DOI:
10.1039/D2CP00560C
(Perspective)
Phys. Chem. Chem. Phys., 2022,
24, 11455-11470
Exploiting the upconversion luminescence, Lewis acid catalytic and photothermal properties of lanthanide-based nanomaterials for chemical and polymerization reactions
Received
3rd February 2022
, Accepted 12th April 2022
First published on 13th April 2022
Abstract
Lanthanide-based nanocrystals possess three unique physical properties that make them attractive for facilitating photoreactions, namely photon upconversion luminescence, Lewis acid catalytic activity and photothermal properties. When co-doped with a suitable sensitizer and activator lanthanide ions, rare-earth fluoride nanocrystals upconvert near-infrared light to higher energy photons that can be used to excite photosensitizers that absorb ultraviolet and visible light in photocatalytic and photopolymerization reactions. Surface lanthanide ions on nanocrystals also have the propensity to behave as Lewis acid (LA) catalytic sites. In addition, NIR-light excited lanthanides such as Nd3+ undergo cross-relaxation interaction with neighbouring ground-state ions followed by non-radiative decay to generate heat (i.e., photothermal) which enhances the rate of chemical reactions. In this perspective, we provide a survey of the recent progress in the use of lanthanide-based nanocrystals as upconverting nanolamps, LA catalysts and photothermal nanoheaters in driving synthetic and polymerization reactions, and the challenges that need to be further addressed in order for this vibrant research area to develop and grow.
| Xiangyang Wu received his PhD degree from Nanyang Technological University in 2011 under the supervision of Prof. Edwin K. L. Yeow. He then worked as a research fellow with the same research group. His current research interests include the synthesis and characterization of functional nanomaterials, investigation of the photophysical properties of novel optical materials using both ensemble-averaged and single-molecule fluorescence techniques, and NIR-light activated heterogeneous photocatalysis. |
| Edwin K. L. Yeow is an Associate Professor of Chemistry at the Nanyang Technological University, Singapore. He is the recipient of the Photochemistry Association Lectureship Award for Asian and Oceanian Photochemist 2018. His research interests include understanding the role and application of light in photoredox catalysis, antimicrobial activity and analytical sensing. |
1. Introduction
Lanthanides have received enormous attention because of their unique properties such as having rich electronic energy-levels and displaying near infrared (NIR) light-activated Stokes and anti-Stokes emission.1–12 Lanthanide-doped materials have therefore broadband absorption, downconversion emission from NIR to mid-infrared, and upconversion emission ranging from deep ultraviolet (UV) to visible regions. Lanthanide luminescence arises from f–f electronic transitions within the 4f orbital and is shielded by 5s and 5p orbitals, making lanthanide emission relatively stable.7,11,13,14 In order to improve emission quantum efficiency, a fluoride-based matrix with low phonon energy is often chosen to host lanthanide dopants (e.g., in lanthanide-based rare-earth metal (Re) fluoride ReF3 and alkaline (A) Re fluoride (AReF4) nanocrystals).
The photon upconversion properties of (alkaline) Re fluoride nanocrystals allow them to behave as NIR-activated UV/visible ‘nanolamps’.15–22 The emission upconversion nanocrystals (UCNCs) are capable of facilitating a series of important light-induced reactions such as photoisomerization, photopolymerization, photolysis and photocoupling.7,19–22 The energy of light emitted from UCNCs, covering a wide spectrum range from UV to NIR, can be selectively tuned to excite various photosensitizers. For example, semiconductors excited by upconverted UV photons from UCNCs have been used in water splitting,6,23,24 wastewater treatment,6,25–27 nitrogen photofixation28–30 and photoelectrocatalysis.6,23,31–34
To date, most photocatalytic reactions are driven by photoredox catalysts that absorb UV/visible light (Fig. 1a).35–40 Unfortunately, there are several challenges associated with the utilization of UV/visible light sources: (i) low penetration of UV/visible light in reaction mixtures and opaque reactors, (ii) photodegradation of substrates by direct UV light irradiation, and (iii) competition of the reactants and photocatalysts for the absorption of excitation light; limiting large-scale reactions. NIR light circumvents the above issues since it has good penetration and most reactants do not absorb NIR light.41,42 Direct excitation of NIR light-absorbing photoredox catalysts such as plasmonic nanoparticles (e.g., Au–Pd43), organometallic complexes (e.g., Os(II)-polypyridyl complexes44) and organic dyes (e.g., cyanines45,46) has been applied in organic synthesis.47 Alternatively, the use of molecular photon upconversion materials and lanthanide-based UCNCs as internal light sources has been introduced. For example, upconversion photons from NIR-activated triplet–triplet annihilation involving a palladium(II) octabutoxyphthalocyanine sensitizer and a furanyl diketopyrrolopyrrole annihilator are transferred to photoredox catalysts (e.g., Eosin Y, Rose Bengal) needed to drive a series of reactions including hydrodehalogenation, oxidation, radical cyclization, [2+2] cyclization, vinyl azide sensitization and radical polymerization.42
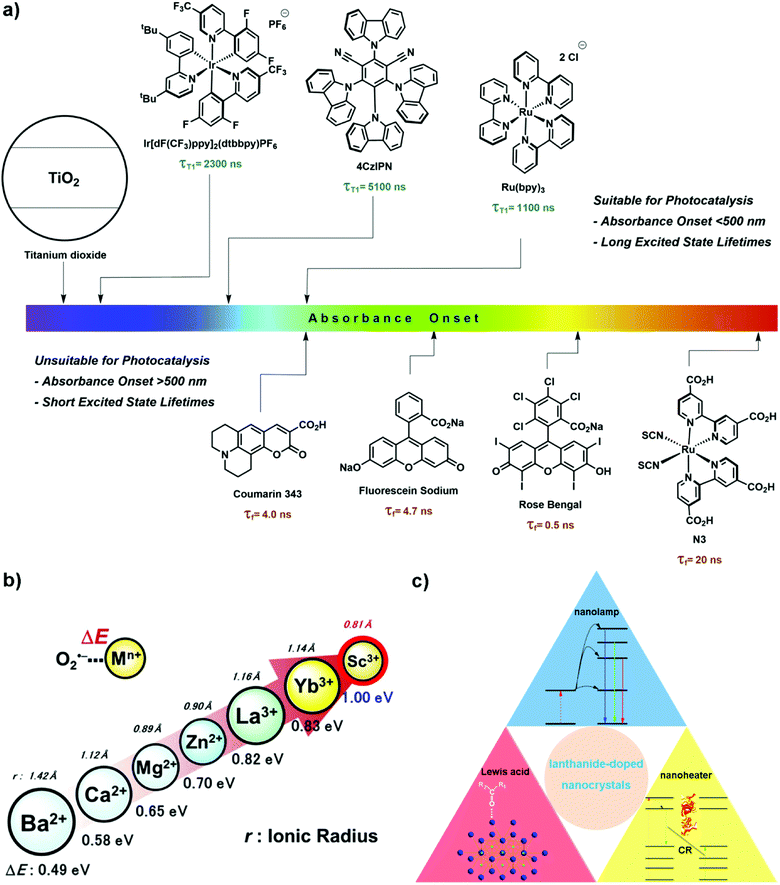 |
| Fig. 1 (a) Absorption window of photosensitizers including dyes and semiconductors.35 Reprinted with permission from ref. 35. Copyright 2021, Elsevier. (b) The relationship between the Lewis acidity (ΔE) and ionic radius of redox-inactive Re3+ ions.51 Details of the measurement of ΔE can be found in ref. 51. Reprinted with permission from ref. 51. Copyright 2017, Wiley-VCH. (c) Scheme of the three functions (i.e., Lewis acid catalyst, upconversion nanolamp, and photothermal nanoheater) of lanthanide-doped nanocrystals. | |
Most Re including lanthanides exist in their 3+ oxidation state, except for those with stable 4f configurations (e.g., Ce4+, Tb4+, Eu2+ and Yb2+).48 The electropositive ions act as a Lewis acid (LA) catalyst by accepting electrons from a reactant and increasing its reactivity (e.g., the nucleophilicity of the carbonyl compound).49 In particular, lanthanides have the propensity to activate double and triple bonds, and coordinate with heteroatoms such as carbonyl oxygen. Compared to other LAs (e.g., Ba2+, Ca2+, Mg2+ and Zn2+), the strongly electropositive Re ions are regarded as hard LAs whose Lewis acidity increases with reduced ionic radius (Fig. 1b).50,51 As such, lanthanide ions have been extensively used as LA catalysts in various organic reactions including aldol reactions, Mannich reactions, cycloaddition reactions, Friedel–Crafts reactions, cyanosilylation and ring opening reactions.49,52,53
Photothermal materials (PTMs), capable of converting light into thermal energy, have found useful applications in solar evaporation and photothermal catalysis.54–56 PTMs including metals (e.g., Au,57,58 Ag,59 and Cu59), semiconductors,60,61 carbon materials62 and polymers63,64 display efficient light-to-heat conversion efficiency via a variety of mechanisms such as plasmonic heating, electron–hole pair relaxation and non-radiative vibrational relaxation. (Alkaline) Re fluoride nanocrystals are also attractive PTMs. The thermal energy generated from these ‘nanoheaters’ upon NIR light irradiation has been employed in a wide-range of applications such as photothermal therapy,65–67 anti-counterfeiting technology68 and nanothermometry.65,69–72 Unfortunately, lanthanide-based nanomaterials are not widely used in photothermal catalysis despite possessing several advantages over fossil fuels traditionally employed in thermally driven reactions: (i) maximum temperature reached within a short timescale (e.g., down to a few picoseconds) and quick transfer of heat to the environment, (ii) temperature enhancement facilely controlled with a high spatial resolution (e.g., nanometre scale), (iii) use of cumbersome external heating appliances avoided, and (iv) elimination of fossil fuel combustion, hence achieving a greener and more sustainable method of producing heat.25,54,73
Given the unique properties of lanthanide-based nanomaterials (e.g., ReF3 and AReF4) it would be advantageous to exploit their upconversion luminescence, LA catalytic activity and photothermal behaviour to effectively drive organic synthesis and polymerization reactions (Fig. 1c). This perspective introduces previously reported studies relevant to this subject and provides a perspective on its future outlook. Briefly, in Section 2, the working mechanisms and applications of lanthanide-based nanocrystals as NIR-activated ‘nanolamps’ in photoredox catalysis and photopolymerization will be presented. Photodegradation of organic pollutants will not be included here since it has been well reviewed in ref. 25–27. The role of lanthanide-based nanocrystals as a heterogeneous LA catalyst is discussed in Section 3. This is followed by a discussion on the mechanisms behind their NIR-activated photothermal effect, factors affecting light-to-heat conversion efficiency and applications to reactions. Finally, a short discussion on the challenges faced by ReF3 and AReF4 nanocrystals as functional heterogeneous catalysts is given.
2. Upconversion nanocrystals
2.1. Photon upconversion
(Alkaline) Re fluoride nanocrystals containing both sensitizer and activator dopants can undergo upconversion luminescence when excited using NIR light. Nd3+ and Yb3+ with light absorption cross-sections of 1.2 × 10−19 cm2 at 808 nm and 1.2 × 10−20 cm2 at 980 nm, respectively, are often employed as the sensitizer, whereas Tm3+ and Er3+ are common activators.4,74,75 A core/shell/shell NaYF4:Yb3+,Er3+@NaYF4@NaYF4:Nd3+,Yb3+,Tm3+ nanocrystal is used here to illustrate the commonly invoked energy transfer upconversion mechanism (Fig. 2).76 The effects of using different excitation wavelengths (i.e., 1540, 980 and 808 nm) on the energy of emitted photons are discussed. The NaYF4 shell prevents cross-talk between the emissive NaYF4:Yb3+,Er3+ core and the NaYF4:Nd3+,Yb3+,Tm3+ shell. Upon 1540 nm light excitation, Er3+ ions in the core undergo multiple excited state absorption processes (4I15/2 → 4I13/2, 4I13/2 → 4I9/2, 4I9/2 → 2H11/2, and 4I11/2 → 4S3/2), followed by blue light emission corresponding to the 2H11/2 → 4I15/2 and 4S3/2 → 4I15/2 transitions and red light emission corresponding to the 4F9/2 → 4I15/2 transition.
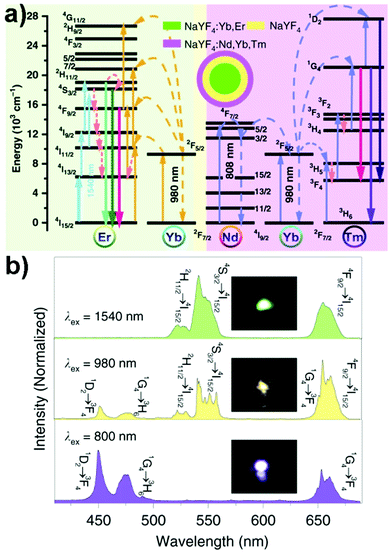 |
| Fig. 2 (a) Proposed multiple excited state absorption and energy transfer mechanism in Nd3+, Yb3+, Er3+, and Tm3+-codoped UCNC.76 (b) Upconversion emission spectra of UCNCs shown in (a) and the corresponding photo of UCNC films excited using λex = 800, 980 and 1540 nm laser excitation.76 Reprinted with permission from ref. 76. Copyright 2020, Nature Publishing Group. | |
When an 808 nm excitation light is used instead, non-radiative energy transfer from the excited Nd3+ to Yb3+ in the outermost shell takes place (4F3/2 (Nd3+) + 2F7/2 (Yb3+) → 4I9/2 (Nd3+) + 2F5/2 (Yb3+)). The excited Yb3+ subsequently transfers its energy to Tm3+, pumping the latter from its 3H6 state to higher energy levels via sequential photon absorption:
2F5/2 (Yb3+) + 3H6 (Tm3+) → 2F7/2 (Yb3+) + 3H5 (Tm3+) |
2F5/2 (Yb3+) + 3F4 (Tm3+) → 2F7/2 (Yb3+) + 3F2 (Tm3+) |
2F5/2 (Yb3+) + 3H4 (Tm3+) → 2F7/2 (Yb3+) + 1G4 (Tm3+) |
2F5/2 (Yb3+) + 1G4 (Tm3+) → 2F7/2 (Yb3+) + 1D2 (Tm3+) |
The excited Tm
3+ subsequently emits blue-violet light by the relaxation pathways
1D
2 →
3F
4 and
1G
4 →
3H
6 and red light by
1G
4 →
3F
4.
When a 980 nm excitation light is used to directly excite the Yb3+ ions, they can undergo non-radiative energy transfer to either Tm3+ in the outermost shell or Er3+ in the core. Energy transfer from the photoexcited Yb3+ to Er3+ gives rise to the population of 2H11/2, 4S3/2 and 4F9/2 states which then emit radiatively. The observed yellowish luminescence is thus derived from upconversion emissions from both Tm3+ and Er3+. Apart from the energy transfer upconversion mechanism, other explanations used to understand the anti-Stokes shift in emission include excited state absorption, photon avalanche, cooperative upconversion and energy migration-mediated upconversion.1,6,12,77
2.2. Photochemical reactions
In 2014, Lee et al. performed a NIR-driven photoenzymatic conversion of α-ketoglutarate to L-glutamate using an UCNC-based system involving both photocatalytic and biocatalytic cycles (Fig. 3a).15 In the former cycle, Rose Bengal (RB) dye molecules, bound onto the surface of a silica shell coated NaYF4:Yb3+,Er3+ core, were excited by upconverted emission light when irradiated with a 980 nm light. The close proximity between RB and UCNC ensures an efficient Förster resonance energy transfer process. In the latter cycle, photoexcited electrons from RB were transferred to NAD+via a Rhodium(III)-based mediator (i.e., [Cp*Rh(bpy)H2O]+, Cp* = C5Me5, bpy = 2,2′-bipyridine); reducing NAD+ to NADH (a nicotinamide cofactor) that was needed for the conversion of α-ketoglutarate to L-glutamate in the presence of L-glutamate dehydrogenase. An L-glutamate yield of ∼20.25% was achieved when the reaction mixture was exposed to NIR light for 5 h.
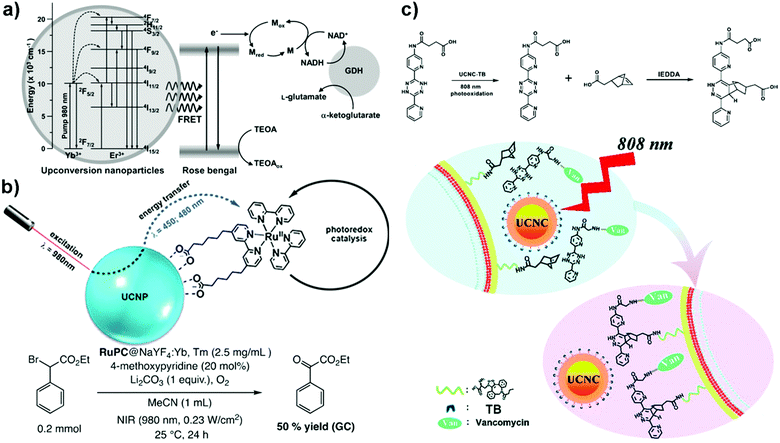 |
| Fig. 3 (a) NIR-driven photoenzymatic catalysts combining NaYF4:Yb3+,Er3+, Rose Bengal (RB), a rhodium(III)-based mediator (M), nicotinamide adenine dinucleotide (NAD+) and L-glutamate dehydrogenase (GDH).15 Reprinted with permission from ref. 15. Copyright 2014, Wiley-VCH. (b) NIR-driven photocatalyst combining NaYF4:Yb3+, Tm3+ and a heteroleptic derivative of [Ru(bpy)3]2+.16 Reprinted with permission from ref. 16. Copyright 2018, Wiley-VCH. (c) NIR-driven photocatalyst combining NaYF4:Nd3+,Yb3+,Er3+@NaYF4:Nd3+ and Toluidine Blue O (TB).17 Reprinted with permission from ref. 17. Copyright 2021, Royal Society of Chemistry. | |
Freitag et al. designed an NIR-activated photocatalyst containing a heteroleptic [Ru(bpy)2L]2+ complex (i.e., L = 6,6′-([2,2′-bipyridine]-4,4′-diyl)dihexanoic acid) attached onto the surface of NaYF4:Yb3+,Tm3+ UCNC via ligand-exchange (Fig. 3b).16 Through careful steady-state and time-resolved luminescence studies, the authors proposed that radiative upconverted energy transfer (i.e., trivial energy transfer mechanism) occurred from the excited UCNCs to the bound transition-metal complex which in turn photocatalysed the overall oxidation of ethyl 2-bromo-2-phenylacetate to ethyl 2-oxo-2-phenylacetate under aerobic conditions.
Recently, our group designed an NIR-activated photocatalyst system comprised of Toluidine Blue (TB) molecules bound onto the surface of an UCNC (i.e., NaYF4:Nd3+,Yb3+,Er3+@NaYF4:Nd3+) (Fig. 3c).17 Upon light irradiation with an 808 nm light, the excited TB, formed after resonance energy transfer from UCNC, were able to photocatalyze the conversion of dihydrotetrazine (dHTz) to tetrazine. The latter subsequently underwent an inverse electron demand Diels–Alder reaction (IEDDA) with a strained dienophile. This concept was applied to covalently attached a dHTz-vancomycin (Van) antibiotic system to norbornene molecules on bacterial cell walls, and the strategy significantly improved the activity of Van against drug-resistant Enterococci (i.e., MIC was reduced by 6- to 7-fold as compared to neat Van). Wu et al. have also labelled sialic acids incorporated into bacterial cells by tetrazole-modified UCNCs via photoclick chemistry.18
2.3. Photopolymerization
Compared to conventional photopolymerization using direct UV/visible light, UCNC-assisted polymerization has several advantages including easier medical treatment procedures (e.g., treatment of carious lesions), enhanced polymerization rate, improved degree of monomer conversion, and increased polymerization depth.77–81 The combination of a UCNC and a photoinitiator allows emitted upconverted photons to excite the latter to produce active species that initiate the polymerization reaction. For example, Liu et al. performed the polymerization of epoxy acrylate by coupling NaYF4:Yb3+,Tm3+ UCNC and the photoinitiator Irgacure 784.81 Due to the deep penetration and weak Rayleigh scattering of NIR excitation light, an impressive curing depth of 13.7 cm was obtained when 0.3 wt% concentration of UCNC was used (Fig. 4a). It is worth noting that the concentration of UCNCs used in the polymerization should be carefully tuned since it significantly affected the degree of monomer conversion and polymerization depth (Fig. 4b).
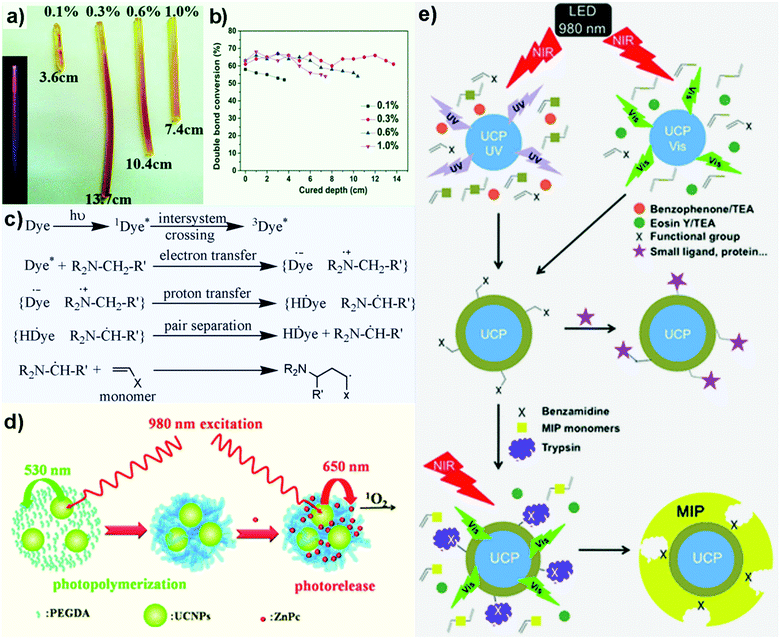 |
| Fig. 4 (a) Curing depths obtained using different concentrations of NaYF4:Yb3+,Tm3+ UCNC.81 (b) Degree of monomer conversion at different curing depth.81 Reprinted with permission from ref. 81. Copyright 2016, Royal Society of Chemistry. (c) Key steps to generate free radicals by the dye/coinitiator system.82 Reprinted with permission from ref. 82. Copyright 2008, Wiley-VCH. (d) Schematic illustration of NIR light-activated photopolymerization of PEGDA hydrogels and NIR light-controlled release of singlet oxygen from ZnPc loaded into UCNP–PEGDA hybrid microspheres.83 Reprinted with permission from ref. 83. Copyright 2013, Royal Society of Chemistry. (e) Schematic illustration of a generalized strategy to coat a polymer shell with different functional groups (X) on UCNC via UCNC-assisted photopolymerization and to further coat a molecularly imprinted polymer (MIP) layer in the presence of a molecular template (e.g., trypsin) and MIP monomers.84 Reprinted with permission from ref. 84. Copyright 2014, Wiley-VCH. | |
Another common method for initiating photopolymerization is to generate free radicals using a photosensitizer (i.e., dye)/coinitiator system.82 Briefly, initiating radicals are produced via the following steps (Fig. 4c): (a) an appropriate organic dye is first photoexcited, (b) a charge-separated radical-ion pair is formed after electron transfer from the coinitiator (e.g., amines R2N–CH2–R′) to the photoexcited dye, (c) a radical pair is formed following proton (H+) transfer within the radical-ion pair, and (d) radical-pair separation leads to initiating radical generation that (e) reacts with the monomer.
Based on the above mechanism, Xiao et al. synthesized poly(ethylene glycol) diacrylate (PEGDA) microspheres using a photoexcited Eosin Y/triethanolamine coinitiator system together with NaYF4:Yb3+,Er3+ UCNCs as internal lamps.83 When the UCNC was irradiated with a 980 nm light, upconverted energy was transferred to Eosin Y, inducing the formation of the initiating radicals (Fig. 4d). In addition, lipophilic zinc(II) phthalocyanine (ZnPc) was successfully loaded into the hydrophobic environment of the UCNC-PEGDA hybrid microspheres via hydrophobic interaction. In this case, green emission (520 to 554 nm) from the UCNC was used to photoinitiate the polymerisation reaction whereas red emission (640 to 680 nm) was used to photoexcite zinc(II) phthalocyanine to generate 1O2 for photodynamic therapy application.
Beyazit et al. provided a general strategy to coat a polymer shell in situ on a UCNC via UCNC-assisted photopolymerization using a 980 nm laser (Fig. 4e).84 Two different UCNCs were utilized: Na0.6K0.4YF4:Yb3+,Tm3+ for UV-light luminescence to excite a benzophenone/triethylamine (TEA) coinitiator system, and NaYF4:Yb3+,Er3+ for visible-light luminescence to excite an Eosin Y/TEA system. After photopolymerization, UCNC core–polymer shell nanostructures were obtained, instead of the aggregates of the UCNC and polymer observed in Xiao and co-workers’ study.83 This method allowed the preparation of polymeric shells with various properties (e.g., hydrophilic or hydrophobic, charged or neutral, containing various functional groups). Interestingly, a second polymeric shell such as a molecularly imprinted polymer (MIP) could also be synthesized on top of the UCNC core–polymer shell nanostructure after re-initiating the polymerization reaction in the presence of a molecular template (e.g., trypsin) and MIP monomers.
Kocaarslan et al. performed free-radical polymerisation of poly (methyl methacrylate) (PMMA) using a tungsten-tellurite glass doped with Yb3+ and Tm3+ ions (UCG) as an internal light source.85 The upconverted photons emitted from the UCG upon NIR light irradiation (975 nm) were used to excite a fluorescein photoinitiator (FL)/pentamethyldiethylene triamine coinitiator system. In addition, free radical-promoted cationic polymerization of oxirane, cyclohexene oxide, isobutyl vinyl ether and N-vinyl carbazole was also performed by first generating α-amino radicals from a FL dye/dimethyl aniline coinitiator system, subsequently oxidized by diphenyl iodonium hexafluorophosphate (Ph2I+PF6−) to form the initiating cations.
Reports on UCNC-assisted cycloaddition polymerization, free-radical polymerization, cationic polymerization, atom transfer radical polymerization, reversible addition–fragmentation chain transfer (RAFT) polymerization and reversible deactivation radical polymerization are found in ref. 86–92. It is important to note that the success of photopolymerization is dependent on a few parameters including a high degree of spectral overlap between the emission of the UCNC (EUCNC(λ)) and absorption of the photoinitiator/photosensitizer (εabs(λ) is the molar extinction coefficient at wavelength λ):93
and the initiation activity of the free radicals as discussed by Zou and co-workers.
94
3. Lewis acid catalyst
Kang et al. have synthesized several hollow mesoporous ReF3 (e.g., LaF3, NdF3 and YF3) nanocrystals in a mixture of tetrabutylammonium hexafluorophosphate (N(Bu)4PF6) and 1-octyl-3-methyl-imidazolium chloride (OmimCl) (Fig. 5).95 It was reported that the Brunauer–Emmett–Teller (BET) surface area of the nanocrystals can be varied by tuning the ratio of the amounts of N(Bu)4PF6 to OmimCl. In addition, the as-prepared nanocrystals have loose structures with several crystal defects that act as LA catalytic sites. The authors tested the catalytic behaviour of their ReF3 nanocrystals by performing the cyanosilylation of benzaldehyde using trimethylsilyl cyanide (TMSCN). At 50 °C and in a solventless environment, the cyanosilylation reaction was completed within 1 min, whereas a significantly lower conversion yield of the substrate was noted when a commercially purchased LaF3, with a lower BET surface area, was used instead (e.g., ∼2.2% for benzaldehyde within 10 min). Clearly, the mesoporous ReF3 nanocrystals exhibited higher catalytic activity due to the presence of more LA sites created by crystal defects. A plausible three-step mechanism for the reaction was proposed (Fig. 5d); coordination of the O in the aldehyde to LA sites on the surface of the nanocrystals (step 1), attack of the CN group in TMSCN on the carbonyl group in the aldehyde (step 2), and isomerisation of the Si(CH3)3 group to form the product (step 3). The same authors also prepared a co-catalyst system by loading noble metals (e.g., Ru nanoparticles) into mesoporous ReF3 nanocrystals (e.g., Ru/LaF3 and Ru/NdF3). The co-catalysts were then used for the efficient hydrogenation of benzene to cyclohexane (Fig. 5e) and levulinic acid to γ-valerolactone (Fig. 5f). In this case, the Ru metal activated H2 whereas ReF3 activated both the benzene ring and levulinic acid's carbonyl group.
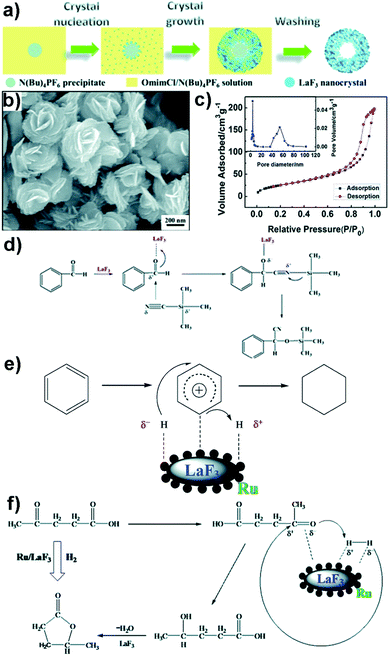 |
| Fig. 5 (a) A scheme for the formation of hollow mesoporous ReF3 (Re is La, Nd, or Y) nanocrystals. (b) SEM image of mesoporous LaF3 nanocrystals. (c) Nitrogen adsorption and desorption isotherms of mesoporous LaF3 nanocrystals shown in (b) (pore-size distribution is included in the inset). (d) Proposed reaction mechanism for the cyanosilylation reaction catalysed by LaF3. (e) Proposed reaction mechanism for the benzene hydrogenation to cyclohexane using the co-catalyst Ru/LaF3. (f) Proposed reaction mechanism for levulinic acid (LA) hydrogenation to γ-valerolactone using the co-catalyst Ru/LaF3.95 Reprinted with permission from ref. 95. Copyright 2015, Royal Society of Chemistry. | |
Yu et al. synthesized hexagonal phase NaScF4 and orthorhombic phase KSc2F7 nanocrystals via the co-thermolysis of Na(CF3COO)/K(CF3COO) and Sc(CF3COO)3 in a binary oleic acid (OA)–oleylamine (OAm) solvent (Fig. 6a and b).96 The nanocrystals were well-dispersed in polar solvents such as ethanol after thermal treatment at 500 °C in Ar/H2 for 1 h to remove the coordinating ligands (Fig. 6c). The cyanosilylation reaction of electron-rich and electron-poor aromatic aldehydes by TMSCN was performed in the presence of either NaScF4 or KSc2F7 nanocrystals to afford excellent product yields under standard conditions (catalyst: 5 mol%; temperature: 25 °C; time: 24 h). In addition, the recycled scandium-based nanocatalysts showed excellent catalytic behaviour even after 10 repeated runs (Fig. 6d).
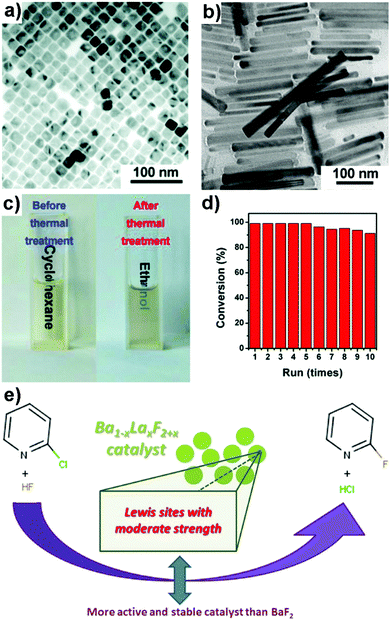 |
| Fig. 6 TEM images of (a) NaScF4 and (b) KSc2F7 nanocrystals. (c) Photograph of NaScF4 nanocrystal solutions before and after thermal treatment at 500 °C under Ar/H2. (d) Reusability test of NaScF4 nanocrystals for 10 cycles in the cyanosilylation reaction.96 Reprinted with permission from ref. 96. Copyright 2017, Royal Society of Chemistry. (e) A scheme for the gas phase fluorination of 2-chloropyridine by HF using Ba1−xLaxF2+x.97 Reprinted with permission from ref. 97. Copyright 2017, Elsevier. | |
Astruc et al. reported the synthesis of Ba1−xLaxF2+x fluoride nanomaterials (e.g., Ba0.5La0.5F2.5 and Ba0.1La0.9F2.9) for the gas phase fluorination of 2-chloropyridine by HF (Fig. 6e).97 The introduction of LaF3 played several important roles in enhancing the catalytic performance of the bimetallic fluoride: (a) LaF3 significantly increased the stability of BaF2 in a wet environment by reducing the sintering of BaF2 in water, (b) LaF3 resulted in a higher surface area and more LA catalytic sites, and (c) LaF3 prevented the chlorination of the BaF2 catalyst by HCl. In a separate study by Li and co-workers, YF3 nanoflowers were demonstrated to be an efficient catalyst for the electrochemical nitrogen reduction reaction under ambient conditions.98 The electrocatalyst elongated the N
N bond and activated it to promote the adsorption of H atoms in various hydrogenation steps to form NH3(g).
4. Photothermal materials
4.1. Light-to-heat conversion mechanism
The most commonly used sensitizer in Re fluoride nanocrystals for photothermal applications is Nd3+ which is excited from its 4I9/2 ground state to an excited state (4F5/2) by ∼800 nm light.69,70,99–101 At this wavelength, the light penetrates deep into biological tissues, and overheating is avoided due to the low absorption of light by water (i.e., absorption coefficients of water at 808 nm and 980 nm are 0.02 cm−1 and 0.48 cm−1, respectively).102,103 In a matrix sparsely doped with Nd3+ (e.g., NaYF4:Nd3+), an excited ion relaxes from the 4F5/2 state to 4F3/2 state before undergoing radiative relaxation to the 4I15/2, 4I13/2, 4I11/2 and 4I9/2 states. Subsequently, non-radiative relaxation from either of the 4Ix/2 (x = 15, 13 and 11) state to the 4I9/2 ground state releases heat (Fig. 7a).70 When the concentration of the dopant is increased (e.g., NaNdF4, NdF3), the distance between Nd3+ ions is reduced and inter-ionic interactions are enhanced. In this case, an excited Nd3+ ion in close proximity to a relaxed Nd3+ ion can undergo efficient cross-relaxation, whereby the excited ion de-excites from the 4F3/2 state to the 4I15/2 state (energy gap = 5749 cm−1), and the partner ion in the ground state is promoted from the 4I9/2 state to the 4I15/2 state (energy gap = 5851 cm−1).99 Both ions in the 4I15/2 state subsequently return to the 4I9/2 ground state via a non-radiative relaxation process that generates heat. Another plausible mechanism to understand the photothermal effect is energy migration between Nd3+ ions followed by energy trapping by non-radiative centres (e.g., surface ligands, defect sites and surrounding solvent) that release heat upon their relaxation.
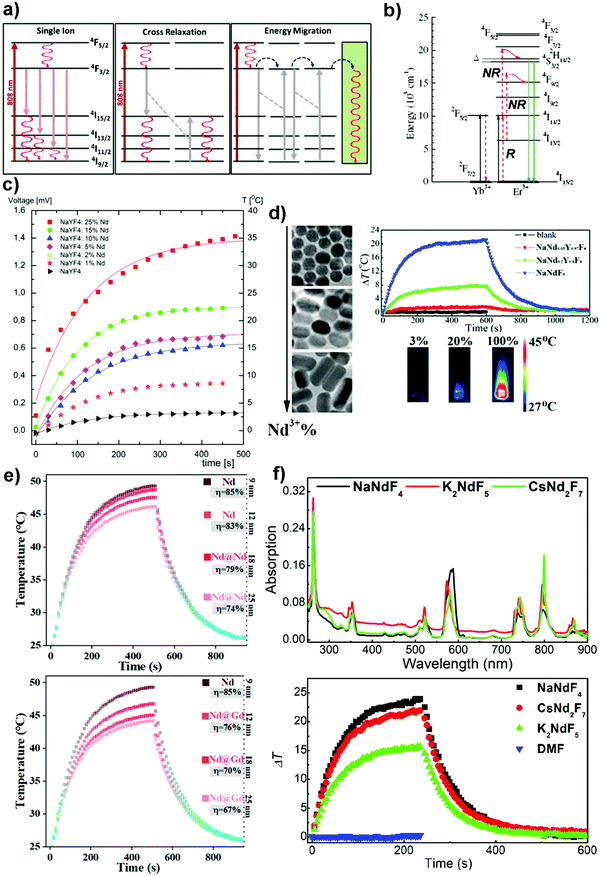 |
| Fig. 7 (a) Simplified energy levels of Nd3+ ions and photophysical processes that lead to photothermal effect after 808 nm light irradiation.70 Reprinted with permission from ref. 70. Copyright 2014, AIP Publishing LLC. (b) Simplified energy levels of Yb3+ and Er3+ ions, and photophysical processes after 975 nm light irradiation.104 Reprinted with permission from ref. 104. Copyright 2009, The Optical Society. (c) Temperature change profiles of a cyclohexane solution of cubic phase NaYF4 nanocrystals doped with Nd3+ (1–25%) excited using an 808 nm laser.99 Reprinted with permission from ref. 99. Copyright 2011, Elsevier. (d) TEM images of NaYF4 nanocrystals doped with different amounts of Nd3+ to illustrate the effects of dopant concentration on the morphology and photothermal performance of NaYF4:Nd3+ (3%, 20% and 100%) nanocrystals.68 Reprinted with permission from ref. 68. Copyright 2019, Royal Society of Chemistry. (e) Effects of the thickness of NaNdF4 (upper panel) and NaGdF4 (lower panel) shells on the temperature change.110 Reprinted with permission from ref. 110. Copyright 2020, American Chemical Society. (f) Absorption spectra of NaNdF4, K2NdF5 and CsNd2F7 nanocrystals (upper panel) and their corresponding temperature change profile (lower panel) obtained after being excited using an 808 nm laser.107 Reprinted with permission from ref. 107. Copyright 2021, American Chemical Society. | |
Tikhomirov et al. have also demonstrated the photothermal effect in a matrix containing both the Yb3+ sensitizer and Er3+ activator ions (i.e., Re10Pb25F65 nanoparticles where Re: Yb3+–Er3+).104 Energy transfer from the photoexcited Yb3+ to Er3+ gave rise to the population of the 2H11/2, 4S3/2 and 4F9/2 states of Er3+ which radiatively emit upconverted energy photons (see discussion in Section 2). At the same time, due to a non-resonant energy transfer process, a mismatch in energy occurred and the excess energy was released as phonon emission (depicted by wavy lines in Fig. 7b) which increased the surrounding temperature. In a separate study, Zhao et al. have shown that non-radiative relaxation of Tm3+ ions in CeO2:Yb3+,Tm3+ nanospheres, after energy transfer from photoexcited Yb3+, did not significantly alter the matrix temperature, and the observed photothermal effect was instead attributed to energy migration between Yb3+ ions before energy trapping by non-radiative surface quenchers.105
4.2. Light-to-heat conversion efficiency determination
The temperature of PTM in photothermal catalysis can be measured using various experimental approaches including non-luminescence methods (e.g., scanning thermal microscopy and scanning transmission electron microscopy) and luminescence methods (e.g., IR thermography, thermoreflectance, optical interferometry and luminescent nanothermometers).73 Among these approaches, IR thermography is the most commonly used to measure the temperature by detecting radiation (i.e., heat) from the targeted PTM. The light-to-heat conversion efficiency (η) of PTM in a solution is determined using the following equation:68,106–108
where h is the heat transfer coefficient, S is the surface area of the container, Tmax is the equilibrium temperature, Tsurr is the ambient surrounding temperature, Qdis is the energy input due to the solvent and container, I0 is the incident laser power and Aexc is the absorbance at the excitation wavelength. Qdis is obtained by measuring the temperature change of the solvent ΔT and is calculated using the following equation:
where m is the mass of the solvent used, C is the heat capacity of the solvent used, ΔT is the obtained increase in the temperature and t is the laser exposure time. In order to obtain hS, a dimensionless driving force temperature θ is defined:
such that when the laser source is turned off, the cooling process that follows can be described by:
t = −τs ln θ |
where T is the temperature of the solution at time t. hS is thus obtained from hS = (mC)/τs. From the cooling curve, τs is obtained by linearly fitting the plot of the cooling time t versus the term −ln
θ.
4.3. Parameters affecting photothermal efficiency
In this section, we briefly discuss the various parameters that affect the photothermal performance of the Re fluoride nanocrystals. Bednarkiewicz et al. have synthesized α-NaYF4 nanocrystals doped with various concentrations of Nd3+ using a thermal deposition method.99 It was noted that when the amount of doped Nd3+ is <25%, the size and morphology of the nanocrystals remain relatively similar. However, the maximum temperature change increased linearly with dopant concentration; a consequence of a stronger cross-relaxation interaction between the Nd3+ ions (Fig. 7c).
Wu et al. demonstrated that the luminescence intensity and lifetime of NaNdxY1−xF4 nanocrystals gradually decreased with an increase in the concentration of Nd3+ whereas the light-to-heat conversion efficiency η increased (e.g., η(NaNd0.03Y0.97F4) ∼ 0.066 < η(NaNd0.2Y0.8F4) ∼ 0.15 < η(NaNdF4) ∼ 0.213).68 The inverse relationship between light-to-heat conversion efficiency and luminescence intensity was also noted when the host matrix was changed to LiYF4. This made the nanocrystals doped with different concentration of Nd3+ good candidates for anti-counterfeiting labels with opposite displays (Fig. 7d).
The effects of the nanocrystal size and laser excitation wavelength on the light-to-heat conversion efficiency of β-NaNdF4 were investigated by Ding and co-workers.109 NaNdF4 nanocrystals of various sizes (i.e., 4.7, 5.9, 12.8, and 15.6 nm) were first prepared by controlling the Ostwald ripening time. It was reported that nanocrystals exhibited better photothermal properties when exposed to an 808 nm laser as compared to a 793 nm laser. Furthermore, the nanocrystal with a diameter of ∼12.8 nm displayed the best photothermal performance, however, an explanation for this observation was not provided.
Li et al. have synthesized β-NaNdF4 nanocrystals of different sizes (i.e., diameter d = 9, 12, 18 and 25 nm) using a hot injection method.110 The light-to-heat conversion efficiency (η) of 85% measured for the NaNdF4 nanocrystals with d = 9 nm was reduced to 74% when d was increased to 25 nm. Depositing an 8 nm thick NaGdF4 shell onto the NaNdF4 core only reduced η by 18% (Fig. 7e). The authors rationalized the above data using the concept of surface quenching suppression, however, they concluded that the main contribution to the photothermal effect came from cross-relaxation interaction between neighbouring Nd3+ ions. In addition, the authors reported that the equilibrium temperature reached increased with an increase in the laser power.
Very recently, we have reported a facile co-precipitation method of synthesizing orthorhombic phase CsNd2F7 and hexagonal phase K2NdF5 nanocrystals that avoids harsh conditions (e.g., high temperatures and long reaction times in hydrothermal synthesis) (Fig. 7f).107 The as-prepared nanocrystals were stable in basic solutions (e.g., 1-octadecene/OAm/OA with OAm:OA molar ratio ∼1.6) but decomposed to hexagonal phase NdF3 or orthorhombic phase KNdF4 in acidic solutions (e.g., 1-octadecene/OA) at a high reaction temperature (e.g., ∼300 °C). In addition, the nanocrystals exhibited good photothermal performance (i.e., η(CsNd2F7) ∼ 26.6 ± 3.4% and η(K2NdF5) ∼ 16.2 ± 1.5%). The absorption spectra of the tetra-, penta- and heptafluoride nanocrystals did not change significantly despite differences in their size, morphology, and crystalline phase. This is different from other PTMs (e.g., gold and silver) whose absorption peaks are strongly dependent on the size and morphology of the nanocrystals.
4.4. Applications of the photothermal effect in catalytic reactions
Recently, we prepared ultrathin (i.e, 3–4 unit cell layers) KNdF4 nanoplates as a synergistic ‘two-in-one’ nanocatalyst, combining both Lewis acid and photothermal effect, for the cyanosilylation of ketones with TMSCN (Fig. 8).108 Near quantitative product yields were observed within a short reaction time (e.g., 4 min) when the reaction mixture containing the KNdF4 nanoplates (∼4.8 mol%) was irradiated with an 808 nm laser. The surface Nd3+ ions endowed LA with catalytic activity whereas the photothermally generated heat improved the reaction rate. Thermal stability is an important requirement for PTM. Even though Au nanostructures have excellent photothermal performances, changes in their size and morphology are usually seen when exposed to light irradiation.57,58 Compared to Au nanostructures, the KNdF4 nanoplates are thermally stable. Their morphology remained relatively intact even after five cycles of cyanosilylation reactions conducted at a reaction temperature of 52 °C.
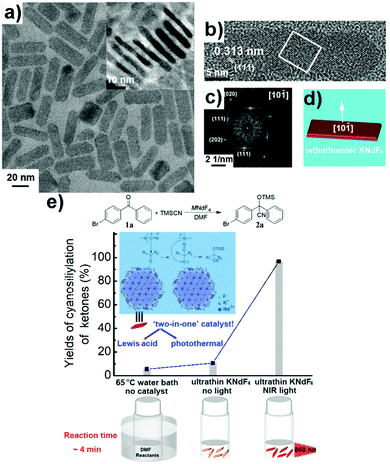 |
| Fig. 8 (a) TEM image of orthorhombic KNdF4 nanoplates (inset shows the TEM image of single nanoplates standing on their edge). (b) HRTEM image of a single nanoplate with a length of 34.1 nm and a width of 10.2 nm. (c) the FFT pattern with [10 ] zone axis for the white-boxed area in (b). (d) Scheme of nanoplate growth. (e) A scheme to show the synergistic effects from NIR light activated KNdF4 nanoplates on the product yields of the cyanosilylation of 4-bromobenzophenone by TMSCN.108 Reprinted with permission from ref. 108. Copyright 2020, Wiley-VCH. | |
5. Conclusion and perspective
In summary, we have introduced the applications of rare-earth fluoride nanocrystals in facilitating organic synthetic and polymerization reactions using either one or a combination of their unique features, namely as a Lewis acid catalyst, upconversion luminescence nanolamp or photothermal nanoheater. This research is still in its early stages of development and growth, and several challenges exist that warrant further investigation as discussed below.
5.1. Enhancing upconversion luminescence and using solar energy
Since IR light accounts for more than half of the solar spectrum (i.e., 51%, 47% and 2% of solar light is made up of IR, visible and UV light, respectively), it would be ideal if solar energy becomes a viable excitation source. By focusing sunlight onto BaCl2:Ho3+ that absorbed maximally at 900, 1157 and 1610 nm using an optical lens, Jia et al. successfully measured its upconversion luminescence.111 To further improve the utilization of solar energy, it is worthwhile to explore the use of effective methods to harvest both visible and IR light of the solar spectrum as an excitation source (e.g., by using luminescent solar concentrators).8,112
One of the limitations of lanthanide-based UCNCs is the low conversion yield of NIR to UV/visible light (<1%). To improve the intensity of upconversion luminescence without the need for increasing laser intensity or concentration of UCNC, several strategies including plasmon-assisted upconversion, core–shell structure design, dye-sensitized UCNC, and host lattice distortion have been proposed.1,9–11,23,103,113 This is particularly useful when overheating, due to the photothermal effect of lanthanide-based UCNC or absorption of excitation light by the solvent, is not desired in a photochemical/photopolymerization reaction. In this case, it is advantageous to be able to produce strong upconversion emission using as low an excitation intensity as possible. The ultimate aim is to employ UCNCs as an efficient NIR-light activated nanolamp to drive scaled-up reactions in industries, and hence the quest to easily and economically prepare brighter UCNCs is both pertinent and necessary.
5.2. Generating more Lewis acid catalytic sites
Since functional groups (e.g., –COO−, –NH2 and –SH) can coordinate with lanthanide ions on the surface of the nanocrystals, capping ligands (e.g., oleate and oleylamine) are usually used in the synthesis to stabilize the (alkaline) Re fluoride nanocrystals.17,68,107,108,114,115 In order to generate more Lewis acid sites, a straightforward approach is to remove the protecting ligands using different strategies such as ligand exchange and thermal treatment.96,108,116 LA sites can also be generated from crystal defects which are usually introduced during the synthesis of nanocrystals. Defects found in nanocrystals with poor crystalline quality and large surface-to-volume ratio can be prepared by tuning the synthesis parameters such as using lower reaction temperatures and shorter reaction times, and introducing extra dopant ions.117
5.3. Improving photothermal performance
The following properties are often considered when designing a functional PTM: (i) strong absorption of light across a wide range of the solar spectrum, (ii) efficient light-to-heat conversion efficiency and (iii) high thermal stability. Similar methods introduced in Section 5.1 can be applied to enhance the absorption of light. On the other hand, options to improve the photothermal effect of lanthanide-based nanomaterials are scarce. By coating NaNdF4 with Prussian Blue (PB), Yu et al. observed a remarkable light-to-heat conversion efficiency of 60.8%.118 The authors attributed the better performance of the NaNdF4@PB core/shell nanocomplex to a new cross-relaxation process between excited Nd3+ and excited PB that populated more of the 4F3/2 state of the lanthanide needed to generate heat. The same technique was also used to enhance the light-to-heat conversion efficiency of a NaErF4@NaYF4@NaNdF4 core/shell/shell nanocrystal.119 Other methods that do not involve the use of dye molecules that may suffer from thermal- and photodegradation are attractive and deserve further investigation.
Re fluoride nanomaterials are non-conducting as the 4f electrons of lanthanides are well shielded by their filled 5s25p6 orbitals; charge carriers generated from photoexcitation do not participate in catalytic reactions.7,11,13,14,120 Instead, these nanomaterials aid in increasing the surrounding temperature and enhancing reaction rates. An area that is currently lacking is the exploitation of Re fluoride nanocrystals as PTMs in conjunction with photoredox catalysts (e.g., MOFs, organometallic complexes, and organic dyes) to drive photocatalytic reactions.
The ratio of the upconversion luminescence intensity of the thermally coupled 2H11/2 → 4I15/2 transition (520 nm) and 4S3/2 → 4I15/2 transition (540 nm) of Er3+ in a tetrafluoride matrix co-doped with Yb3+ and Er3+ has been shown to be a convenient readout in ratiometric thermometry.121,122 By constructing a nanocomposite containing a lanthanide-based nanocrystal in a covalent organic framework that was crafted with Cu ions, Krishnaraj et al. managed to perform simultaneous Cu-catalyzed reaction of indole, aldehyde and malononitrile to yield 2-((1H-indol-3-yl)(phenyl)methyl)malononitrile, and nanothermometry to track the local temperature of the reaction.123 It would therefore be useful to explore the possibility of generating heat from lanthanide-based nanoheaters in catalytic reactions while concurrently measuring the internal temperature of the reaction mixture using the same PTM.
When employing a combination of the upconversion emission and photothermal effect of lanthanide-based nanomaterials, their inverse relationship should be taken into account. To optimize the usage of each property in a single system, the structural design of the nanocrystal by confining each function to a specific area (e.g., upconversion luminescence to the core and photothermal to the shell) may address this issue in future applications.
5.4. Toxicity and environmental impact
Studies on the toxicity of lanthanide-based upconversion nanomaterials have generally shown that they have low toxicity.124,125 For example, ligand-free α-NaYF4:Yb3+,Tm3+ and ligand-free core–shell α-NaYF4:Yb3+,Er3+@NaLuF4 have no side effects when accumulated in human pancreatic cancer cells (Panc 1) and Caenorhabditis elegans worms, respectively.126,127 Caution should still be exercised to ensure that potentially harmful lanthanide ions and ligands are not leached into the solution. Another area of concern is the lack of a standard protocol to test and assess the toxicity of the various lanthanide-based nanomaterials.128 A systematic comparison between nanostructures is best made when a uniform set of factors such as size, morphology, crystalline phase, types of surface ligands, chemical compositions, etc. is considered.
The impact of lanthanide-based nanomaterials on the environment and ecosystem has received less attention. A study previously reported that plants can uptake water soluble NaYF4:Yb3+,Er3+ upconversion nanoparticles through the roots with minimal detrimental effects.129 In fact, the growth of mung bean plants was demonstrated to improve due to better water absorption caused by NaYF4:Yb3+,Er3+@CDs nanocomposites, where CDs are carbon dots.130 Future studies on the positive and negative impact of the interaction of lanthanide-based nanomaterials with soil, water and air are warranted.
Author contributions
X. W. and E. K. L. Y. proposed the topic of this review, interpreted the relevant literature and co-wrote the manuscript.
Conflicts of interest
There are no conflicts to declare.
Acknowledgements
We acknowledge financial support from the Singapore Ministry of Education MoE Tier 1 grant (RG89/20).
References
- S. Han, R. Deng, X. Xie and X. Liu, Enhancing luminescence in lanthanide-doped upconversion nanoparticles, Angew. Chem., Int. Ed., 2014, 53, 2 CrossRef
.
- S. P. Tiwari, S. K. Maurya, R. S. Yadav, A. Kumar, V. Kumar, M.-F. Joubert and H. C. Swart, Future prospects of fluoride based upconversion nanoparticles for emerging applications in biomedical and energy harvesting, J. Vac. Sci. Technol., B, 2018, 36, 060801 CrossRef
.
-
R. Wang and F. Zhang, in Near-infrared Nanomaterials: Preparation, Bioimaging and Therapy Applications, ed. F. Zhang, Royal Society of Chemistry, Cambridge, UK, 2016, ch. 1, pp. 1–39 Search PubMed
.
- Y.-F. Wang, G.-Y. Liu, L.-D. Sun, J.-W. Xiao, J.-C. Zhou and C.-H. Yan, Nd3+-sensitized upconversion nanophosphors: efficient in vivo bioimaging probes with minimized heating effect, ACS Nano, 2013, 7, 7200 CrossRef CAS PubMed
.
-
M. Tan and G. Chen, in Near Infrared-Emitting Nanoparticles for Biomedical Applications, ed. A. Benayas, E. Hemmer, G. Hong and D. Jaque, Springer Nature, Switzerland AG, 2020, ch. 4, pp. 63–81 Search PubMed
.
- W. Yang, X. Li, D. Chi, H. Zhang and X. Liu, Lanthanide-doped upconversion materials: emerging applications for photovoltaics and photocatalysis, Nanotechnology, 2014, 25, 482001 CrossRef PubMed
.
- K. Zheng, K. Y. Loh, Y. Wang, Q. Chen, J. Fan, T. Jung, S. H. Nam, Y. D. Suh and X. Liu, Recent advances in upconversion nanocrystals: Expanding the kaleidoscopic toolbox for emerging applications, Nano Today, 2019, 29, 100797 CrossRef CAS
.
- B. S. Richards, D. Hudry, D. Busko, A. Turshatov and I. A. Howard, Photon upconversion for photovoltaics and photocatalysis: a critical review, Chem. Rev., 2021, 121, 9165 CrossRef CAS PubMed
.
- S. Gai, C. Li, P. Yang and J. Lin, Recent progress in rare earth micro/nanocrystals: soft chemical synthesis, luminescent properties, and biomedical applications, Chem. Rev., 2014, 114, 2343 CrossRef CAS PubMed
.
- X. Zhu, J. Zhang, J. Liu. and Y. Zhang, Recent progress of rare-earth doped upconversion nanoparticles: synthesis, optimization, and applications, Adv. Sci., 2019, 6, 1901358 CrossRef CAS PubMed
.
- X. Li, F. Zhang and D. Zhao, Lab on upconversion nanoparticles: optical properties and applications engineering via designed nanostructure, Chem. Soc. Rev., 2015, 44, 1346 RSC
.
- G. Chen, H. Qiu, P. N. Prasad and X. Chen, Upconversion nanoparticles: design, nanochemistry, and applications in theranostics, Chem. Rev., 2014, 114, 5161 CrossRef CAS PubMed
.
- A. Kar and A. Patra, Impacts of core–shell structures on properties of lanthanide-based nanocrystals: crystal phase, lattice strain, downconversion, upconversion and energy transfer, Nanoscale, 2012, 4, 3608 RSC
.
- R. K. Sharma, A.-V. Mudring and P. Ghosh, Recent trends in binary and ternary rare-earth fluoride nanophosphors: How structural and physical properties influence optical behaviour, J. Lumin., 2017, 189, 44 CrossRef CAS
.
- J. S. Lee, D. H. Nam, S. K. Kuk and C. B. Park, Near-infrared-light-driven artificial photosynthesis by nanobiocatalytic assemblies, Chem. – Eur. J., 2014, 20, 3584 CrossRef CAS PubMed
.
- M. Freitag, N. Möller, A. Rühling, C. A. Strassert, B. J. Ravoo and F. Glorius, Photocatalysis in the dark: near-infrared light driven photoredox catalysis by an upconversion nanoparticle/photocatalyst system, ChemPhotoChem, 2018, 2, 1 CrossRef
.
- Q. Du, X. Wu, W. Bi, B. G. Xing and E. K.-L. Yeow, Increasing antibiotic activity by rapid bioorthogonal conjugation of drug to resistant bacteria using an upconverted light-activated photocatalyst, J. Mater. Chem. B, 2021, 9, 3136 RSC
.
- Y. Wu, J. Zheng, D. Xing and T. Zhang, Near-infrared light controlled fluorogenic
labeling of glycoengineered sialic acids in vivo with upconverting photoclick nanoprobe, Nanoscale, 2020, 12, 10361 RSC
.
- S. Wu, J. P. Blinco and C. Barner-Kowollik, Near-infrared photoinduced reactions assisted by upconverting nanoparticles, Chem. – Eur. J., 2017, 23, 8325 CrossRef CAS
.
- Z. Wang, D. C. Thang, Q. Han, X. Zhao, X. Xie, Z. Wang, J. Lin and B. G. Xing, Near-infrared photocontrolled therapeutic release via upconversion nanocomposites, J. Controlled Release, 2020, 324, 104 CrossRef CAS PubMed
.
- S. Wu and H.-J. Butt, Near-infrared photochemistry at interfaces based on upconverting nanoparticles, Phys. Chem. Chem. Phys., 2017, 19, 23585 RSC
.
- Y. Tang and G. Wang, NIR light-responsive nanocarriers for controlled release, J. Photochem. Photobiol., C, 2021, 47, 100420 CrossRef CAS
.
- Y. Wu, S. Y. Chan, J. Xu and X. Liu, Multiphoton upconversion materials for photocatalysis and environmental remediation, Chem. – Asian J., 2021, 16, 2596 CrossRef CAS PubMed
.
- Z. Zhang, Y. Liu, Y. Fang, B. Cao, J. Huang and K. Liu, Bin Dong, Near-infrared-plasmonic energy upconversion in a nonmetallic heterostructure for efficient H2 evolution from ammonia borane, Adv. Sci., 2018, 5, 1800748 CrossRef PubMed
.
- M.-Q. Yang, M. Gao, M. Hong and G. W. Ho, Visible-to-NIR photon harvesting: progressive engineering of catalysts for solar-powered environmental purification and fuel production, Adv. Mater., 2018, 30, 1802894 CrossRef
.
- A. A. Ansari and M. Sillanpää, Advanced in upconversion nanoparticles based NIR-driven photocatalysts, Renewable Sustainable Energy Rev., 2021, 151, 111631 CrossRef CAS
.
- B. Li, Y. Hu, Z. Shen, Z. Ji, L. Yao, S. Zhang, Y. Zou, D. Tang, Y. Qing, S. Wang, G. Zhao and X. Wang, Photocatalysis driven by near-infrared light: materials design and engineering for environmentally friendly photoreactions, ACS ES&T Eng., 2021, 1, 947 Search PubMed
.
- M. Zhong, Z. Wang, D. Dai, B. Yang, S. Zuo, C. Yao, F. Wu and X. Li, Upconversion hollow nanospheres CeF3 co-doped with Yb3+ and Tm3+ for photocatalytic nitrogen fixation, J. Rare Earths, 2022, 40, 586 CrossRef CAS
.
- C. He, X. Li, X. Chen, S. Ma, X. Yan, Y. Zhang, S. Zuo and C. Yao, Palygorskite supported rare earth fluoride for photocatalytic nitrogen fixation under full spectrum, Appl. Clay Sci., 2020, 184, 105398 CrossRef CAS
.
- S. Zuo, H. Zhang, X. Li, C. Han, C. Yao and C. Ni, Dual active sites boosting photocatalytic nitrogen fixation over upconversion mineral nanocomposites under the full spectrum, ACS Sustainable Chem. Eng., 2022, 10, 1440 CrossRef CAS
.
- W. Fan, H. Bai and W. Shi, Semiconductors with NIR driven upconversion performance for photocatalysis and photoelectrochemical water splitting, CrystEngComm, 2014, 16, 3059 RSC
.
- C. K. Chen, H. M. Chen, C.-J. Chen and R.-S. Liu, Plasmon-enhanced near-infrared-active materials in photoelectrochemical water splitting, Chem. Commun., 2013, 49, 7917 RSC
.
- K. Feng, Z. Cai, D. Huang, L. Li, K. Wang, Y. Li, C. Wang, J. Song, L. Zhao, W. Wei and F. Jiang, Near-infrared-driven water splitting for hydrogen evolution using a Cu2ZnSnS4-based photocathode by the application of upconversion nanoparticles, Sustain, Energy Fuels, 2020, 4, 2669 CAS
.
- M. Zhang, Y. Lin, T. J. Mullen, W.-F. Lin, L.-D. Sun, C.-H. Yan, T. E. Patten, D. Wang and G.-Y. Liu, Improving hematite's solar water splitting efficiency by incorporating rare-earth upconversion nanomaterials, J. Phys. Chem. Lett., 2012, 3, 3188 CrossRef CAS PubMed
.
- S. Reischauer and B. Pieber, Emerging concepts in photocatalytic organic synthesis, iScience, 2021, 24, 102209 CrossRef CAS
.
- F. Strieth-Kalthoff, M. J. James, M. Teders, L. Pitzer and F. Glorius, Energy transfer catalysis mediated by visible light: principles, applications, directions, Chem. Soc. Rev., 2018, 47, 7190 RSC
.
- M. H. Shaw, J. Twilton and D. W.-C. MacMillan, Photoredox catalysis in organic chemistry, J. Org. Chem., 2016, 81, 6898 CrossRef CAS PubMed
.
- G. E.-M. Crisenza and P. Melchiorre, Chemistry glows green with photoredox catalysis, Nat. Commun., 2020, 11, 803 CrossRef
.
- H. Hayashi, B. Wang, X. Y. Wu, S. J. Teo, A. Kaga, K. Watanabe, R. Takita, E. K.-L. Yeow and S. Chiba, Biaryl cross-coupling enabled by photo-induced electron transfer, Adv. Synth. Catal., 2020, 362, 2223 CrossRef
.
- Y. Z. Tan, X. Y. Wu, T. N. Do, H. L. Nguyen, H.-S. Tan, S. Chiba and E. K.-L. Yeow, Electron transfer quenching of Rhodamine 6G by N-methylpyrrole is an unproductive process in the photocatalytic heterobiaryl cross-coupling reaction, J. Phys. Chem. B, 2021, 125, 8550 CrossRef CAS PubMed
.
- A. Mavridi-Printezi, A. Menichetti, M. Guernelli and M. Montalti, Extending photocatalysis to the visible and NIR: the molecular strategy, Nanoscale, 2021, 13, 9147 RSC
.
- B. D. Ravetz, A. B. Pun, E. M. Churchill, D. N. Congreve, T. Rovis and L. M. Campos, Photoredox catalysis using infrared light via triplet fusion upconversion, Nature, 2019, 565, 343 CrossRef CAS
.
- F. Wang, C. Li, H. Chen, R. Jiang, L.-D. Sun, Q. Li, J. Wang, J. C. Yu and C.-H. Yan, Plasmonic harvesting of light energy of Suzuki coupling reactions, J. Am. Chem. Soc., 2013, 135, 5588 CrossRef CAS PubMed
.
- B. D. Ravetz, N. E.-S. Tay, C. L. Joe, M. Sezen-Edmonds, M. A. Schmidt, Y. Tan, J. M. Janey, M. D. Eastgate and T. Rovis, Development of a platform for near-infrared photoredox catalysis, ACS Cent. Sci., 2020, 6, 2053 CrossRef CAS PubMed
.
- C. Kütahya, Y. Yagci and B. Strehmel, Near-infrared photoinduced copper-catalyzed azide-alkyne click chemistry with a cyanine comprising a barbiturate group, ChemPhotoChem, 2019, 3, 1180 CrossRef
.
- A. R.-O. Kosso, N. Sellet, A. Baralle, M. Cormier and J.-P. Goddard, Cyanine-based near infra-red organic photoredox catalysis, Chem. Sci., 2021, 12, 6964 RSC
.
- N. Sellet, M. Cormier and J.-P. Goddard, The dark side of photocatalysis: near-infrared photoredox catalysis for organic synthesis, Org. Chem. Front., 2021, 8, 6783 RSC
.
- J. C. Wedal and W. J. Evans, A rare-earth metal retrospective to stimulate all fields, J. Am. Chem. Soc., 2021, 143, 18354 CrossRef CAS PubMed
.
-
M. Shibasaki, K. Yamada and N. Yoshikawa, in Lewis Acids in Organic Synthesis, ed. H. Yamamoto, Wiley-VCH, Weinheim, 2000, ch. 20, pp. 911–944 Search PubMed
.
- J. A. Cotruvo, Jr., The chemistry of lanthanides in biology: recent discoveries, emerging principles, and technological applications, ACS Cent. Sci., 2019, 5, 1496 CrossRef PubMed
.
- S. Fukuzumi, J. Jung, Y.-M. Lee and W. Nam, Effects of Lewis acids on photoredox catalysis, Asian J. Org. Chem., 2017, 6, 397 CrossRef CAS
.
- H. Pellissier, Recent developments in enantioselective lanthanide-catalyzed transformations, Coord. Chem. Rev., 2017, 336, 96 CrossRef CAS
.
- Z. Hu and D. Zhao, Metal–organic frameworks with Lewis acidity: synthesis, characterization, and catalytic applications, CrystEngComm, 2017, 19, 4066 RSC
.
- C. Song, Z. Wang, Z. Yin, D. Xiao and D. Ma, Principles and applications of photothermal catalysis, Chem. Catal., 2022, 2, 1 CrossRef
.
- C. Chen, Y. Kuang and L. Hu, Challenges and opportunities for solar evaporation, Joule, 2019, 3, 683 CrossRef CAS
.
- M. Gao, L. Zhu, C. K. Peh and G. W. Ho, Solar absorber material and system designs for photothermal water vaporization towards clean water and energy production, Energy Environ. Sci., 2019, 12, 841 RSC
.
- S. Link, C. Burda, B. Nikoobakht and M. A. El-Sayed, Laser-induced shape changes of colloidal gold nanorods using femtosecond and nanosecond laser pulses, J. Phys. Chem. B, 2000, 104, 6152 CrossRef CAS
.
- K. M. Haas and B. J. Lear, Billion-fold rate enhancement of urethane polymerization via the photothermal effect of plasmonic gold nanoparticles, Chem. Sci., 2015, 6, 6462 RSC
.
- M. Kim, J.-H. Lee and J.-M. Nam, Plasmonic photothermal nanoparticles for biomedical applications, Adv. Sci., 2019, 6, 1900471 CrossRef PubMed
.
- Q. Tian, F. Jiang, R. Zou, Q. Liu, Z. Chen, M. Zhu, S. Yang, J. Wang, J. Wang and J. Hu, Hydrophilic Cu9S5 nanocrystals: a Photothermal agent with a 25.7% heat conversion efficiency for photothermal ablation of cancer cells in Vivo, ACS Nano, 2011, 5, 9761 CrossRef CAS PubMed
.
- S. Ariyasu, J. Mu, X. Zhang, Y. Huang, E. K.-L. Yeow, H. Zhang and B. Xing, Investigation of thermally induced cellular ablation and heat response triggered by planar MoS2-based nanocomposite, Bioconjugate Chem., 2017, 28, 1059 CrossRef CAS PubMed
.
- M.-C. Wu, A. R. Deokar, J.-H. Liao, P.-Y. Shih and Y.-C. Ling, Graphene-based photothermal agent for rapid and effective killing of bacteria, ACS Nano, 2013, 7, 1281 CrossRef CAS PubMed
.
- L. Xiao, X. Chen, X. Yang, J. Sun and J. Geng, Recent advances in polymer-based photothermal materials for biological applications, ACS Appl. Polym. Mater., 2020, 2, 4273 CrossRef CAS
.
- T. D. Cong, Z. Wang, M. Hu, Q. Han and B. Xing, Extraspecific manifestation of nanoheater's position effect on distinctive cellular photothermal responses, ACS Nano, 2020, 14, 5836 CrossRef CAS PubMed
.
- K. Zhao, J. Sun, F. Wang, A. Song, K. Liu and H. Zhang, Lanthanide-based photothermal materials: fabrication and biomedical applications, ACS Appl. Bio Mater., 2020, 3, 3975 CrossRef CAS PubMed
.
- A. Gupta, S. Ghosh, M. K. Thakur, J. Zhou, K. Ostrikov, D. Ji and S. Chattopadhyay, Up-conversion hybrid nanomaterials for light- and heat-driven applications, Prog. Mater. Sci., 2021, 121, 100838 CrossRef CAS
.
- D. Jaque, L. M. Maestro, B. del Rosal, P. Haro-Gonzalez, A. Benayas, J. L. Plaza, E. M. Rodríguez and J. G. Solé, Nanoparticles for photothermal therapies, Nanoscale, 2014, 6, 9494 RSC
.
- X. Y. Wu and E. K.-L. Yeow, Tuning the NIR downconversion luminescence and photothermal conversion efficiencies of MNdxY1−xF4 (M = Na and Li) nanocrystals for use in anti-counterfeiting labels with opposite displays, Nanoscale, 2019, 11, 15259 RSC
.
- L. Marciniak, A. Pilch, S. Arabasz, D. Jin and A. Bednarkiewicz, Heterogeneously Nd3+ doped single nanoparticles for NIR-induced heat conversion, luminescence, and thermometry, Nanoscale, 2017, 9, 8288 RSC
.
- U. Rocha, K. U. Kumar, C. Jacinto, J. Ramiro, A. J. Caamaño, J. G. Solé and D. Jaque, Nd3+ doped LaF3 nanoparticles as self-monitored photo-thermal agents, Appl. Phys. Lett., 2014, 104, 053703 CrossRef
.
- Q. Shao, Z. Yang, G. Zhang, Y. Hu, Y. Dong and J. Jiang, Multifunctional lanthanide-doped core/shell nanoparticles: integration of upconversion luminescence, temperature sensing, and photothermal conversion properties, ACS Omega, 2018, 3, 188 CrossRef CAS PubMed
.
- E. C. Ximendes, U. Rocha, K. U. Kumar, C. Jacinto and D. Jaque, LaF3 core/shell nanoparticles for subcutaneous heating and thermal sensing in the second biological-window, Appl. Phys. Lett., 2016, 108, 253103 CrossRef
.
- D. Mateo, J. L. Cerrillo, S. Durini and J. Gascon, Fundamentals and applications of photo-thermal catalysis, Chem. Soc. Rev., 2021, 50, 2173 RSC
.
- X. Xie, N. Gao, R. Deng, Q. Sun and Q.-H. Xu, X. Liu. Mechanistic investigation of photon upconversion in Nd3+-sensitized core-shell nanoparticles, J. Am. Chem. Soc., 2013, 135, 12608 CrossRef CAS PubMed
.
- R. Deng, F. Qin, R. Chen, W. Huang, M. Hong and X. Liu, Temporal full-colour tuning through non-steady-state upconversion, Nat. Nanotechnol., 2015, 10, 237 CrossRef CAS
.
- Y. Ji, W. Xu, N. Ding, H. Yang, H. Song, Q. Liu, H. Ågren, J. Widengren and H. Liu, Huge upconversion luminescence enhancement by a cascade optical field modulation strategy facilitating selective multispectral narrow-band near-infrared photodetection, Light: Sci. Appl., 2020, 9, 184 CrossRef CAS
.
- Q. Li, S. Yuan, F. Liu, X. Zhu and J. Liu, Lanthanide-doped nanoparticles for near-infrared light activation of photopolymerization: fundamentals, optimization and applications, Chem. Rec., 2021, 21, 1681 CrossRef CAS
.
- Z. Chen, X. Wang, S. Li, S. Liu, H. Miao and S. Wu, Near-infrared light driven photopolymerization based on photon upconversion, ChemPhotoChem, 2019, 3, 1077 CrossRef CAS
.
- K. Wang, J. Peña and J. Xing, Upconversion nanoparticle-assisted photopolymerization, Photochem. Photobiol., 2020, 96, 741 CrossRef CAS PubMed
.
- A. Stepuk, D. Mohna, R. N. Grass, M. Zehnde, K. W. Krämer, F. Pellé, A. Ferrier and W. J. Stark, Use of NIR light and upconversion phosphors in light-curable polymers, Dent. Mater., 2012, 28, 304 CrossRef CAS PubMed
.
- R. Liu, H. Chen, Z. Li, F. Shi and X. Liu, Extremely deep photopolymerization using upconversion particles as internal lamps, Polym. Chem., 2016, 7, 2457 RSC
.
- R. Popielarz and O. Vogt, Effect of coinitiator type on initiation efficiency of two-component photoinitiator systems based on eosin, J. Polymer Sci., Part A, 2008, 46, 3519 CrossRef CAS
.
- Q. Xiao, Y. Ji, Z. Xiao, Y. Zhang, H. Lin and Q. Wang, Novel multifunctional NaYF4:Er3+,Yb3+/PEGDA hybrid microspheres: NIR-light-activated photopolymerization and drug delivery, Chem. Commun., 2013, 49, 1527 RSC
.
- S. Beyazit, S. Ambrosini, N. Marchyk, E. Palo, V. Kale, T. Soukka, B. T.-S. Bui and K. Haupt, Versatile synthetic strategy for coating upconverting nanoparticles with polymer shells through localized photopolymerization by using the particles as internal light sources, Angew. Chem., Int. Ed., 2014, 53, 8919 CrossRef CAS PubMed
.
- A. Kocaarslan, S. Tabanli, G. Eryurek and Y. Yagci, Near-infrared free-radical and free-radical-promoted cationic photopolymerizations by in-source lighting using upconverting glass, Angew. Chem., Int. Ed., 2017, 56, 14507 CrossRef CAS PubMed
.
- P. Lederhose, Z. Chen, R. Müller, J. P. Blinco, S. Wu and C. Barner-Kowollik, Near-infrared photoinduced coupling reactions assisted by upconversion nanoparticles, Angew. Chem., Int. Ed., 2016, 55, 12195 CrossRef CAS PubMed
.
- Z. Li, X. Zou, F. Shi, R. Liu and Y. Yagci, Highly efficient dandelion-like near-infrared light photoinitiator for free radical and thiol-ene photopolymerizations, Nat. Commun., 2019, 10, 3560 CrossRef PubMed
.
- X. Meng, H. Lu, Z. Li, C. Wang, R. Liu, X. Guan and Y. Yagci, Near-infrared light induced cationic polymerization based on upconversion and ferrocenium photochemistry, Polym. Chem., 2019, 10, 5574 RSC
.
- Z. Li, J. Zhu, X. Guan, R. Liu and Y. Yagci, Near-infrared-induced cationic polymerization initiated by using upconverting nanoparticles and titanocene, Macromol. Rapid Commun., 2019, 40, 1900047 CrossRef PubMed
.
- W. Zhang, J. He, C. Lv, Q. Wang, X. Pang, K. Matyjaszewski and X. Pan, Atom transfer radical polymerization driven by near-infrared light with recyclable upconversion nanoparticles, Macromolecules, 2020, 53, 4678 CrossRef CAS
.
- L. Hu, Q. Hao, L. Wang, Z. Cui, P. Fu, M. Liu, X. Qiao and X. Pang, The in situ “grafting from” approach for the synthesis of polymer brushes on upconversion nanoparticles via NIR-mediated RAFT polymerization, Polym. Chem., 2021, 12, 545 RSC
.
- C. Ding, J. Wang, W. Zhang, X. Pan, Z. Zhang, W. Zhang, J. Zhu and X. Zhu, Platform of near-infrared light-induced reversible deactivation radical polymerization: upconversion nanoparticles as internal light sources, Polym. Chem., 2016, 7, 7370 RSC
.
- D. Oprych and B. Strehmel, Mediated generation of conjugate acid by UV and blue sensitizers with upconversion nanoparticles at 980 nm, Chem. – Eur. J., 2021, 27, 4297 CrossRef CAS PubMed
.
- X. Zou, J. Zhu, P. Hu and R. Liu, Methods to evaluate near-infrared photoinitiating systems for photopolymerisation reactions assisted by upconversion materials, ChemPhotoChem, 2021, 5, 915 CrossRef CAS
.
- X. Kang, W. Shang, Q. Zhu, J. Zhang, T. Jiang, B. Han, Z. Wu, Z. Li and X. Xing, Mesoporous inorganic salts with crystal defects: unusual catalysts and catalyst supports, Chem. Sci., 2015, 6, 1668 RSC
.
- B. Yu, E. Hao, S. Fang, Z. Liu, Y. Wang, Z. Lv, N. Li, X. Zhang, L. Shi and Y. Du, Controlled synthesis of high quality scandiumbased nanocrystals as promising recyclable catalysts for silylcyanation reaction, Nanoscale, 2017, 9, 10987 RSC
.
- A. Astruc, S. Célérier, E. Pavon, A.-S. Mamede, L. Delevoye and S. Brunet, Mixed Ba1−xLaxF2+x fluoride materials as catalyst for the gas phase fluorination of 2-chloropyridine by HF, Appl. Catal., B, 2017, 204, 107 CrossRef CAS
.
- T. Li, Q. Chen, J. Yu, J. Xia, Y. Li, K. Xu, Y. Luo, Q. Liu and H. Guo, YF3: a nanoflower-like catalyst for efficient nitrogen fixation to ammonia under ambient conditions, Catal. Sci. Technol., 2021, 11, 6750 RSC
.
- A. Bednarkiewicz, D. Wawrzynczyk, M. Nyk and W. Strek, Optically stimulated heating using Nd3+ doped NaYF4 colloidal near infrared nanophosphors, Appl. Phys. B, 2011, 103, 847 CrossRef CAS
.
- A. Bednarkiewicz, D. Wawrzynczyk, M. Nyk and W. Strek, Synthesis and spectral properties of colloidal Nd3+ doped NaYF4 nanocrystals, Opt. Mater., 2011, 33, 1481 CrossRef CAS
.
- A.-R. Hong, J. Y. Byun, K. Lee and H. S. Jang, Sub-20 nm LiErF4-based upconversion nanophosphors for simultaneous imaging and photothermal therapeutics, ACS Appl. Nano Mater., 2020, 3, 8662 CrossRef CAS
.
- B. Liu, C. Li, P. Yang, Z. Hou and J. Lin, 808-nm-light-excited lanthanide-doped nanoparticles: rational design, luminescence control and theranostic applications, Adv. Mater., 2017, 29, 1605434 CrossRef PubMed
.
- S. T. Dibaba, X. Ge, W. Ren and L. Sun, Recent progress of energy transfer and luminescence intensity boosting mechanism in Nd3+-sensitized upconversion nanoparticles, J. Rare Earths, 2019, 37, 791 CrossRef CAS
.
- V. K. Tikhomirov, K. Driesen, V. D. Rodriguez, P. Gredin, M. Mortier and V. V. Moshchalkov, Optical nanoheater based on the Yb3+–Er3+ co-doped nanoparticles, Opt. Express, 2009, 17, 11794 CrossRef CAS PubMed
.
- X. Zhao, H. Suo, Z. Zhang and C. Guo, Upconverting CeO2: Yb3+/Tm3+ hollow nanospheres for photo-thermal sterilization and deep-tissue imaging in the first biological window, Ceram. Int., 2019, 45, 21910 CrossRef CAS
.
-
X. Huang and M. A. El-Sayed, in Nanotechnology in Biology and Medicine Methods, Devices, and Applications, ed. T. Vo-Dinh, Taylor & Francis Group, Boca Raton, 2nd edn, 2018, ch. 28, pp. 627–650 Search PubMed
.
- X. Y. Wu, J. Y. Tham, A.-M. Chacko and E. K.-L. Yeow, The role of ligand acid–base reaction in the facile synthesis of alkali metal neodymium penta- and heptafluoride nanocrystals, Chem. Mater., 2021, 33, 8367 CrossRef CAS
.
- X. Y. Wu and E. K.-L. Yeow, Ultrathin near-infrared light activated nano-hotplate catalyst, Small, 2020, 16, 2002698 CrossRef CAS PubMed
.
- L. Ding, F. Ren, Z. Liu, Z. Jiang, B. Yun, Q. Sun and Z. Li, Size-dependent photothermal conversion and photoluminescence of theranostic NaNdF4 nanoparticles under excitation of differentwavelength lasers, Bioconjugate Chem., 2020, 31, 340 CrossRef CAS PubMed
.
- L. Xu, J. Li, K. Lu, S. Wen, H. Chen, M. K. Shahzad, E. Zhao, H. Li, J. Ren, J. Zhang and L. Liu, Sub-10 nm NaNdF4 nanoparticles as near-infrared photothermal probes with self-temperature feedback, ACS Appl. Nano Mater., 2020, 3, 2517 CrossRef CAS
.
- H. Jia, Z. Liu, L. Liao, Y. Gu, C. Ding, J. Zhao, W. Zhang, X. Hu, X. Feng, Z. Chen, X. Liu and J. Qiu, Upconversion luminescence from Ln3+(Ho3+, Pr3+) ion-doped BaCl2 particles via NIR light of sun excitation, J. Phys. Chem. C, 2018, 122, 9606 CrossRef CAS
.
- D. Cambié, J. Dobbelaar, P. Riente, J. Vanderspikken, C. Shen, P. H. Seeberger, K. Gilmore, M. G. Debije and T. Noël, Energy-efficient solar photochemistry with luminescent solar concentrator based photomicroreactors, Angew. Chem., Int. Ed., 2019, 131, 14512 CrossRef
.
- T. Sheng, M. Xu, Q. Li, Y. Wu, J. Zhang, J. Liu, X. Zhu and Y. Zhang, Elucidating the role of energy management in making brighter, and more colorful upconversion nanoparticles, Mater. Today Phys., 2021, 20, 100451 CrossRef CAS
.
- H.-X. Mai, Y.-W. Zhang, R. Si, Z.-G. Yan, L.-D. Sun, L.-P. You and C.-H. Yan, High-quality sodium rare-earth fluoride nanocrystals: controlled synthesis and optical properties, J. Am. Chem. Soc., 2006, 128, 6426 CrossRef CAS PubMed
.
- A. Gee and X. Xu, Surface functionalization of upconversion nanoparticles with different moieties for biomedical applications, Surfaces, 2018, 1, 96 CrossRef
.
- A. Dong, X. Ye, J. Chen, Y. Kang, T. Gordon, J. M. Kikkawa and C. B. Murray, A generalized ligand-exchange strategy enabling sequential surface functionalization of colloidal nanocrystals, J. Am. Chem. Soc., 2011, 133, 998 CrossRef CAS PubMed
.
- H. Dong, L.-D. Sun and C.-H. Yan, Local structure engineering in lanthanide-doped nanocrystals for tunable upconversion emissions, J. Am. Chem. Soc., 2021, 143, 20546 CrossRef CAS PubMed
.
- Z. Yu, W. Hu, H. Zhao, X. Miao, Y. Guan, W. Cai, Z. Zeng, Q. Fan and T. T.-Y. Tan, Generating new cross-relaxation pathways by coating Prussian blue on NaNdF4 to fabricate enhanced photothermal agents, Angew. Chem., Int. Ed., 2019, 58, 8536 CrossRef CAS PubMed
.
- X. Wang, H. Li, F. Li, X. Han and G. Chen, Prussian blue-coated lanthanide-doped core/shell/shell nanocrystals for NIR-II image-guided photothermal therapy, Nanoscale, 2019, 11, 22079 RSC
.
- Y. Wu, J. Xu, X. Qin, J. Xu and X. Liu, Dynamic upconversion multicolour editing enabled by molecule-assisted opto-electrochemical modulation, Nat. Commun., 2021, 12, 2022 CrossRef CAS PubMed
.
- R. G. Geitenbeek, A.-E. Nieuwelink, T. S. Jacobs, B. B.-V. Salzmann, J. Goetze, A. Meijerink and B. M. Weckhuysen, In situ luminescence thermometry to locally measure temperature gradients during catalytic reactions, ACS Catal., 2018, 8, 2397 CrossRef CAS PubMed
.
- A. M. Kaczmarek, H. S. Jena, C. Krishnaraj, H. Rijckaert, S. K.-P. Veerapandian, A. Meijerink and P. Van Der Voort, Luminescent ratiometric thermometers based on a 4f–3d grafted covalent organic framework to locally measure temperature gradients during catalytic reactions, Angew. Chem., Int. Ed., 2021, 60, 3727 CrossRef CAS PubMed
.
- C. Krishnaraj, H. Rijckaert, H. S. Jena, P. Van Der Voort and A. M. Kaczmarek, Upconverting Er3+–Yb3+ inorganic/covalent organic framework core–shell nanoplatforms for simultaneous catalysis and nanothermometry, ACS Appl. Mater. Interfaces, 2021, 13, 47010 CrossRef CAS PubMed
.
- G. Jalani, V. Tam, F. Vetrone and M. Cerruti, Seeing, targeting and delivering with upconverting nanoparticles, J. Am. Chem. Soc., 2018, 140, 10923 CrossRef CAS PubMed
.
- A. Gnach, T. Lipinski, A. Bednarkiewicz, J. Rybka and J. A. Capobianco, Upconverting nanoparticles: assessing the toxicity, Chem. Soc. Rev., 2015, 44, 1561 RSC
.
- M. Nyk, R. Kumar, T. Y. Ohulchanskyy, E. J. Bergey and P. N. Prasad, High contrast in vitro and in vivo photoluminescence bioimaging using near infrared to near infrared up-conversion in Tm3+ and Yb3+ doped fluoride nanophosphors, Nano Lett., 2008, 8, 3834 CrossRef CAS PubMed
.
- A. Lay, O. H. Sheppard, C. Siefe, C. A. McLellan, R. D. Mehlenbacher, S. Fischer, M. B. Goodman and J. A. Dionne, Optically robust and biocompatible mechanosensitive upconverting nanoparticles, ACS Cent. Sci., 2019, 5, 1211 CrossRef CAS PubMed
.
- H. Oliveira, A. Bednarkiewicz, A. Falk, E. Fröhlich, D. Lisjak, A. Prina-Mello, S. Resch, C. Schimpel, I. V. Vrček, E. Wysokińska and H. H. Gorris, Critical considerations on the clinical translation of upconversion nanoparticles (UCNPs): recommendations from the European upconversion network (COST Action CM1403), Adv. Healthcare Mater., 2019, 8, 1801233 CAS
.
- A. Hischemöller, J. Nordmann, P. Ptacek, K. Mummenhoff and M. Haase, In-vivo imaging of the uptake of upconversion nanoparticles by plant roots, J. Biomed. Nanotechnol., 2009, 5, 278 CrossRef PubMed
.
- X. Xu, W. Li, C. Hu, B. Lei, X. Zhang, Y. Li, Q. Zhan, Y. Liu and J. Zhuang, Promoting the growth of mung bean plants through uptake and light conversion of NaYF4:Yb,Er@CDs nanocomposites, ACS Sustainable Chem. Eng., 2020, 8, 9751 CrossRef CAS
.
|
This journal is © the Owner Societies 2022 |
Click here to see how this site uses Cookies. View our privacy policy here.