Reproduced with permission.53 Copyright 2018, American Association for the Advancement of Science. | |
2.2 Performance optimization of flexible optoelectronic devices
The preface thoroughly discusses ways to realize the shape adaptability of the device from the aspects of optimizing the graphical mechanical structure and thinning of the overall thickness of the device along with ways to resist the interference of external stress on the performance stability of the device.101 As a flexible optoelectronic device with sensing performance, to obtain more accurate test performance, the optimization of the optoelectronic characteristics of the device itself is particularly important.102 Owing to their excellent photoelectric properties, two-dimensional materials have more response time and detection rate performance optimization characteristics compared with traditional silicon-based and GaAs-based semiconductor PDs. Similarly, compared with traditional bulk materials, two-dimensional materials are more flexible and show great potential in meeting the developmental trend of wearable flexible devices.
2.2.1 Flexible PPG signal sensor based on performance-enhanced photodetector (PD).
Currently, for flexible wearable PPG sensing devices, the commonly used photodetector is a rigid photodetector based on silicon or GaAs materials. Owing to the characteristics of the material, such as hardness and stability, accuracy decreases in some test scenarios due to motion or external forces because of the weak shape adhesion, although the conventional rigid detectors can accurately detect optoelectronic information reflected by the human skin.41–43,66–70 The extraction environment of PPG signals is usually complex. In addition to the common in vitro test environment, such as the wrist, finger, and neck, there is a complex real-time monitoring environment implanted in patients during surgery. this introduces forward response requirements for the extension and flexibility of the device. To improve the comprehensive performance of flexible PPG sensors, preparing the detectors with both scalability and the excellent photoelectric response has become a mainstream development direction. Fig. 5 shows a representative flexible photodetector applied to PPG signal sensing at this stage, which improves the photoelectric performance on the premise of ensuring ductility in terms of material, structure, etc. Graphene sensitive with semiconductor quantum dot (GQD) material has a wide wavelength response range (300–2000 nm) and a high-response rate. In Fig. 5(a–c), graphene prepared by the CVD process is combined with PbS quantum dot materials to prepare 30 nm ultra-thin films.41 A flexible photodetector that can monitor the response of the human body to a PPG signal over a wide wavelength range is prepared by combining the GQD ultrathin film with a flexible substrate. The flexible detector is integrated with a green LED (535 nm) in the wearable bracelet, which can detect the pulse rate of the human body, and the value of blood oxygen saturation can be obtained by integrating it with the red near-infrared dual-wavelength LED.41 The GQD detector can withstand the developed strain of a 16 mm bending curvature up to 2000 times and still maintains good detection performance. The sensitivity and other detection performances of the device were improved considerably through local gate control, and the maximum response rate reached values of the order of ∼105 A W−1.41 On the premise of good photoelectric performance, it can simultaneously realize good shape adaptability and biological compatibility.
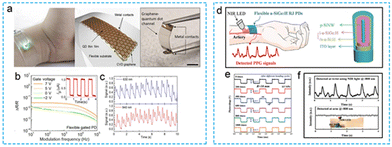 |
| Fig. 5 Flexible photodetactor Based on inorganic materials for flexible PPG signal monitoring. (a–c) Physical diagram, working principle, application scenario and signal acquisition under different working modes of flexible PPG signal sensor based on GQD photodetector. Reproduced with permission.41 Copyright 2019, American Association for the Advancement of Science. (d–f) Physical diagram, working principle, application scenario and signal acquisition of flexible PPG signal sensor based on 3D a-SiGe: H p–i–n radial junction. Reproduced with permission.75 Copyright 2021, Wiley-VCH Verlag. | |
Based on the stability and broad spectral response range of SiGe materials, PDs with a three-dimensional (3D) SiGe radial structure made of P-I were prepared by the PECVD process and VLS growth method to realize the optoelectronic response to the light source (Fig. 5d–f).75 Owing to the mechanical advantages of the radial structure, the device has good flexibility and mechanical stability, which is sufficient to withstand 1000 continuous bending cycles with a bending curvature of 10 mm.75 Meanwhile, the device has a superior response time (5.4/17.6 μs) and high sensitivity of 140 mA W−1 (at 800 nm) to meet the monitoring requirements associated with PPG signal recordings at human wrists at 655 nm, 520 nm and 800 nm.
In the daily PPG signal application scenario, some of the test light source signals will be responded by the photodetector after the absorption, reflection or transmission of the human blood tissue. However, most of the light sources will be scattered in the application environment and cannot be captured by the photodiode. Similar to the other photoelectric signals of human beings, the monitoring of the PPG signal is easily disturbed by the test environment as well.103 Therefore, in order to obtain ideal signal characterization results, it is necessary to add filtering and amplification circuits at the back end of the photodetector to ensure the accuracy of real-time monitoring. However, the design and preparation of the back-end signal processing circuit will also increase the complexity and the overall of the sensing system, which may reduce the wearability.104–106 Based on this consideration, it is significant to introduce a high response photodetector with weak light detection ability into the PPG signal sensing system. Fig. 6 demonstrates two organic photodetectors from the perspective of materials, structures and performance in details. In addition, combined with the developmental needs of faint light detection in recent years, multiplier polymer photodetectors (PM PPDs) have attracted considerable attention. Faint optical signals from the human body play an important role in the field of health monitoring. Based on the above considerations, Zhang et al.76 proposed a flexible optoelectronic detector for monitoring blood oxygen saturation based on the PMBBDT:Y6 binary system material in 2021 (Fig. 6a–c).76 The spectral response range of the device ranges from ultraviolet to near-infrared, and the ultraviolet quantum efficiency can reach 37300%. PM PPDs can obtain PPG signals with peaks of 0.17/0.38 μA, and the accuracy of human physiological signal parameters such as pulse rate and blood oxygen saturation can be compared with those obtained by commercial PPG sensors. Similarly, in 2021, the Duan's group77 proposed a large-area low-noise flexible organic PD based on a P3HT:ICBA photosensitive layer, which can be used to detect faint, visible light (Fig. 6d and e).77 The low-noise current of the device can be as low as 37 fA. On this premise, given that 1.8 × 1012 cm Hz1/2 W−1 is a high-detection rate, and the effective detection area on a flexible substrate is comparable with that on a rigid substrate, the PPG signal can be detected in real time when the device is exposed to a 26 mW red light source by increasing its area by 10 times (1 cm2).77 The device can achieve nearly the same performance as a commercial rigid small-area silicon photodetector. Meanwhile, the flexibility of the flexible device increases wearability. This research provides a reference for OPD devices to realize weak, visible light detection in the future. The enhanced-performance PDs mentioned above have great application potential in the field of wearable electronic devices for monitoring human physiological signals.
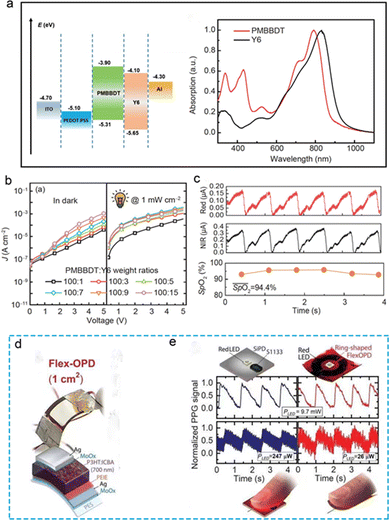 |
| Fig. 6 Flexible photodetector Based on organic materials for flexible PPG signal monitoring. (a–c) Physical diagram, working principle, basic photoelectric performance, application scenarios and signal acquisition of PM PPDs flexible PPG signal sensor based on PDBBDT:Y2 binary system. Reproduced with permission.76 Copyright 2021, Science China Press. (d and e) Physical diagram, application scenarios and acquisition of organic polymer Photodetector Based on P3HT:ICBA blend membrane PEIE-MoO3. Reproduced with permission.77 Copyright 2021, American Association for the Advancement of Science. | |
2.2.2 Flexible photoelectric signal sensor based on μ-LED and μ-IPD.
With the gradual development of micro–nano processing technology in recent years, semiconductor optoelectronic devices have also been developed toward the appearance of small sizes and volumes. Micro-LEDs (micron sized) have the advantages of higher external quantum efficiency, lower switching voltage, and higher power density.54,117 Their good luminous and power consumption performance ensure that attenuation the photoelectric signal is extremely faint in biological tissues and stable model output can be obtained under complex physiological environment. Meanwhile, micro-LEDs (μ-LEDs) and μ-IPDs have a small volume, which also determines that they have good integration and can be assembled into microprobes with good shape adaptation, implantability, and sensing components of the microcatheter structure.54,80,127 In 2013, Rogers group proposed a method to assemble blue light μ-LED and micro size μ-IPD on the surface of photoelectric implantable microneedle.117 As illustrated in Fig. 7a–c. The μ-LED in this sensor can irradiate all biological tissues in its emission area, on this basis, the limitations of the radiation area of the original optical fiber light guide sensor can be eliminated effectively. Furthermore, the power consumption of micro light source is only 40 mW during experiment, which is less than a number of conventional bulk LEDs in past studies. After implantation into rat brain, multifunctional sensor components can work together to obtain corresponding optogenetic information. At the same time, the use of μ-IPD non-invasive wearable photoelectric sensor has also been reported in the decade (Fig. 7d). The μ IPD array is integrated with a single wavelength light source (red) to realize real-time monitoring of human pulse PPG signals. Fig. 7e–h depict the flexible PPG signal sensor with a microneedle structure based on a micro-LED and micro-IPD (540 nm, 635 nm).128 The full-width at half-maximum values of the two wavelength μ-LEDs at their respective central wavelengths are 10 nm and 30 nm, respectively, and the external quantum efficiency of μ-IPD in the wavelength range can reach 74% at 540 nm and 82% at 635 nm.128 The device had a size of only 400 μm and an extremely lightweight mass of 80 mg. The device uses a wireless transmission module to supply energy to the micro-LED array, which reduces the overall volume of the device and the wound damage to the body during implantation. Meanwhile, compared with large-size, light-emitting components, micro-LED have less heat dissipation, avoid the damage of local high temperatures to the surface of biological tissue, and help improve the lifetime of the device.
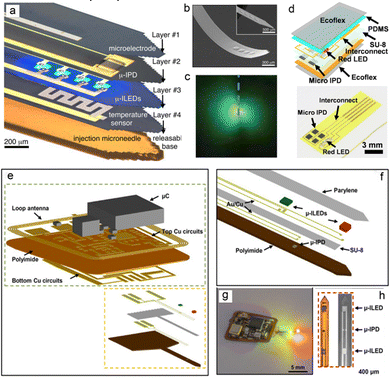 |
| Fig. 7 Diagrams of flexible photoelectric signal sensing systems based on μ-LED and μ-PD. (a) Schematic diagram of implantable physiological model photoelectric sensor based on micronneedle size. (b and c) SEM morphology characterization and physical diagram of the micronneedle-type photoelectric sensor. Reproduced with permission.117 Copyright 2013, AAAS. (d) Patch type single light source flexible PPG signal sensor based on micro IPD array. Reproduced with permission.54 Copyright 2017, American Chemical Society. (e) The schematic diagram of the overall structure of the microprobe PPG signal sensor. (Reproduced with permission. (f) The detailed picture of device composition. (g and h) The physical diagram and SEM morphology of the micro sensor. Reproduced with permission.128 Copyright 2019, American Association for the Advancement of Science. | |
2.3 Flexible self-powered PPG sensors
Among the research results obtained, the flexible PPG sensor has improved its performance in many aspects such as materials, devices and mechanical structures. In the back-end algorithm, the photoelectric information is integrated and calculated, and information, such as blood oxygen, blood pressure, and pulse rate, was obtained that reflects the state of human cardiovascular health. The light-emitting elements in the device required an external power supply.94–101 From the perspective of energy conservation and sustainability, it is an ideal way to convert the easily available and environmentally friendly energy into electrical energy reserves and supply energy for photoelectric devices.101–104 In the current research state, self-powered PPG sensors have accomplished some research achievements by combining energy devices with photoelectric devices to realize energy collection, conversion and storage of the overall system. Flexible triboelectric nanogenerators (TENGs) and solar cells are common energy devices with stable performance and mature technology.94–106
Fig. 8 reveals the self-powered-wearable PPG signal sensing system composed of a flexible TENG and a flexible PPG sensing device. Chen connects the crumpled gold and PDMS friction layer to realize a flexible vertical-contact TENG with the rectifier circuit and energy storage battery (Fig. 8a–d), and then combined the structures above with the flexible IR-R dual wavelength PPG light source probe to form the self-powered sensing system.94 Through the periodic mutual contact separation between the crumpled gold (30 nm) and PDMS materials with different polarities, the pulse-mode electrical signal output is used to supply power to the R-IR dual wavelength LED continuously (Fig. 8c).94 The microstructure of the wrinkled morphology in the bottom electrode can increase the contact area, so as to enhance the output performance and stretchability of the device. The peak value of the output voltage reached a maximum charge density of 160 μC m−2. In the flexible PPG sensor, the light sources with two wavelengths of red light (630 nm) and near-infrared (850 nm) can be turned on alternately during TENG operation, and the silicon-based PD can characterize the absorption and reflection of human tissue to the light sources with the use of two wavelengths (Fig. 8d).94 The copper-based flexible TENG based on another surface micro morphology is introduced. In Fig. 8e and f Friction charge of this device is generated by the polarity difference between PEDOT:PSS and PTFE with a surface micro-histogram, so as to obtain the electrical signal output.100 In the experiment, the dielectric constant of the material is adjusted by doping porous carbon in PEDOT:PSS solution, and the mixed solution is spin coated on the pre stretched ecoflex flexible substrate to prepare the micro graphic structure which can improve the ductility and surface roughness.100 These two methods jointly promote the improvement of the output performance of the device. Subject to the action of a vertical external force, the maximum output voltage of the device can reach 74.3 V. Compared with crumpled Au, PEDOT:PSS based flexible TENG has simple fabrication technology and low cost, which is suitable for large-scale production and promotion.100
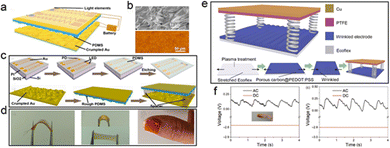 |
| Fig. 8 Schematic diagram of self powered flexible PPG signal sensor based on Triboelectric nanogenerator (TENG) system. (a–d) The schematic diagram, surface morphology characterization, fabrication process and the physical diagram of the all inorganic self powered flexible PPG blood oxygen detector integrated with crumpled Au film TENG. Reproduced with permission.99 Copyright 2019, MDPI. (e and f) The schematic diagram, the working principle and the physical diagram under working state of the flexible self-powered device integrated with wrinkled patterned PEDOT:PSS TENG. Reproduced with permission.100 Copyright 2022, Wiley-Blackwell. | |
Additionally, for converting mechanical energy into electrical energy, photovoltaic devices that convert light energy into electrical energy are also a highly reliable way to obtain electrical energy.119–123 With the development of technology, a certain number of phased achievements have been expended in the preparation and research of solar cells, which are simple and environmentally friendly. Meanwhile, with the in-depth study of material properties and the development of micro-nano processing technology, the energy conversion efficiency and integrability have improved considerably. Fig. 9a and b demonstrates self-powered sensing system, which combines solar cells with flexible PPG sensors. A yellow OLED with an 8-quinolinolato lithium-doped peie layer was prepared as the flip structure of the electron transport layer by the Sumeya group.122 The yellow OLED was used as the light source, and the OPD (ptzntz Bobo pc70bm 300–800 nm) and solar cell OPV were integrated to form an ultrathin, flexible, self-powered PPG signal monitoring system. As an energy device, organic solar cells have a high-energy capacity, and their power conversion efficiency (PCE) can reach 28.1% in a 1000 lux indoor lighting environment. In the experiment, 10 organic solar cells were connected in series and were used to provide energy for the OLED light source. The self-powered photoelectric detection system can detect the PPG signal of a human pulse beat well, which introduces a new design idea for flexible self-powered PPG sensors. As a photovoltaic device with high reliability and a certain research foundation, solar cells have been extensively used as energy supply units in flexible sensing systems. As shown in Fig. 9c–e, Sumeya's123 group integrated active area grating-enhanced organic photovoltaic devices and organic chemical transistors on an ultrathin parylene substrate with a thickness of 1 μm. A flexible self-powered test system with high-tensile and high energy conversion efficiency (PCE 10.5%) was realized.123 This structure combines one-dimensional patterned, double-grating, performance-enhanced organic solar cells with functional devices. On the premise of realizing high energy conversion efficiency, it also ensures the biological compatibility of the device, realizes the test of physiological signals on the surface of the skin and biological tissue, and avoids the damage of high temperature, caused by the traditional charging process, to the performance of functional devices.
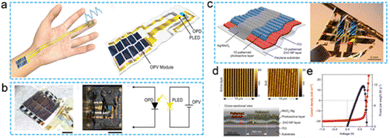 |
| Fig. 9 Schematic diagram of self-powered flexible PPG signal sensor based on organic photovoltage system. (a and b) Physical diagram and application scenario of organic flexible self-powered PPG signal sensor integrated with OPV. Reproduced with permission.122 Copyright 2021, Springer Nature. (c–e) Physical diagrams, Surface topography and Basic Photoelectric performance of ultra thin organic flexible PPG sensor integrated with solar cell. Reproduced with permission.123 Copyright 2016, Springer Nature. | |
3. Promising applications of flexible and wearable PPG sensors in health monitoring
Different types, concentrations and depths of substances have different absorption and reflection abilities to the light source in the flexible PPG sensor. Through the test of the reflected light by the photodetector, the physiological information reflecting the health status of human device tissue can be obtained, and some evaluation results can be obtained from the analysis of the test data. The main waveform of the PPG signal contains a sharp peak with distinct amplitude. The contraction and relaxation of human blood vessels and the flow of blood cells are accompanied by the pulse beating. Therefore, in non-invasive tests, the physiological parameters that can be obtained from the analysis of PPG signals include pulse rate, blood pressure, and blood oxygen saturation. In the case of the PPG sensor, the completion of the material synthetic process used for the preparation of the device, the improvement in the device functional layer, its overall wearability, and the improvement of the basic photoelectric performance have led to many research achievements at this stage, which were discussed in detail in a previous section. In this section, the potential representative application scenarios of PPG signals are summarized and discussed from the perspective of practical applications.
When the degree of hemoglobin oxygenation changes, the absorption capacity of light will show some differences. The blood flow is also carried out with the vasoconstriction caused by the beating of the pulse, so the basic waveform of the obtained PPG signal is a curve with the same pulse frequency). Therefore, according to Lambert Beer's law, when the light source wavelength of the PPG sensor is in the wave band that can be absorbed by the blood, the pulse rate signal can be obtained easily, and the device with a single light source can also realize this process. However, for the acquisition of the blood oxygen saturation signal, the proportion of oxygenated hemoglobin needs to be obtained. Therefore, it is necessary to design a multi wavelength light source to meet the measurement of hemoglobin content with different oxygenated saturation and corresponding signal extraction. Compared with pulse rate monitoring, the realization of this application scenario also needs to design the corresponding processing circuit to calculate the rear signal. Fig. 10a demonstrates the working principle of PPG sensor, including the extraction of PPG signal and the back-end processing of the signal to meet different application scenarios. Fig. 10b shows the response curve of the HbO2 and HbO of the blood cell to variable light wavelength, the basic situation of hemoglobin's absorption and reflection of light during the PPG sensor working is discussed as well.59,126 Generally, transmission mode and reflection mode are two common process, which will exist at the same time.59–70,124–126Fig. 10c depicts the calculation process of blood oxygen value according the acquired PPG sensor to the light sources with different wavelength.59,126 To extract the bleeding oxygen saturation signal from the PPG signal, it is necessary to test the optical response curves of devices under at least two different wavelengths to meet the calculation requirements. The device intrinsic structure based on the requirements of various application scenarios were summarized in Table 2. According to the development trend of wearable devices in the medical field, PPG sensors have carried out relevant research work in terms of biocompatibility and implantability.
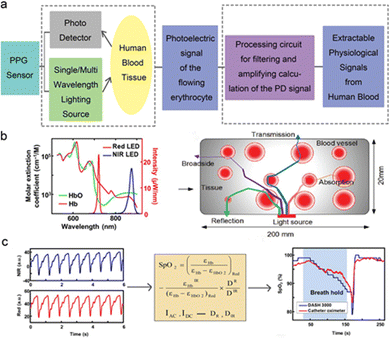 |
| Fig. 10 The working mechanism of the PPG sensors and corresponding signal acquisition method. (a) The process of the extraction and acquisition of PPG sensors. (b) Absorption of hemoglobin with different oxygen content to different wavelengths. (c) Process of converting PPG signal into blood oxygen saturation. Reproduced with permission.59 Copyright 2017, John Wiley and Sons. Reproduced with permission.126 Copyright 2021, American Association for the Advancement of Science. | |
Table 2 Main intrinsic functions and relevant applications of flexible PPG sensors
Structural type |
Structure combination |
Application scenarios |
Relevant functions |
Ref. |
Patch Type |
PPG sensoring system with single wavelength lighting source |
In vitro, noninvasive test, skin surface |
Real time monitoring of the pulse frequency of the human body |
35 and 50
|
54 and 77
|
66, 122 and 129 |
PPG sensoring system based on multi wavelength lighting sources |
In vitro, noninvasive test, skin surface |
Real time monitoring of pulse, oxygen saturation, blood pressure, etc. |
40, 43–45 and 59–61 |
75, 76, 99 and 100 |
48–51 and 130
|
Patch type (contactless reflective-type) |
PPG sensoring system based on double wavelengths LED arrays |
In vitro, noninvasive test, skin surface and non contact real-time monitoring |
Real time monitoring of pulse blood oxygen and non-contact monitoring of wound healing in reflex mode |
42
|
Probe type |
PPG sensoring system based on multi wavelength lighting source with wireless charging and transmission module |
Implanted into the organism, sutured in the femoral artery or brain ventricle, to monitor the corresponding physiological signal. |
It can realize the real-time monitoring of femoral artery blood flow and local oxygen saturation in the deep ventricle |
37 and 124–127
|
Catheter type |
Multi wavelength LED, silicon micro IPD encapsulated in biocompatible silica gel catheter |
Tested in vivo and attached to the surface of the heart |
Real time monitoring of local oxygen saturation and heart beat frequency of organs in vivo |
80 and 128
|
3.1 Noninvasive tests in vitro
The application scenarios of in vitro testing of flexible PPG stress sensors differ according to the device structure and sampling method. When the source is absorbed and reflected by human tissue, the flow of blood cells is accompanied by a pulse beat. Thus the pulse rate can be obtained from the time interval between the peak and peak of the test curve, Fig. 11 demonstrates the application of a flexible PPG sensor in a pulse rate test.54,57 The two devices can well monitor the pulse rate of the human body in different motion states. The noninvasive photoelectric test method is a reliable and stable means to characterize the human heart rate in addition to tactile sensors. In terms of data processing, it is relatively simple to obtain the relevant signals. PPG sensors have different structures and can analyze more information when processing data. Human blood vessels contain a variety of substances, among which only the flow of blood cells is accompanied by the beat pulse. Other substances are in a static state, but the absorption capacities of blood with different oxygen contents to light sources at different wavelengths are different.
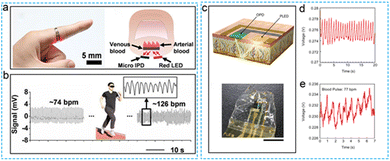 |
| Fig. 11 Application scenario of flexible PPG sensor applied to in vitro noninvasive testing. (a) The flexible devices applied to human pulse rate monitoring. (b) Real time monitoring of the pulse signal. Reproduced with permission.54 Copyright 2017, American Chemical Society. (c) Flexible PPG detector based on PLEDs ingle light source (d) Output voltage characteristics of the OPD. (e) Response of device to pulse signal. Reproduced with permission.122 Copyright 2021, Springer Nature. | |
The measurement of blood oxygen saturation of human body needs to know the proportion of oxygenated hemoglobin in the whole, which will require polychromatic light sources with at least two wavelengths that can be absorbed to realize the measurement and calibration of the flow rate and oxygenation capacity of corresponding blood tissues. As shown in Fig. 12, the multi-wavelength sensing system realizes the detection of human blood oxygen, blood pressure, pulse, and other multi-signal, real-time monitoring tasks.59–61 For the research achievements that have been reported, the multi wavelengths PPG sensor can realize the monitoring of the pulse and blood oxygen saturation in a single point area. To broaden the application field of devices and obtain more accurate test results, A C. Arias proposed a printed reflection oxygen array that can obtain the oxygen saturation in the two-dimensional plane region. The multiple signals detected by the dual wavelength sensor array can achieve more accurate correction and on this basis, the researchers look forward to the future application prospect of the device for skin wound repair, skin graft survival rate monitoring and other aspects. By testing the oxygen saturation in the two-dimensional plane, the recovery of patients can be more accurately judged.42 Additionally, the stability and accuracy of the device are sufficient to apply the device to the detection of patient signs in the intensive care unit. It is expected to become a promising choice in the field of intelligent, personalized medicine.
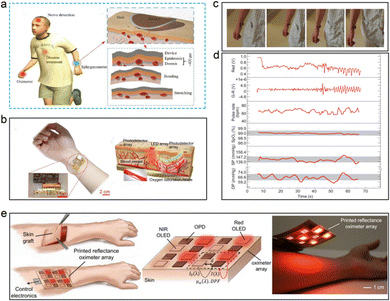 |
| Fig. 12 The flexible devices applied to the blood oxygen and blood pressure test of human body. (a) Schematic diagram of in vitro application scenario of flexible PPG sensor. Reproduced with permission.59 Copyright 2017, John Wiley and Sons. (b) The physical schematic and the working principle of in vitro application. (c) The physical schematic of the human in motion. (d) The corresponding physiological parameters of human body obtained from the test. Reproduced with permission.61 Copyright 2020, Oxford University Press. (e) The schematic diagrams of the printed reflection oxygen array and corresponding application scenario outlook. Reproduced with permission.42 Copyright 2018, PNAS. | |
3.2
In vivo PPG signal of implantable device
The application of flexible PPG sensors for noninvasive monitoring of human physiological signals in vitro has achieved phased results. In this test method, the pain of piercing human tissue and the sharing of wound infection caused by external stimulation are reduced. In terms of real-time supervision of human health, it is an application with mature technology and optimistic prospects.13,80,124–128 In some special cases, there are many organs and fat in positions deep inside the organism. Owing to the limited penetration of the light source, it is difficult to monitor the signal of specific parts in the body in real time; this leads to inaccurate test results.37,111 Based on the above reasons, some technical requirements have been proposed for the biocompatibility and implantability of the device. In the research progress achieved to date, researchers have conducted experiments wherein the device has been implanted into the abdominal cavity, hearts and brains of experimental mice, whereby the device can work stably. Accordingly, they obtained a stable monitoring signal without affecting the biological rhythm of mice to provide a reference for operations in the field of human treatment progress monitoring. In 2015, Yun. proposed an optical fiber oxygenation sensor based on hydrogel material to test the change of blood oxygen content in the rat abdominal cavity. For the application scenario of extracting photoelectric signals in organism, solving the attenuation of light after entering the body is the key factor to determine the accuracy and stability of signal extraction. As shown in Fig. 13a and b, the laser source was coupled into the flexible core-clad structure hydrogel fiber and introduced into the abdominal cavity of the rate. In this work, the monitoring results of the oxygenation in the abdominal cavity of the mice can be obtained, which is due to the low attenuation rate of the light source. These efforts have laid the foundation for postoperative oxygen content monitoring. This section presents the research outcomes pertaining to two implantable flexible PPG sensors. Fig. 13(c and d) presents the experimental scene in which the device is implanted into mice and attached to the heart surface, and the wireless charging system is used to supply power to the devices.126 The blood oxygen sensors were encapsulated using transparent biocompatible silicone materials. The red light, near-infrared dual-wavelength probe, and light baffle were integrated to form a small sensing component based on the catheter's structure. The implantable blood oxygen signal sensor that can be attached to the surface of the mouse heart is composed of a flexible printed circuit board (PCB), an elastic substrate, and a back-end processing circuit. After implantation, the dual-wavelength probe still has stable performance, and can effectively output pulse, blood oxygen and other signals, thus providing a potential choice for real-time monitoring of vital signs during thoracotomy in the future. Fig. 13(f–h) shows the representative research results of another implantable blood oxygen signal sensor.128 The device adopts μ-LED based on red and green wavelengths and a micron-sized probe integrated with μ-IPD, which is designed as a structural model of a flexible microprobe encapsulated by parylene material to improve the shape adaptability and biocompatibility of the device after implantation in vivo. As demonstrated in the figure, the device was implanted into the femoral artery area and the surface of the brain of mice. This work characterized the oxygen content of relevant organs and tissues and the basic information of ventricular oxygen content can be extracted during the implantation experiment, which is capable of proving the stability of the device internal organism environment. This sensing system also provides a new option for monitoring the recovery of patients after human surgery and craniotomy in the future.
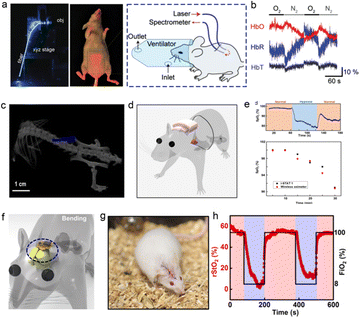 |
| Fig. 13 Application scenarios and signal extraction of implantable PPG signal photoelectric sensor. (a) Physical image of implanting optical fiber conductive PPG signal sensor based on hydrogel material into mouse abdomen. (b) The oxygen content data in mice measured by the device. Reproduced with permission.127 Copyright 2015, Wiley-VCH Verlag. (c and d) Schematically diagram and image of device attached to the surface of mouse heart characterized by CT equipment. (e) Signal monitoring application scenario and Acquisition of corresponding physiological signal data. Reproduced with permission.126 Copyright 2021, American Association for the Advancement of Science. (f and g) Schematic diagram and physical diagram of the device implanted inside the rat body (near femoral artery). (h) Real time monitoring of the rStO2 of the experimental rat and related organs.128 Copyright 2019, American Association for the Advancement of Science. | |
4. Challenges and future outlook
Considering the wearability, real-time monitoring, and stability of human health monitoring, the research on flexible, blood oxygen PD based on the PPG principle has achieved phased results gradually realized good shape adaptability and performance stability. Self-supplied electrical parts and implantable biocompatible devices have also been explored. In this review, representative details of the current state-of-the art flexible PPG sensor are presented. Device-based sensors are mainly used in biomedicine. In future work, it is expected to realize the following points to improve the application potential of devices. Firstly, the combination of self-healing materials and flexible blood oxygen photoelectric probes is expected to improve the mechanical stability and bionics ability of the device. The self-healing ability is an important feature of the biological skin tissue. If the two are combined, the service life of the device can be improved and the bionics of the device can be realized. Secondly, at this stage, the implantable research of the device in animals has been realized, and it is expected to detect the blood oxygen saturation on the surface of the symptomatic viscera inside the human body in the future. However, the process of implanting and removing the device is an invasive process. If the device is degradable in vivo, one invasive operation will be reduced. Therefore, in the next research work, it is expected to realize the biodegradability of the device. It can greatly improve the real-time continuous monitoring of the healing state of organs or wounds after future surgery. Thirdly, as the illustrated results, the vast majority of PPG signal sensors are based on the single point monitoring mode, and the obtained signals can analyze the pulse and the blood oxygen value of the measured position. However, the research on wearable sensors for accurate monitoring of blood oxygen saturation in two-dimensional plane should be paid more attention in future research. The reflectance PPG sensors can accurately determine the wound recovery of patients' skin surface, which is of great significance in the clinical treatment of burn department and general surgery. Ultimately, as an important factor that can measure human health status, ppg signal sensor can be integrated with multifunctional signal senses to achieve more effective and highly sensitive comprehensive health medical equipment. Moreover, being combined with machine learning and other back-end data processing technologies, the intelligent multi-function oximeter Is expected to become valuable and innovative in the field of intelligent medicine.
Author contributions
Weitong Wu: conceptualization, writing original draft. Lili Wang: conceptualization, editing, reviewing. Guozhen Shen: conceptualization, editing, reviewing.
Conflicts of interest
The authors declare that they have no known competing financial interests or personal relationships that could have appeared to influence the work reported in this paper.
Acknowledgements
The authors sincerely acknowledge financial support from the National Natural Science Foundation of China (NSFC Grant No. 62174152, 61888102), and the Science and Technology Development Plan of Jilin Province (20210101168JC).
Notes and references
- Y. M. Zhang, J.-M. Cao, Z. Y. Yuan, H. Xu, D. D. Li, Y. L. Li, W. Han and L. L. Wang, Small, 2022, 18, 2202313 CrossRef CAS PubMed.
- D. Y. Wang, L. L. Wang and G. Z. Shen, J. Semicond., 2020, 41, 041605 CrossRef CAS.
- K. Dong, X. Peng and Z. L. Wang, Adv. Mater., 2020, 32, 1902549 CrossRef CAS.
- Y. Wang and J. Huang, J. Semicond., 2020, 41, 040401 CrossRef.
- S. F. Zhao, W. H. Ran, L. L. Wang and G. Z. Shen, J. Semicond., 2022, 43, 082601 CrossRef.
- D. J. Duncker and R. J. Bache, Phys. Rev., 2008, 88, 1009 CAS.
- J. Allen, Phys. Meas, 2007, 28, 1 CrossRef PubMed.
- D. H. Kim, N. S. Lu, R. Ma, Y. S. Kim, R. H. Kim, S. D. Wang, J. Wu, S. M. Won, H. Tao, A. Islam, K. J. Yu, T. Kim, R. Chowdhury, M. Ying, L. Z. Xu, M. Li, H. J. Chung, H. H. Keum, M. McCormick, P. Liu, Y. W. Zhang, F. G. Omenetto, Y. G. Huang, T. Coleman and J. A. Rogers, Science, 2011, 333, 838–843 CrossRef CAS PubMed.
- J. Heikenfeld, A. Jajack, J. A. Rogers, P. Gutruf, L. Tian, T. Pan, R. Li, M. Khine, J. Kim, J. Wang and J. Kim, Lab Chip, 2018, 18, 217 RSC.
- J. Choi, Y. G. Xue, W. Xia, T. R. Ray, J. T. Reeder, A. J. Bandodkar, D. S. Kang, S. Xu, Y. G. Huang and J. A. Rogers, Lab Chip, 2017, 17, 2572 RSC.
- T. Hamaoka, K. K. McCully, V. Quaresima, K. Yamamoto and B. Chance, J. Bio. Opt., 2007, 12, 062105 CrossRef PubMed.
- L. L. Wang, K. Jiang and G. Z. Shen, Adv. Mater. Technol., 2021, 6, 2100107 CrossRef.
- J. K. Song, M. S. Kim, S. W. Yoo, J. H. Koo and D. H. Kim, Nano Res., 2014, 14, 2919 CrossRef.
- A. Nathan, A. Ahnood, M. T. Cole, S. S. Lee, Y. Suzuki, P. Hiralal, F. Bonaccorso, T. Hasan, L. G. Gancedo, A. Dyadyusha, S. Haque, P. Andrew, S. Hofmann, J. Moultrie, D. P. Chu, A. J. Flewitt, A. C. Ferrari, M. J. Kelly, J. Robertson, G. Amaratunga and W. Milne, IEEE, 2012, 100, 1486 Search PubMed.
- S. K. Hwang, C. H. Lee, H. Y. Cheng, J. W. Jeong, S. K. Kang, J. H. Kim, J. H. Shin, J. Yang, Z. J. Liu, G. A. Ameer, Y. G. Huang and J. A. Rogers, Nano Lett., 2015, 15, 2801 CrossRef CAS PubMed.
- Z. Lou and G. Z. Shen, Small Struct., 2021, 2, 2000152 CrossRef CAS.
- A. Campana, T. Cramer, D. T. Simon, M. Berggren and F. Biscarini, Adv. Mater., 2014, 26, 3874 CrossRef CAS PubMed.
- L. L. Wang, K. Jiang and G. Z. Shen, Appl. Phys. Lett., 2021, 119, 150501 CrossRef CAS.
- Y. H. Chen, S. Y. Lu, S. S. Zhang, Y. Li, Z. Qu, Y. Chen, B. W. Lu, X. Y. Wang and X. Feng, Sci. Adv., 2017, 3, e1701629 CrossRef PubMed.
- S. Y. Heo, J. H. Kim, P. Gutruf, A. Banks, P. Wei, R. Pielak, G. Balooch, Y. Z. Shi, H. Araki, D. Rollo, C. Gaede, M. Patel, J. W. Kwak, A. E. Peña-Alcántara, K. T. Lee, Y. J. Yun, J. K. Robinson, S. Xu and J. A. Rogers, Sci. Transl. Med., 2018, 10, eaau1643 CrossRef CAS PubMed.
- V. K. Singh, A. Anis, S. A. Zahrani, D. K. Pradhan and K. Pal, Int. J. Electrochem. Sci., 2014, 9, 5640 Search PubMed.
- E. M. Song, J. H. Li, S. M. Won, W. B. Bai and J. A. Rogers, Nat. Mater., 2020, 19, 590 CrossRef CAS PubMed.
- Y. H. Seok and J. J. Kwok, Nat. Biomed. Eng., 2017, 1, 1 CrossRef.
- S. J. Kwon, Y. T. Kwon, Y. S. Kim, H. R. Lim, M. Mahmoo and W. H. Yeo, Biosens. Bioelectron., 2020, 151, 111981 CrossRef CAS PubMed.
- T. Tamura, Y. Maeda, M. Sekine and M. Yoshida, Electronics, 2014, 3, 282 CrossRef.
- K. Takei, W. Honda, S. Harada, T. Arie and S. Akita, Adv. Healthcare Mater., 2015, 4, 487 CrossRef CAS PubMed.
- M. K. Choi, J. W. Yang, K. H. Kang, D. C. Kim, C. S. Choi, C. Park, S. J. Kim, S. I. Chae, T. H. Kim, J. H. Kim, T. H. Hyeon and D. H. Kim, Nat. Commun., 2015, 6, 1 Search PubMed.
- W. H. Ran, Z. H. Ren, P. Wang, Y. X. Yan, K. Zhao, L. L. Li, Z. X. Li, L. L. Wang, J. H. Yang, Z. M. Wei, Z. Lou and G. Z. Shen, Nat. Commun., 2021, 12, 1 CrossRef.
- R. R. Bao, C. F. Wang, L. Dong, C. Y. Shen, K. Zhao and C. F. Pan, Nanoscale, 2015, 8, 8078 RSC.
- B. W. Zhong, K. Jiang, L. L. Wang and G. Z. Shen, Adv. Sci., 2022, 9, 2103257 CrossRef CAS PubMed.
- Y. G. Wang, Y. Y. Huang, Y. Wang, P. J. Lyu and M. R. Hamblin, Biochim. Biophys. Acta, 2017, 1862, 441 CrossRef.
- J. A. Rogers, MRS Bull., 2014, 39, 549 CrossRef.
- X. Y. Fu, L. L. Wang, L. J. Zhao, Z. Y. Yuan, Y. P. Zhang, D. Y. Wang, D. P. Wang, J. Z. Li, D. D. Li, V. Shulga, G. Z. Shen and W. Han, Adv. Funct. Mater., 2021, 31, 2010533 CrossRef CAS.
- J. M. May, J. P. Phillips, T. Fitchat, S. Ramaswamy, S. Snidvongs and P. A. Kyriacou, Biosensors, 2019, 9, 119 CrossRef PubMed.
- S. C. Zhang, C. M. Qu, Y. Xiao, H. Y. Liu, G. F. Song and Y. Xu, Nanoscale, 2022, 14, 4244 RSC.
- Y. Cui, Y. H. Li, Y. F. Xing, T. Z. Yang and J. Z. Song, Int. J Therm. Sci., 2018, 127, 321 CrossRef CAS.
- M. Humara, S. Kwoka, M. H. Choi, A. K. Yetisen, S. Y. Cho and S. H. Yun, Nano Photo., 2017, 6, 414 Search PubMed.
- M. Elgendi, Curr. Cardiol. Rev., 2012, 8, 14 CrossRef PubMed.
- S. Majumder, T. Mondal and M. J. Deen, Sensors, 2017, 17, 130 CrossRef.
- H. K. Ballaji, R. Correia, S. Korposh, B. R. Hayes-Gill, F. U. Hernandez, B. Salisbury and S. P. Morgan, Sensors, 2020, 20, 6568 CrossRef CAS PubMed.
- O. Polat, G. Mercier, I. Nikitskiy, E. Puma, T. Galan, S. Gupta, M. Montagut, J. J. Piqueras, M. Bouwens, T. Durduran, G. Konstantatos, S. J. Goossens and F. Koppens, Sci. Adv., 2019, 5, eaaw7846 CrossRef PubMed.
- Y. Khan, D. G. Han, A. Pierre, J. Ting, X. C. Wang, C. M. Lochnera, G. Bovob, N. Y. Grossb, C. Newsome, R. Wilson and A. C. Arias, Proc. Natl. Acad. Sci. U. S. A., 2018, 115, E11015 CAS.
- S. H. Kang, V. P. Rachim, J. H. Baek, S. Y. Lee and S. M. Park, IEEE Access, 2020, 30, 17218 Search PubMed.
- C. M. Lochner, Y. Khan, A. Pierre and A. C. Arias, Nat. Commun., 2014, 5, 1 Search PubMed.
- J. H. Kim, B. W. Jo, J. H. Jo, Y. S. Lee and D. K. Kim, Sustainability, 2021, 13, 1102 CrossRef.
- F. Elsamnah, A. Bilgaiyan, M. Affifiq, C. H. Shim, H. Ishidai and R. Hattori, Biosensors, 2019, 9, 48 CrossRef CAS PubMed.
- J. H. Lee, K. Matsumura, K. Yamakoshi, P. Rolfe, S. Tanaka and T. Yamakoshi, IEEE, 2013, 1724 CAS.
- H. W. Lee, E. H. Kim, Y. S. Lee, H. Y. Kim, J. H. Lee, M. Kim, H. J. Yoo and S. H. Yoo, Sci. Adv., 2018, 4, eaas9530 CrossRef CAS PubMed.
- D. H. Kim, Y. S. Lee, W. K. Park, J. S. Yoo, C. Shim, Y. J. Hong, B. K. Kang, D. H. Yoon and W. S. Yang, Sensors, 2020, 20, 2651 CrossRef CAS.
- S. J. Park, K. Fukuda, M. Wang, C. H. Lee, T. Yokota, H. Jin, H. Jinno, H. Kimura, P. Zalar, N. Matsuhisa, S. Umezu, G. C. Bazan and T. Someya, Adv. Mater., 2018, 30, 1802359 CrossRef.
- A. V. Chong, M. Terosiet, A. Histace and O. Romain, Microelectron. J., 2019, 88, 128 CrossRef.
- I. H. Lee, N. K. Park, H. B. Lee, C. J. Hwang, J. H. Kim and S. J. Park, Appl. Sci., 2021, 11, 2313 CrossRef CAS.
- T. Yokota, P. Zalar, M. Kaltenbrunner, H. Jinno, N. Matsuhisa, H. Kitanosako, Y. Tachibana, W. Yukita, M. Koizumi and T. Someya, Sci. Adv., 2016, 2, e1501856 CrossRef.
- J. H. Kim, N. Y. Kim, M. J. Kwon and J. H. Lee, ACS Appl. Mater. Interfaces, 2017, 9, 25700 CrossRef CAS PubMed.
- P. I. Mak, J. Semicond., 2020, 41, 11301 CrossRef.
- J. A. Rogers, T. Someya and Y. G. Huang, Science, 2010, 327, 1603 CrossRef CAS.
- H. J. Lee, T. K. Choi, Y. B. Lee, H. R. Cho, R. Ghaffari, L. Wang, H. J. Cho, T. D. Chung, N. S. Lu, T. H. Hyeon, S. H. Choi and D. H. Kim, Nat. Nanotechnol., 2016, 11, 566 CrossRef CAS PubMed.
- J. S. Kim, S. J. Park, T. Nguyen, M. Chu, J. D. Pegan and M. Khine, Appl. Phys. Lett., 2016, 108, 061901 CrossRef PubMed.
- H. C. Li, Y. Xu, X. M. Li, Y. Chen, Y. Jiang, C. X. Zhang, B. W. Lu, J. Wang, Y. J. Ma, Y. H. Chen, Y. Huang, M. Q. Ding, H. H. Su, G. F. Song, Y. Luo and X. Feng, Adv. Healthcare Mater., 2017, 6, 1601013 CrossRef PubMed.
- J. H. Kim, G. A. Salvatore, H. Araki, A. M. Chiarelli, Z. Q. Xie, A. Banks, X. Sheng, Y. H. Liu, J. W. Lee, K. I. Jang, S. Y. Heo, K. Y. Cho, H. Y. Luo, B. Zimmerman, J. H. Kim, L. Q. Yan, X. Feng, S. Xu, M. Fabiani, G. Gratton, Y. G. Huang, U. Y. Paik and J. A. Rogers, Sci. Adv., 2016, 2, e1600418 CrossRef PubMed.
- H. C. Li, Y. J. Ma, Z. W. Liang, Z. H. Wang, Y. Cao, Y. Xu, H. Zhou, B. W. Lu, Y. Chen, Z. Y. Han, S. S. Cai and X. Feng, Natl. Sci. Rev., 2020, 7, 849 CrossRef PubMed.
- M. Wang, H. X. Sun, F. R. Cao, W. Tian and L. Li, Adv. Mater., 2021, 33, 2100625 CrossRef CAS PubMed.
- M. Hossain, G. S. Kumar, S. N. B. Prabhava, E. D. Sheerin, D. McCloskey, S. Acharya, K. D. M. Rao and J. J. Boland, ACS Nano, 2018, 12, 4727 CrossRef CAS PubMed.
- C. H. An, F. M. Nie, R. J. Zhang, X. L. Ma, D. H. Wu, Y. Sun, X. D. Hu, D. Sun, L. Pan and J. Liu, Adv. Funct. Mater., 2021, 31, 2100136 CrossRef CAS.
- A. Bilgaiyan, F. Elsamnah, H. Ishidai, C. H. Shim, M. A. B. Misran, C. Adachi, R. Hattori and A. C. S. Appl, Electron. Mater., 2020, 2, 1280 CAS.
- H. X. Jun, J. Liu, J. Zhang, G. D. Zhou, N. Q. Luo and N. Zhao, Adv. Mater., 2017, 29, 1700975 CrossRef PubMed.
- S. J. Wei, Reconfigurable computing: a promising microchip architecture for artificial intelligence, J. Semicond., 2020, 41, 020301 CrossRef.
- D. P. Wang, L. L. Wang and G. Z. Shen, J. Semicond., 2020, 41, 041605 CrossRef CAS.
- G. H. Kim, J. Y. Oh, Y. S. Yang, L. M. Do and K. S. Suh, Thin. Soli. Film., 2004, 467, 1 CrossRef CAS.
- E. G. Jeong, S. Kwon, J. H. Han, H. G. Im, B. S. Bae and K. C. Choi, Nanoscale, 2013, 9, 6370 RSC.
- J. S. Yoon, S. J. Jo, I. S. Chun, I. H. Jung, H. S. Kim, M. Meitl, E. Menard, X. L. Li, J. J. Coleman, U. Y. Paik and J. A. Rogers, Nature, 2010, 465, 329 CrossRef CAS PubMed.
- S. I. Park, Y. J. Xiong, R. H. Kim, P. Elvikis, M. Meitl, D. H. Kim, J. Wu, J. S. Yoon, C. J. Yu, Z. J. Liu, Y. G. Huang, K. C. Hwang, P. Ferreira, X. L. Li, K. Choquette and J. A. Rogers, Science, 2009, 325, 977 CrossRef CAS.
- D. G. Han, Y. Khan, J. Ting, S. M. King, N. Yaacobi-Gross, M. J. Humphries, C. J. Newsome and A. C. Arias, Adv. Mater., 2017, 29, 1606206 CrossRef.
- I. Nikitskiy, S. Goossens, D. Kufer, T. Lasanta, G. Navickaite, F. H. L. Koppens and G. Konstantatos, Nat. Commun., 2016, 7, 1 Search PubMed.
- S. B. Zhang, T. Zhang, Z. G. Liu, J. Z. Wang, J. Xu, K. J. Chen and L. W. Yu, Adv. Funct. Mater., 2021, 32, 2107040 CrossRef.
- Z. J. Zhao, B. Q. Liu, C. L. Xie, Y. Ma, J. Wang, M. Liu, K. X. Yang, Y. H. Xu, J. Zhang, W. W. Li, L. Shen and F. J. Zhang, Sci. China: Chem., 2021, 64, 1302 CrossRef CAS.
- C. F. Hernandez, W. F. Chou, T. M. Khan, L. Diniz, J. Lukens, F. A. Larrain, V. A. Rodriguez-Toro and B. Kippelen, Science, 2020, 370, 698 CrossRef.
- K. Dong and Z. L. Wang, J. Semicond., 2021, 42, 101601 CrossRef CAS.
- H. O. Yang, J. J. Tian, G. L. Sun, Y. Zou, Z. Liu, H. Li, L. M. Zhao, B. J. Shi, Y. B. Fan, Y. F. Fan, Z. L. Wang and Z. Li, Adv. Mater., 2017, 29, 1703456 CrossRef PubMed.
- A. J. J. M. Breemen, R. Ollearo, S. Shanmugam, B. Peeters, L. C. J. M. Peter, R. L. Ketterji, I. Katsouras, H. B. Akkerman, C. H. Frijters, F. D. Giacomo, S. Veenstra, R. Andriessen, R. A. J. Janssen, E. A. Meulenkamp and G. H. Gelinck, Nat. Electron., 2021, 4, 818 CrossRef.
- T. H. Kim, C. S. Lee, S. W. Kim, J. H. Hur, S. M. Lee, K. W. Shin, Y. Z. Yoon, M. K. Choi, J. W. Yang, D. H. Kim, T. H. Hyeon, S. J. Park and S. W. Hwang, ACS Nano, 2017, 11, 5992 CrossRef CAS.
- J. Y. Zhao, L. W. Lo, H. C. Wan, P. S. Mao, Z. B. Yu and C. Wang, Adv. Mater., 2021, 33, 2102095 CrossRef CAS.
- R. H. Kim, D. H. Kim, J. L. Xiao, B. H. Kim, S. I. Park, B. Panilaitis, R. Ghaffari, J. M. Yao, M. Li, Z. J. Liu, V. Malyarchuk, D. G. Kim, A. P. Le, R. G. Nuzzo, D. L. Kaplan, F. G. Omenetto, Y. G. Huang, Z. Kang and J. A. Rogers, Nat. Mater., 2010, 9, 929 CrossRef CAS PubMed.
- Y. M. Zhang, Z. J. Wang, J. H. Feng, S. Q. Ming, F. R. Qu, Y. Xia, M. He, Z. M. Hu and J. Wang, J. Semicond., 2022, 43, 102002 CrossRef.
- L. L. Li, S. F. Zhao, W. H. Ran, Z. X. Li, Y. X. Yan, B. W. Zhong, Z. Lou, L. L. Wang and G. Z. Shen, Nat. Commun., 2022, 13, 5975 CrossRef CAS PubMed.
- A. L. Olatomiwa, T. Adam, S. C. B. Gopinath, S. Y. Kolawole, O. H. Olayinka and U. Hashim, J. Semicond., 2022, 43, 061101 CrossRef.
- S. F. Zhao, W. H. Ran, Z. Lou, L. L. Li, S. Poddar, L. L. Wang, Z. Y. Fan and G. Z. Shen, Natl. Sci. Rev., 2022, nwac158 CrossRef PubMed.
- Q. Zhou, C. T. Zuo, Z. L. Zhang, P. Gao and L. M. Ding, J. Semicond., 2022, 43, 010202 CrossRef CAS.
- C. Li, P. Li, S. Yang and C. Y. Zhi, J. Semicond., 2021, 42, 101603 CrossRef CAS.
- B. M. Lee, J. Y. Oh, H. Cho, C. W. Joo, H. S. Yoon, S. J. Jeong, E. H. Oh, J. H. Byun, H. Kim, S. H. Lee, J. S. Seo, C. W. Park, S. K. Choi, N. M. Park, S. Y. Kang, C. S. Hwang, S. D. Ahn, J. I. Lee and Y. T. Hong, Nat. Commun., 2020, 11, 1 CrossRef.
- X. J. Jin, L. L. Li, S. F. Zhao, X. H. Li, K. Jiang, L. L. Wang and G. Z. Shen, ACS Nano, 2021, 15, 18385 CrossRef CAS PubMed.
- W. X. Yang, X. L. Wang, H. Q. Li, J. Wu and Y. Q. Hu, Nano Energy, 2018, 51, 241 CrossRef CAS.
- K. Q. Xia, Z. Y. Zhu, H. Z. Zhang, C. L. Du, Z. W. Xu and R. J. Wang, Nano Energy, 2018, 50, 571 CrossRef CAS.
- H. M. Chen, Y. Xu, J. S. Zhang, W. T. Wu and G. F. Song, Nano Energy, 2019, 58, 304 CrossRef CAS.
- Y. Shao, C. P. Feng, B. W. Deng, B. Yin and M. B. Yang, Nano Energy, 2019, 62, 620 CrossRef CAS.
- K. Q. Xia, H. Z. Zhang, Z. Y. Zhu and Z. W. Xu, Sens. Actuators, A, 2018, 272, 28 CrossRef CAS.
- Y. P. Jeon, J. H. Park and T. W. Kim, Appl. Surf. Sci., 2019, 466, 210 CrossRef CAS.
- Z. M. Tian, G. C. Shao, Q. Zhang, Y. N. Geng and X. Chen, Micromachines, 2019, 10, 656 CrossRef PubMed.
- H. M. Chen, Y. Xu, J. S. Zhang, W. T. Wu and G. F. Song, Nanomaterials, 2019, 9, 778 CrossRef PubMed.
- H. M. Chen, W. Yang, M. Q. Wu, W. J. Li, Y. X. Zhou, L. F. Lv, H. L. Yu, H. Z. Ke, R. P. Liu, Y. Xu, J. Wang and Z. Li, Nano Res., 2022, 15, 2465 CrossRef CAS.
- A. Ullah, A. K. Kasi, J. K. Kasi and M. U. M. Bokhari, Curr. Appl. Phys., 2020, 20, 137 CrossRef.
- H. M. Chen, L. Bai, T. Li, C. Zhao, J. S. Zhang, N. Zhang, G. F. Song, Q. Q. Gan and Y. Xu, Nano Energy, 2018, 46, 73 CrossRef CAS.
- Z. Wen, Y. Q. Yang, N. Sun, G. F. Li, Y. N. Liu, C. Chen, J. H. Shi, L. J. Xie, H. X. Jiang, D. Q. Bao, Q. Q. Zhuo and X. H. Sun, Adv. Funct. Mater., 2018, 28, 1803684 CrossRef.
- J. B. Yu, X. J. Hou, J. He, M. Cui, C. Wang, W. P. Geng, J. L. Mu, B. Han and X. J. Chou, Nano Energy, 2020, 69, 104437 CrossRef CAS.
- Y. Wei, H. M. Chen, M. Q. Wu, Z. Y. Sun, M. Gao, W. J. Li, C. Y. Li, H. L. Yu, Y. Xu and J. Wang, Adv. Mater. Interfaces, 2022, 9, 2102124 CrossRef.
- Z. L. Li, M. M. Zhu, Q. Qiu, J. Y. Yu and B. Ding, Nano Energy, 2018, 53, 726 CrossRef CAS.
- Z. Li, G. Zhu, R. S. Yang, A. C. Wang and Z. L. Wang, Adv. Mater., 2010, 22, 2534 CrossRef CAS PubMed.
- D. S. Choi, S. M. Yang, C. H. Lee, W. J. Kim, J. H. Kim and J. P. Hong, ACS Appl. Mater. Interfaces, 2018, 10, 33221 CrossRef CAS PubMed.
- L. L. Li, D. P. Wang, D. Zhang, W. H. Ran, Y. X. Yan, Z. X. Li, L. L. Wang and G. Z. Shen, Adv. Funct. Mater., 2021, 31, 2104782 CrossRef CAS.
- G. H. Lee, H. Moon, H. M. Kim, G. H. Lee, W. S. Kwon, S. H. Yoo, D. Myung, S. H. Yun, Z. N. Bao and S. K. Hahn, Nature, 2020, 5, 149 Search PubMed.
- D. H. Kim, S. D. Wang, H. H. Keum, R. Ghaffari, Y. S. Kim, H. Tao, B. Panilaitis, M. Li, Z. Kang, F. Omenetto, Y. G. Huang and J. A. Rogers, Small, 2012, 21, 3263 CrossRef.
- T. Yamanaka, H. Nakanotani, S. Hara, T. Hirohata and C. Adach, Appl. Phys. Express, 2017, 10, 074101 CrossRef.
- Y. S. J. Hong, H. Y. Jeong, K. W. Cho, N. S. Lu and D. H. Kim, Adv. Funct. Mater., 2019, 29, 1808247 CrossRef.
- Z. Zhang, C. Chen, T. R. Fei, H. Xiao, G. J. Xie and X. Chen, J. Semicond., 2020, 41, 102403 CrossRef.
- G. Cohen and P. Chazal, Comput. Biol. Med., 2015, 63, 118 CrossRef PubMed.
- G. C. Shin, A. M. Gomez, R. Al-Hasani, Y. R. Jeong, J. H. Kim, Z. Q. Xie, A. Banks, S. M. Lee, S. Y. Han, C. J. Yoo, J. L. Lee, S. H. Lee, J. Kurniawan, J. Tureb, Z. Z. Guo, J. Y. Yoon, S. Park, S. Y. Bang, Y. H. Nam, M. C. Walicki, V. K. Samineni, A. D. Mickle, K. H. Lee, S. Y. Heo, J. G. McCall, T. S. Pan, L. Wang, X. Feng, T. Kim, J. K. Kim, Y. H. Li, Y. G. Huang, R. W. Gereau IV, J. S. Ha, M. R. Bruchas and J. A. Rogers, Neuron, 2017, 93, 1 CrossRef PubMed.
- T. Kim, J. G. McCall, Y. H. Jung, X. Huang, E. R. Siuda, Y. H. Li, J. Z. Song, Y. M. Song, H. A. Pao, R. H. Kim, C. F. Lu, S. D. Lee, S. Song, G. C. Shin, R. A. Hasani, S. Kim, M. P. Tan, Y. G. Huang, F. G. Omenetto, J. A. Rogers and M. R. Bruchas, Science, 2013, 340, 211 CrossRef CAS PubMed.
- S. F. Wu, Z. Li, J. Zhang, X. Wu, X. Deng, Y. M. Liu, J. K. Zhou, C. Y. Zhi, X. G. Yu, W. C. H. Choy, Z. L. Zhu and A. K. Y. Jen, Adv. Mater., 2021, 33, 2105539 CrossRef CAS PubMed.
- C. D. Ge, X. T. Liu, Z. Q. Yang, H. M. Li, W. W. Niu, X. K. Liu and Q. F. Dong, Angew. Chem., Int. Ed., 2022, 61, e202116602 CAS.
- S. X. Xiong, K. Fukuda, S. Y. Lee, K. Nakano, X. Y. Dong, T. Yokota, K. Tajima, Y. H. Zhou and T. Someya, Adv. Sci., 2022, 9, 2105288 CrossRef CAS PubMed.
- M. Kaltenbrunner, M. S. White, E. D. Głowacki, T. Sekitani, T. Someya, N. S. Sariciftci. and S. Bauer, Nat. Commun., 2012, 3, 1 Search PubMed.
- H. Jinno, T. Yokota, M. Koizumi, W. Yukita, M. Saito, I. Osaka, K. Fukuda and T. Someya, Nat. Commun., 2021, 12, 1 CrossRef PubMed.
- S. J. Park, S. W. Heo, W. R. Lee, D. S. Inoue, Z. Jiang, K. H. Yu, H. Jinno, D. Hashizume, M. Sekino, T. Yokota, K. Fukuda, K. Tajima and T. Someya, Nature, 2018, 561, 516 CrossRef CAS PubMed.
- S. Chen, A. Z. Weitemier, X. Zeng, L. M. He, X. Y. Wang, Y. Q. Tao, J. Y. Huang, Y. Hashimotodani, M. Kano, H. Iwasaki, L. K. Parajuli, S. Okabe, D. B. L. Teh, A. H. All, I. T. Kimura, K. F. Tanaka, X. G. Liu and T. J. McHugh, Science, 2018, 359, 679 CrossRef CAS PubMed.
- S. Park, D. S. Brenner, G. C. Shin, C. D. Morgan, B. A. Copits, H. U. Chung, M. Y. Pullen, K. N. Noh, S. Davidson, S. J. Oh, J. Y. Yoon, K. I. Jang, V. K. Samineni, M. Norman, J. G. Gragales-Reyes, S. K. Vogt, S. S. Sundaram, K. M. Wilson, J. S. Ha, R. X. Xu, T. S. Pan, T. Kim, Y. G. Huang, M. C. Montana, J. P. Golden, M. R. Bruchas, R. W. Gereau and J. A. Rogers, Nat. Biotechnol., 2015, 33, 1280 CrossRef CAS PubMed.
- W. Lu, W. B. Bai, H. Zhang, C. K. Xu, A. M. Chiarelli, A. V. Guardado, Z. Q. Xie, H. X. Shen, K. Nandoliya, H. B. Zhao, K. H. Lee, Y. X. Wu, D. Franklin, R. Avila, S. Xu, A. Rwei, M. D. Han, K. H. Kwon, Y. J. Deng, X. G. Yu, E. B. Thorp, X. Feng, Y. G. Huang, J. Forbess, Z. D. Ge and J. A. Rogers, Sci. Adv., 2021, 7, eabe0579 CrossRef CAS PubMed.
- M. H. Choi, M. Humar, S. H. Kim and S. H. Yun, Adv. Mater., 2015, 27, 4081–4086 CrossRef CAS PubMed.
- H. Zhang, P. Gutruf, K. Meacham, M. Montana, X. Y. Zhao, A. M. Chiarelli, A. Guardado, A. Norris, L. Y. Lu, Q. L. Guo, C. K. Xu, Y. X. Wu, H. B. Zhao, X. Ning, W. B. Bai, I. Kandela, C. Haney, D. Chanda, R. W. Gereau and J. A. Rogers, Sci. Adv., 2019, 5, eaaw0873 CrossRef CAS PubMed.
- S. H. Kang, V. P. Rachim, J. H. Baek, S. Y. Lee and S. M. Park, IEEE Access, 2017, 8, 152105 Search PubMed.
- Y. J. Lee, J. W. Chung, G. H. Lee, H. B. Kang, J. Y. Kim, C. S. Bae, H. J. Yoo, S. J. Jeong, H. Cho, S. G. Kang, J. Y. Jung, D. W. Lee, S. A. Gam, S. G. Hahm, Y. Kuzumoto, S. J. Kim, Z. N. Bao, Y. T. Hong, Y. J. Yun and S. H. Kim, Sci. Adv., 2021, 7, eabg9180 CrossRef CAS PubMed.
|
This journal is © The Royal Society of Chemistry 2023 |
Click here to see how this site uses Cookies. View our privacy policy here.