DOI:
10.1039/C9QM00639G
(Review Article)
Mater. Chem. Front., 2020,
4, 1328-1339
The power of dissociation: development of displacement assays for chemosensing and latent catalytic systems†
Received
15th October 2019
, Accepted 25th February 2020
First published on 26th February 2020
Abstract
Disassembly is an essential process in supramolecular chemistry, and its exploitation has resulted in the development of four different displacement assays: (a) indicator-displacement assay (IDA), (b) indicator/catalyst-displacement assay (ICDA), (c) catalytic/indicator-displacement assay (CIDA), and (d) catalyst-displacement assay (CDA). These consist of bimetallic donor–acceptor ensembles (BmDAEs), which incorporate various metallic signal transducers, receptors, inhibitors, catalysts, and pre-catalysts. Applications thereof include pollutant degradation, signal amplification, chemosensing detection, and latent catalysis. This review discusses the use of bimetallic supramolecular systems for the development of catalysts, chemosensors, and chemodosimeters.
1. Introduction
Supramolecular chemistry is the study of the association, dissociation, and exchange of molecules.1 Reversibility and dynamics are the characteristics that originate from these three processes.2 Initially, during the development of supramolecular chemistry, much attention was focused on exploiting intermolecular interactions to self-assemble molecules to create functional materials,3a catalysts,3b chemosensors,3c drug delivery vehicles,3d and molecular machines.3e Recently, the molecular exchange has been used to show the adaptability and evolution of supramolecular systems in response to environmental changes.4 However, the disassembly in supramolecular chemistry remains relatively underexplored.5–7
Disassembly and its reverse process (self-assembly) are ubiquitous and significant in nature. A well-established example of these processes is cytoskeleton dynamics, in which the constant dissociation and association of actin filaments mediate cell growth, motility, and membrane internalization.5 Based on the same principle, various sensing disassembly assays, including antibody-based immunoassays,6a enzyme-linked immunosorbent assays,6b and indicator displacement assays,6c have been developed to detect antigens, proteins, and pollutants, respectively, for clinical diagnoses, biopharmaceutical analysis, environmental monitoring, and food testing. These detections often use the presence of an analyte to dissociate the intermolecular interaction(s) of receptor–chromophore ensemble by forming a new receptor–analyte adduct and releasing a chromophore for chemosensing detection. Another significant application of supramolecular disassembly assays is in the development of latent catalytic systems, in which a “smart off–on” catalytic function is achieved by the use of heat, light, mechanical stimuli, or chemical agents to cleave an inhibitor from the catalyst.7
Bimetallic donor–acceptor ensembles (BmDAEs) are of particular interest in the chemosensing and catalytic sciences. The judicious choice of the employed transition metals, ligands, and bridging units allows the tuning of the intrinsic structural, catalytic, spectroscopic, and spectrofluorometric properties of the bimetallic-based systems,8 and the bifunctional properties of the latter can be rationally controlled for synergistic9a or antagonistic9b sensing and catalytic effects. While recent reports have covered several different perspectives of supramolecular chemistry, this review will focus mainly on the recent developments of disassembly assay-based bimetallic donor–acceptor catalytic and chemosensing systems based on the disassembly assays as vital tools for pollutant degradation and detection.
2. Indicator-displacement assay (IDA) for chemodosimetric detection
The incidence of hazardous toxic substances and persistent organic pollutants in food and in the environment can create significant problems for society. The development of practical molecular detectors for toxic chemicals represents a novel solution to this problem. Over the past decades, significant studies on the use of supramolecular chemosensors and chemodosimeters for the detection of toxins in food, environmental pollutants, and metabolites in living organisms have been reported.10 Synthetic design, binding and signal triggering mechanisms, sensing reversibility, selectivity, and sensitivity are the essential factors that must be considered for the development of these detecting systems. By definition, both chemosensors and chemodosimeters are molecular detectors composed of a recognition unit (binding site) and a signal transduction unit (signaling site) linked by a covalent11a and/or supramolecular interaction.11b,c In the presence of an analyte, the signal transducer produces a sensing response, such as electrochemical,12a color,12b and/or luminescent change12cvia its interaction(s) with the receptor.12 Although both types of supramolecular devices have been developed for molecular detection, chemosensors are suitable for reversible and real-time detection, whereas chemodosimeters are used for irreversible and cumulative detection.13
Supramolecular dissociation has become a useful tool for the development of chemodosimeters. Recently, indicator displacement assays (IDAs) have been exploited as an irreversible approach to detect analytes.14a–e This assay uses the dissociation of the supramolecular linkage(s) between the recognition and signal transduction units induced by the analyte to produce a dosimetric signal. The working principle of the IDA involves (i) initial binding of a chromophore to a receptor forming a “receptor–indicator ensemble”, (ii) introduction of a competitive analyte into the system, causing dissociation of the ensemble and triggering of an optical signal from the release of an indicator, and (iii) formation of a new “receptor–analyte adduct”. The irreversibility of these processes enhances the suitability of chemodosimeters particularly for quality monitoring, as the detection of pollution, spoilage or disease is unequivocal.
2.1 Bimetallic donor–acceptor ensembles (BmDAEs)
We have developed a series of BmDAEs for the IDA detection of zwitterions,15a,b anions,15c,d and neutral organic molecules.15e–j The following section covers the effect of variation of the (i) metallic receptor (MA), (ii) metallic signal-transducer (MB), (iii) auxiliary ligands (Ligand), and (iv) bridging unit (Bridge), on the chemodosimetric selectivity and sensitivity of the BmDAE (Scheme 1).
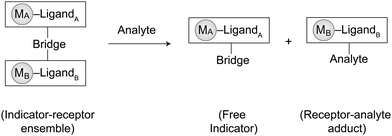 |
| Scheme 1 Bimetallic donor–acceptor ensemble (BmDAEs) for the detection of analytes via the IDA. | |
The BmDAE RuII(phen)2(CN)2–[PtII(DMSO)Cl2]2 (1, phen = 1,10-phenanthroline and DMSO = dimethyl sulfoxide) was prepared for the detection of amino acids in aqueous media (Fig. 1).15a The three metal centers in the BmDAE adopt a V-shaped configuration. The two PtII(DMSO)Cl2 receptors recognize the sulfide moieties (R-SH)16a of amino acids and quench the triplet metal-to-ligand charge transfer (3MLCT) luminescence of the RuII–diimine center.16b The formation of 1 is shown in eqn (1), and its Gibbs free energy (ΔG°) was determined to be −24.6 kJ mol−1 by the expression ΔG° = −RT
ln
K, where R is the universal gas constant, T is the temperature, and K is the experimental formation constant (determined at 1 atm and 298 K), unless otherwise stated.
| RuII(phen)2(CN)2 + 2PtII(DMSO)2Cl2 → RuII(phen)2(CN)2–[PtII(DMSO)Cl2]2 + 2DMSO | (1) |
| Hcys + PtII(DMSO)2Cl2 → PtII(DMSO)(Hcys)Cl2 + DMSO | (2) |
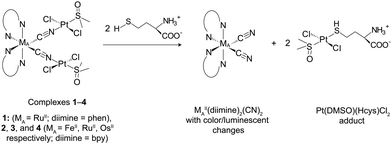 |
| Fig. 1 The detection of Hcys by complexes 1–4via the IDA. | |
From eqn (1), it is expected that any amino acids that can form a more stable PtII(DMSO)(amino acid)Cl2 adduct with the receptors in 1 will cause the BmDAE to dissociate, releasing RuII(phen)2(CN)2. The sulfide group in homocysteine (Hcys: HOOC–CH(NH2)CH2CH2–SH) was used to test this hypothesis. The ΔG° of eqn (2) was determined to be −27.4 kJ mol−1. In the presence of Hcys, the dissociation of PtII from the bridging CN ligands was concomitant with the formation of the more stable PtII(DMSO)(Hcys)Cl2 complex, which was detected by ESI-MS. This disassembly not only resulted in the formation of PtII–Hcys adduct, but also in the release of RuII(phen)2(CN)2 which triggered a luminescent change. Finally, the generated optical signal was directly proportional to the concentration of Hcys (Fig. 1).
2.2 Tuning the IDA sensitivity by varying the MA unit in BmDAEs
To achieve high sensitivity for an IDA, a chromotropic donor that can produce a large optical change upon cleavage of the bimetallic donor–acceptor linkage is key to success. A common strategy is to use the dissociation to disrupt the electron-transfer mechanism in the BmDAE, so that the metallic signal-transducer exhibits two different optical outputs in its free and bound phases during the IDA. The solvatochromic studies of MIIA(bpy)2(CN)2 (MIIA = FeII, RuII, and OsII; and bpy = 2,2′-bipyridine) revealed that the MLCT transitions of these complexes can undergo a large Stokes shift by coordination of an electron metallic acceptor via its cyano-bridges.16c Therefore, the three bimetallic complexes MIIA(bpy)2(CN)2–[PtII(DMSO)Cl2]2 with MIIA = FeII (2), RuII (3), and OsII (4) were synthesized for the detection of Hcys (Fig. 2).15b The crystal structures of complexes 2–4 reveal a V-shaped configuration with two Pt(DMSO)Cl2 acceptors bonded to their corresponding MIIA donor through cyano bridges. The calculated ΔG° of the formation of 2, 3, and 4 were −24.5 kJ mol−1, −24.6 kJ mol−1, and −25.5 kJ mol−1, respectively. Under the abovementioned mechanism (Section 2.1), all complexes could detect Hcys via the IDA (the ΔG° of PtII(DMSO)(Hcys)Cl2 is −27.4 kJ mol−1). Interestingly, by using different chromotropic signal transducers in the BmDAEs, different colorimetric or luminometric responses could be observed. In the presence of Hcys, the yellowish-orange complex 2 yielded a reddish-purple optical colorimetric signal, while the yellow complex 4 changed to greenish-yellow and the weakly luminescent complex 3 provided a strong orange luminescence. The optical responses originated from the perturbance of the MLCT and 3MLCT of signal transducers, MIIA(bpy)2(CN)2, upon assembly and disassembly with the PtII acceptor.16d The detection limits of complexes 2, 3, and 4 for Hcys were 17.4 mg L−1, 5.4 mg L−1, and 13.9 mg L−1, respectively.17
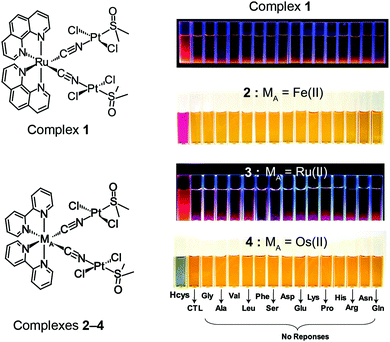 |
| Fig. 2 Structures and chemodosimetric responses of the BmDAEs, 2–4, towards different amino acids in an aqueous medium. | |
2.3 Tuning the IDA selectivity by varying the BmDAEs components
2.3.1 Varying the MB receptor unit in BmDAEs.
The development of BmDAEs for the selective IDA detection of analytes without perturbation by interferences is essential. A straightforward method involves the use of a specific receptor, MB, to achieve excellent selectivity.18 A RuII–CuII-based BmDAE, {RuII(tBubpy)(CN)4–[CuII(dien)]2}(ClO4)2 (5, tBubpy = 4,4′-di-tert-butyl-2,2′-bipyridine and dien = diethylenetriamine),15c was synthesized for the detection of the extremely toxic cyanide anion (Fig. 3a).19 The CuII metallic receptor was chosen not only because it coordinates the cyanide strongly in aqueous media,20 but also because if functions as a luminescent quencher for the 3MLCT of [RuII(tBubpy)(CN)4]2−. The crystallographic analysis of 5 reveals a one-dimensional polymeric structure in which the RuII metallic centers are cyano-bridged to two CuII receptors each. In the spectroscopic and spectrofluorimetric titration experiments with 5, the selective chemodosimetric response to the cyanide anion consisted of a color change from green to orange and a luminescence change from nil to orange. No optical and luminescent signals were generated by 5 in the presence of potentially interfering anions (including SO42−, HCO3−, HPO42−, N3−, CH3COO−, NCS−, NO3−, and Cl−). The detection limit of 5 for cyanide was determined to be 0.03 mg L−1. A detailed thermodynamic study was performed to explain the observed selectivity. Fig. 3b shows the ΔG° of the formation of various [CuII(dien)–anion] adducts. Only the [CuII(dien)(CN)2] adduct exhibited a smaller ΔG° (−37.9 kJ mol−1) than that of 5 (−29.1 kJ mol−1). This suggests that the driving force for the cleavage of 5 by CN− is the formation of the more stable [CuII(dien)(CN)2] species, which explains the selectivity of 5 toward cyanide.
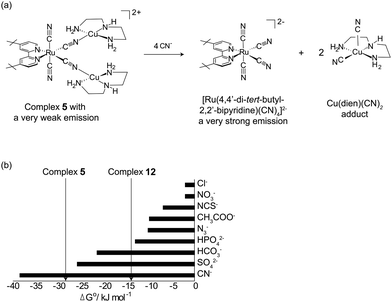 |
| Fig. 3 (a) The detection of cyanide by complex 5via the IDA; (b) binding strengths (ΔG°) of various [Cu(dien)–analyte] adducts. The vertical lines represent the ΔG° values of complexes 5 and 12. | |
By changing the metallic receptor from CuII to NdIII, an acceptor which is well-known to have a strong affinity to amino group, a RuII–NdIII-based BmDAE, K{[NdIII(H2O)4]–[RuII(tBubpy)(CN)4]2} (6) was synthesized for detecting biogenic amine. Histamine, which is one of the most important biogenic amines and the causative agent of scombroid poisoning,21,22 is of particular interest due to its impact in areas ranging from biomarkers of diseases21d and to quality control of foodstuffs.21e Complex 6 was formed by stirring two equivalents of K2[RuII(tBubpy)(CN)4] with one molar equivalent of NdIII(NO3)3·hydrate in a water/methanol mixture in an open atmosphere at 298 K. Fig. 4 shows its molecular structure, in which two independent [RuII(tBubpy)(CN)4]2− units and one NdIII center are connected by two RuII–C
N–NdIII cyanide bridges, forming a V-shaped configuration.15f Upon exposure of different biogenic vapors to complex 6, only histamine induced a spectrofluorometric enhancement of the testing solution, whereas other common food odor chemicals including aniline, H2S, CO, N2, CH4, H2, and atmospheric air did not induce any observable spectrofluorometric changes. It was found that the detection proceeded via the abovementioned IDA mechanism, in which the cyano-bridges of the trinuclear complex (CN–NdIII) are cleaved after binding of histamine to the lanthanide center. The observation of {K[RuII(tBubpy)(CN)4]}− (m/z = 513) in the electrospray ionization mass spectrometry of the complex 6-histamine mixture confirmed this suggestion. A solid-supported chemodosimetric material for histamine sensing was fabricated by blending submicron-rods of complex 6 (length = 2.1 ± 0.6 μm and width = 230 ± 15 μm) with a porous polystyrene film (Fig. 4b).15f The detection limit of the prepared system for histamine vapor was 15 mg L−1.
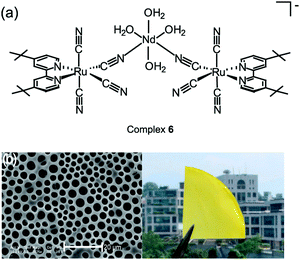 |
| Fig. 4 (a) The structure of complex 6; (b) the photos of a porous polystyrene film where the sub-micron rods of complex 6 were immobilized. | |
2.3.2 Varying the bridging unit in BmDAEs.
The development of an appropriate supramolecular linkage between the receptor and the indicator in BmDAEs is another key for achieving a high chemodosimetric selectivity of the IDA. If the bridge between the donor and acceptor is too strong, the analyte cannot dissociate the ensemble, whereas a too weak linkage is susceptible to dissociation by interferents. The two ReI–bridge–PtII BmDAEs [ReI(tBubpy)(CO)3(NCS)]–[PtII(DMSO)(Cl)2] (7) and [ReI(tBubpy)(CO)3(CN)]–[PtII(DMSO)(Cl)2] (8), featuring different bridging linkages (isothiocyano- and cyano-bridge) were synthesized for the detection of mercapto-containing pesticides in freshwater (Fig. 5a).15g Complexes 7 and 8 were synthesized by stirring equimolar amounts of Pt(DMSO)2Cl2 and ReI(tBubpy)(CO)3(NCS) or ReI(tBubpy)(CO)3(CN), respectively, in methanol–chloroform mixture at ambient conditions. The νN
C
S peak of ReI(tBubpy)(CO)3(NCS) at 2161 cm−1 shifted to 2088 cm−1 for 7 while the νC
N peak of ReI(tBubpy)(CO)3(CN) at 2117 cm−1 shifted to 2182 cm−1 for 8.
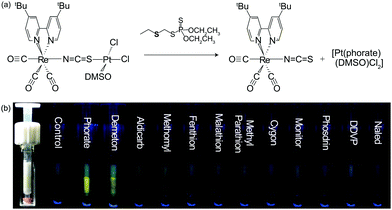 |
| Fig. 5 (a) IDA of complex 7 for the detection of phorate; (b) photographs of the luminometric responses of the solid-supported chemodosimeter 7 with various pesticides (8.0 ppm). | |
The spectrofluorimetric titrations revealed that, among the tested pesticides, only those containing an aliphatic mercapto functionality, such as phorate, induced a spectrofluorimetric response from 7. In contrast, pesticides with aromatic or conjugated mercapto functionalities, such as methomyl and fenthion, and with thiophosphates (P
S and P–S), including malathion, methyl parathion, cygon, monitor, phosdrin, DDVP, and naled, did not produce any signal changes. Interestingly, all tested pesticides did not produce any spectrofluorimetric changes of the cyano-bridged complex 8. The ΔG° values of complexes 7 and 8 were determined to be −20.9 kJ mol−1 and −22.4 kJ mol−1, respectively, whereas that of PtII(DMSO)(phorate)Cl2 is −21.9 kJ mol−1. These results suggest that the control of the bridging linkage in ReI–bridge–PtII-based BmDAEs can lead to the regulation of their chemodosimetric selectivity. Furthermore, a solid-supported chemodosimetric device based on complex 7, fabricated by blending the complex with Al2O3, had a detection limit toward phorate of 0.48 mg L−1 (Fig. 5b).
2.3.3 Varying the ligand component on the MA signal transducer unit in BmDAEs.
In addition to changing the receptor and the bridging unit of a BmDAE to achieve a specific chemodosimetric detection, varying the auxiliary ligand at the signal transducer results in selective detection. Three ReI–PtII-based BmDAEs,15h [ReI(diimine-ligand)(CO)3(CN)]–[PtII(DMSO)(Cl)2] [9 = 2,2′-biquinoline (biq), 10 = 5-phenyl-1,10-phenanthroline (5-ph-phen), and 11 = bpy] were synthesized for the detection of dimethyl sulfide (CH3SCH3), a biomarker used for testing the freshness of meats23 (Fig. 6). Complexes 9–11 are analogues of 8. The crystal structure of 9 reveals that the two metal centers in the complex adopt a linear configuration with one Pt(DMSO)Cl2 moiety bridged to the Re(I) center via cyano linkages.
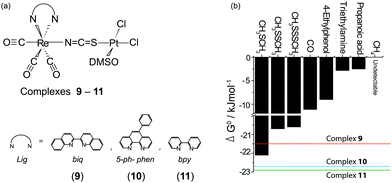 |
| Fig. 6 (a) Structures of complexes 9–11; (b) binding strengths (ΔG°) of the [PtII(DMSO)Cl2–analyte] adducts. The three horizontal lines represent the ΔG° values of complexes 9–11. | |
The spectrofluorimetric titrations revealed that dimethyl sulfide induced a spectrofluorimetric response from 9 but not from 10 or 11. Other biogenic molecules, including dimethyl disulfide (CH3SSCH3), dimethyl trisulfide (CH3SSSCH3), 4-ethylphenol, triethylamine, propanoic acid, methane, and carbon monoxide, did not yield any spectrofluorimetric changes from 9, 10, and 11. Among these three BmDAEs, 9 has the largest formation energy owing to the extensive π–π conjugation between the pyridine rings in biq (the ΔG° of the formation of 9, 10, and 11 are −21.5 kJ mol−1, −22.7 kJ mol−1, and −22.9 kJ mol−1, respectively), which also explains its specific chemodosimetric detection. Thus, the ΔG° value of 9 was smaller than those of all the PtII–analyte adducts except for PtII–CH3SCH3 (the ΔG° of its formation is −22.1 kJ mol−1), and 9 selectively responded to CH3SCH3 (Fig. 6).
2.3.4 Varying the MA signal transducer unit in BmDAEs.
A RuII–CuII-based BmDAE, [RuII(bpy)2(CN)2]–[CuII(dien)](ClO4)2 (12), was synthesized to study the effect of varying the signal transducer unit (MA) of BmDAE on the chemodosimetric selectivity.15c The main difference between complexes 12 and 5 is that the RuII–diimine donor in 5 possesses a net negative charge, while that of 12 is neutral. The donor–acceptor binding strength of 12 was expected to be weaker than that of 5. The ΔG° of 12 was only −14.8 kJ mol−1 which is larger than the [CuII(dien)–CN−/SO42−/HCO3−] adducts (−37.9 kJ mol−1, −26.6 kJ mol−1, and −21.2 kJ mol−1, respectively, Fig. 3b). Hence, the spectrofluorometric titrations of 12 with the relevant anions showed non-selective results. CN−, SO42−, and HCO3− were able to cleave this analogue and produce the luminescent responses. The weaker metallic donor (MA) in 12 is the reason for its loss of selectivity.
3. Indicator/catalyst-displacement assay (ICDA) for molecular detection and degradation
Supramolecular devices that not only provide highly sensitive and selective detection but can also quickly degrade and mineralize persistent pollutants are highly sought after. In this section, a novel disassembly approach using BmDAEs, the so-called indicator/catalyst-displacement assay (ICDA), that features chemodosimetric detection, signal amplifying, and catalytic degradation of pollutants will be discussed. The concept of the ICDA resembles that of the IDA described above. However, another functional pair (i.e., inhibitor/catalyst) coexists with the signal transducer (MA)/receptor (MB) pair of a BmDAE. In this system, the metallic receptor, MB, that also functions as a catalyst, first binds to the signal transducer, MA, which is also an inhibitor. Then, a competitive analyte is introduced, causing the displacement of the signal transducer/inhibitor from the receptor/catalyst, which activates both the signal transducer and the catalyst. Ultimately, the analyte can be catalytically degraded into harmless components in a one-step process while its concentration is monitored (Scheme 2). For analytes that are not pollutants and do not require degradation, the released catalyst can be used for dye-formation/-degradation to achieve a higher sensitivity of detection (i.e., signal amplification).
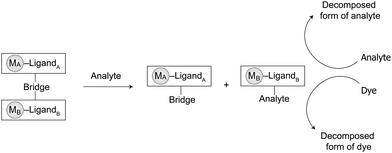 |
| Scheme 2 BmDAEs for the detection, degradation, and signal amplification of analytes through the ICDA. | |
3.1 Selective chemodosimetric detection using the ICDA
Two BmDAEs, [RuII(tBubpy)(CN)4]2–[FeIII(H2O)3Cl]2 (13)24 and {FeII(tBubpy)(CN)4–[CuII(dien)]2}(ClO4)2 (14),25 were synthesized for the detection of oxalic acid and cyanide, respectively, via an ICDA. Overuse of oxalic acid is common in rare-earth metal mining and causes freshwater pollution which can result in renal metabolic disorders.26
Complex 13 was formed by stirring equal equivalent molar of FeCl3 with K2[Ru(tBubpy)(CN)4] in deionized water. The tetranuclear structure was confirmed by ESI-MS and FTIR analysis, where the νC
N peaks of [RuII(tBubpy)(CN)4]2− at 2042 cm−1, 2058 cm−1, and 2093 cm−1 were shifted to 2030 cm−1, 2076 cm−1, and 2114 cm−1. Complex 13 was found to selectively detect oxalate in the presence of anions such as glyoxylate, pyruvate, tartrate, acetate, SO42−, HPO42−, NO3−, N3−, CN−, NCS−, and Br−. The selective spectroscopic and spectrofluorimetric signals were generated according to the abovementioned IDA mechanism and were attributed to the relative stability of 13 (ΔG° = −18.0 kJ mol−1) with respect to the FeIII–oxalate adducts (ΔG° = −20.1 kJ mol−1). Using UV-vis spectroscopy, the visual detection limit of 13 for oxalate was determined to be 100 mg L−1.
Complex 14 is an analogue of complex 5 in which the RuII luminophore is replaced by a FeII chromophore. Each FeII metallic center of 14 is cyano-bridged to two CuII receptors. The IR spectroscopic analysis showed that the νC
N peaks of [FeII(tBubpy)(CN)4]2− at 2085 cm−1, 2067 cm−1, and 2053 cm−1 were shifted to 2118 cm−1, 2095 cm−1, 2076 cm−1, and 2052 cm−1. The ΔG° of 14 is −27.0 kJ mol−1. Thus, the detection of cyanide by 14 proceeded via the same chemodosimetric mechanism as that by 5, and triggered a colorimetric signal (reddish-purple to light pink color change). The visual detection limit of 14 for cyanide ions was determined to be 32 mg L−1.
3.2 Degradation through the ICDA
The efficient degradation of persistent industrial pollutants is a major challenge. Therefore, the degradation properties of 13 and 14 for oxalate and cyanide, respectively, after their chemodosimetric detection have been investigated. The use of a FeIII species, which is released during the chemodosimetric detection of oxalate by 13, as a photo-Fenton catalyst for the degradation of pollutants was studied. The photo-degradation studies showed that the released FeIII–oxalate adduct is a good photo-Fenton's catalyst that can oxidize free oxalate left in the solution and achieve complete degradation of its organic content to CO2 within 6 h (Fig. 7). Oxalate degradation is achieved by hydroxyl radicals which are generated via the photo-Fenton mechanism from the released FeIII–oxalate adducts according to eqn (3).27 This degradation is related to the disassembly of 13 by the introduction of oxalate, causing FeIII catalyst activation by breaking the cyano-linkages from the inhibitor, [RuII(tBubpy)(CN)4]2−. Because common anions, such as glyoxylate, pyruvate, tartrate, acetate, SO42−, HPO42−, NO3−, N3−, CN−, NCS−, and Br− could not dissociate the BmDAE, complex 13 remained intact and the cyano-bridged FeIII catalyst remained inactive in the presence of potential interferents. | Fe3+ + H2O + hν → Fe2+ + HO˙ + H+ | (3) |
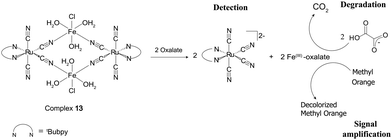 |
| Fig. 7 The mechanism of oxalate detection by complex 13via an ICDA, involving detection, degradation, and signal amplification. | |
To further demonstrate the scope of the ICDA, the catalytic oxidation of cyanide by [CuII(dien)(CN)2] adducts released from the chemodosimetric detection by complex 14 was investigated. Upon addition of cyanide to the ensemble, the displacement of [CuII(dien)]2+ from [FeII(tBubpy)(CN)4]2− afforded the [CuII(dien)(CN)2] adduct. Subsequently, the latter catalyzed the oxidation of free cyanide ions to the much less toxic cyanate using H2O2 as the oxidant within 240 min according to eqn (4) (Fig. 8). From the above results, we can note that the manipulation of the disassembly and assembly forms of the signal transducers of complexes 13 and 14 is the key for effective detection, while the disassembly and assembly of the BmDAE receptors enable tuning of the degradation properties.
| CN− + H2O2 → OCN− + H2O | (4) |
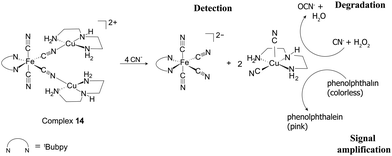 |
| Fig. 8 The mechanism of cyanide detection by complex 14via an ICDA, involving detection, degradation, and signal amplification. | |
3.3 Signal amplification through the ICDA
The detection of pollutants and analytes in low concentrations is essential for environmental monitoring. Although the increasing sophistication of analytical instruments leads to a decrease in the detection limits of many analytes, ultra-sensitive supramolecular detectors have been recently developed. Some chemical systems that can amplify weak input signals have been recently reported by Anslyn,9a,28 Prins,29 and others.30 However, smart molecular devices that can amplify reporting signals and simultaneously degrade pollutants via simple chemical design are rare. Using methyl orange as an additional coloring agent, the signal amplification property of complex 13 in the detection of oxalate via the ICDA mechanism was demonstrated. Upon addition of oxalate to a solution of 13 and methyl orange, hydroxyl radicals generated from the aforementioned FeIII–oxalate adduct-catalyzed photo-Fenton reaction not only mineralized the pollutant, but also led to decomposition of the added methyl orange, leading to a decrease in the intensity of the peak at 510 nm. This caused a sharp color change with a visual detection limit as low as 2 mg L−1 (Fig. 7). Therefore, the sensitivity was amplified approximately 50-fold, from 100 to 2 mg L−1, through this catalytic amplification process.
Using phenolphthalein as the dye, complex 14 could also amplify the colorimetric cyanide detection signal 80-fold via the catalytic amplification (Fig. 8). It was found that, through the ICDA mechanism (Section 3.2), the released [CuII(dien)(CN)2] adduct acted as a catalyst for both the degradation of cyanide and the oxidation of phenolphthalein to phenolphthalein. Therefore, a pink color developed (abs = 551 nm), resulting in a sharply magnified color change. The visual detection limit for the cyanide ion was as low as 0.4 mg L−1 due to the amplification, as opposed to 32 mg L−1 without the indicator.
3.4 Changing the irreversible chemodosimetric detection to reversible chemosensing detection through ICDA
Chemosensors differ from chemodosimeters in that they allow in-situ and reversible detection of analytes.13 A possible solution for the development of real-time and reversible sensing applications of BmDAEs consists in the use of ICDAs. Via the ICDA mechanism, not only the free analyte can be destroyed, but also the bound analyte from the receptor-analyte adduct can be degraded, thus releasing the free receptor into the matrix, and leading to the assembly of the original BmDAE.
A bimetallic complex, (CN)3FeII(tppz)CuIICl (15, where tppz = 2,3,5,6-tetra(2′-pyridyl)pyrazine), including a FeII–diimine MLCT indicator and a bifunctional CuII catalyst/receptor was synthesized to demonstrate the reversible detection and detoxification of cyanide via the ICDA mechanism.31a Complex 15, which features a bridging tppz ligand, was synthesized by stirring equimo amounts of [benzyltriphenylphosphonium]-[FeII(tppz)(CN)3] and CuIICl2 in an aqueous ethanolic medium, and its structure is shown in Fig. 9a.
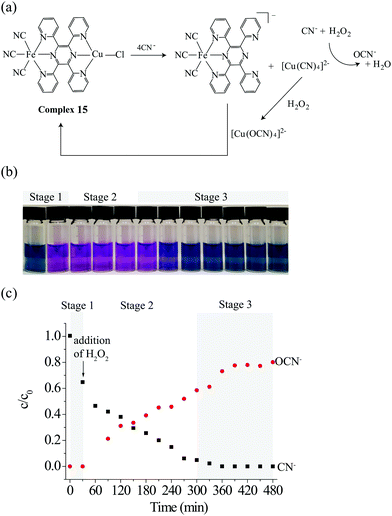 |
| Fig. 9 Reversible detection of cyanide by 15via the degradation of newly formed “receptor–cyanide ensemble”, i.e. [CuII(CN)4]2−, under the ICDA. | |
Complex 15 produced a selective colorimetric response from blue to purple in the presence of cyanide, without interference by other common anions (CH3COO−, N3−, HCO3−, Cl−, NO3−, HPO42−, SO42−, SCN−, and OCN−). The colorimetric signal results from the dissociation of 15 (ΔG°= −16.3 kJ mol−1) into [FeII(tppz)(CN)3]− with concomitant formation of a more stable [CuII(CN)4]2− adduct (ΔG° = −125.5 kJ mol−1).31bFig. 9b (stage 1) shows the color changes in the test solution containing CN−, H2O2, and 15 (molar ratio of 10
:
20
:
1) from 0 to 30 min. Upon addition of cyanide, the color of the solution changes from blue (15) to purple ([FeII(tppz)(CN)3]− and [CuII(CN)4]2−). Correspondingly, the portion of CN− decreased by 40% due to the formation of [CuII(CN)4]2− (Fig. 9c, stage 1).
Fig. 9c (stage 2) shows that the formed [CuII(CN)4]2− is the active catalyst of the oxidative degradation of free CN− to OCN− in the presence of H2O2, and no UV-Vis spectroscopic changes for the test solution were observed (Fig. 9b, stage 2). Interestingly, the purple-colored [FeII(tppz)(CN)3]− solution gradually turned back to the original blue color during stage 3 (300–480 min, Fig. 9b). We found that during the third stage, the bound cyanide ligands in [CuII(CN)4]2− were further catalytically oxidized, in agreement with the increase in the portion of OCN− (Fig. 9c, stage 3). As the [CuII(NCO)4]2− adduct (ΔG° = −14.1 kJ mol−1) is less stable than complex 15, regeneration of the BmDAE was favored.
3.5 Fabrication of nano-materials for the detection and degradation of pollutants by the ICDA
Recently, three Prussian blue bimetallic analogs (PBAs) namely FeIII4[FeII(CN)6]3-, FeIII4[RuII(CN)6]3-, and FeIII4[OsII(CN)6]3-modified TiO2 materials (16–18, respectively) were fabricated and employed as bifunctional materials for the simultaneous detection and degradation of cyanide using the ICDA mechanism.32 The materials (16–18) were synthesized by stirring K4[FeII(CN)6], K4[RuII(CN)6], and K4[OSII(CN)6], respectively, with 20 molar equivalents of TiO2, followed by the addition of 1.33 molar equivalents of FeIIICl3. The sizes of 16–18 were determined to be 28 ± 2 nm, 27 ± 2 nm, and 27 ± 2 nm, respectively. These PBAs not only acted as ‘colored coats’ (16: blue, 17: purple, and 18: purple) but also quenched the photocatalytic properties of TiO2 (eqn (5)–(8)) by facilitating electron and hole recombination (eqn (9)–(11)) due to their metal-to-metal–ligand charge transfer (MMCT) properties.
Upon exposure to cyanide, the disassembly of FeIII from the TiO2 on the PBAs resulted in release of a new FeIII–cyanide adduct, which consequently interrupted the original MMCT of the PBA and restored the photocatalytic properties of TiO2 (Fig. 10). Among the tested organic and inorganic pollutants (e.g. cyanide, aniline, 1,5-naphthalenedisulfonic acid, benzoic acid, and 4-nitrophenol), only cyanide induced a selective colorimetric response (from a colored suspension to a white suspension), and triggered the photocatalytic activity of TiO2. Cyanide ions were subsequently catalytically photo-oxidized into the less toxic compounds cyanate, nitrite, nitrate, and CO2 by the released TiO2–[MIIA(CN)6]4− material. The method detection limit (MDL) values of 16–18 for CN− were 1.10 mg L−1, 0.60 mg L−1, and 0.83 mg L−1, respectively. The degradation efficiencies for 16–18 were found in the range 96.6–98.8% for CN−, while for the other analytes the corresponding values ranged from <0.2 to 6.5%. The detection and degradation of cyanide by 16–18 were also found to be applicable in tap and river water.
| TiO2 + hν → e− + hole+ | (5) |
| 0.5O2˙− + 0.5O2˙− + H+ → 0.5H2O2 + 0.5O2 | (7) |
| e− + hole+ → recombination | (9) |
| FeIII4[MIIA(CN)6]3 + e− → FeII4[MIIA(CN)6]3 | (10) |
| FeII4[MIIA(CN)6]3 + hole+ → FeIII4[MIIA(CN)6]3 | (11) |
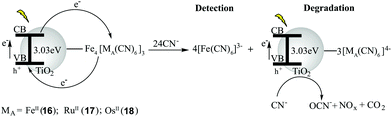 |
| Fig. 10 The detection and degradation of CN− by 16–18via the ICDA. | |
4. Catalyst/indicator-displacement assay (CIDA) for molecular detection and degradation
As mentioned in Section 2, enhanced selectivity is an important property in molecular detection. Efforts toward the increase of the chemodosimetric selectivity by modification of receptors, signal transducers, bridges, and auxiliary ligands of signal transducers of BmDAEs have been discussed in Sections 2.2–2.3. In addition, the use of the catalytic function of BmDAEs is another approach. Typically, a catalyst is tailored to promote a specific transformation. Therefore, by integrating an active catalyst into a BmDAE (in contrast to the ICDA, in the present case no inhibitor is incorporated into it), a target analyte can be initially transformed into a compound with specific functionality that can be more easily recognized via the IDA mechanism. Accordingly, the selectivity of the chemosensing system is expected to increase through, this so-called, catalyst/indicator-displacement assay (Scheme 3).
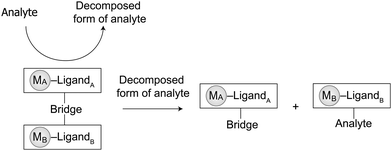 |
| Scheme 3 BmDAEs for the degradation and detection of analytes through the CIDA. | |
A novel RuII–CuI-based BmDAE, [RuII(bpy)2(CN)2]2–(CuII)2 (19) comprised of two active catalytic units (CuI-catalyst) and two signal transducer units (RuII(bpy)2(CN)2), was synthesized for the detection of methyl parathion, a highly toxic organophosphate pesticide via the CIDA.33 Complex 19 was synthesized by stirring equimolar amounts of CuII and Ru(bpy)2(CN)2 in a methanol/acetonitrile mixture (1
:
10 v/v). The CuI metallic receptor was employed because of its strong coordination to the phosphate ester group,34 and its function as a luminescent quencher for the 3MLCT of RuII(bpy)(CN)2. The crystal structure of 19 revealed its tetranuclear rhomboid configuration in which the two RuII centers are cyano-bridged to the two CuII centers.
Unlike IDA and ICDA, the phosphate ester group in methyl parathion cannot dissociate the BmDAE 19 due to its weak nucleophilicity. Interestingly, the active CuI catalytic unit in 19 catalytically hydrolyzed the phosphate ester of methyl parathion into the UV/vis-active 4-nitrophenolate and the o,o-dimethyl thiophosphate (DTP) anions, the latter of which is a strong nucleophile. Because the ΔG° of the CuI(DTP) adduct formation (−20.3 kJ mol−1) is smaller than that of 19 (−20.2 kJ mol−1), the DTP interacted with the CuI center in the bimetallic complex, displacing RuII(bpy)2(CN)2 and inducing a luminescence enhancement (Fig. 11). In contrast, as the active CuI catalytic units of 19 cannot hydrolyze other aromatic and aliphatic organophosphate pesticides, including fenthion, mevinphos, terbufos, and phosalone, a luminescent response is not observed. Owing to the hydrolysis of methyl parathion via the CIDA, the pesticide toxicity was also reduced. The detection limit of complex 19 toward methyl parathion was determined to be 8 mg L−1.
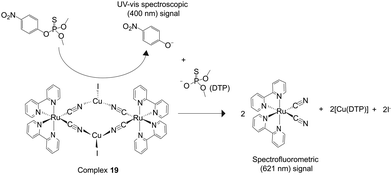 |
| Fig. 11 The CIDA mechanism for the detection of methyl parathion by complex 19. | |
5. Catalyst-displacement assay (CDA) for molecular degradation
The development of “smart” catalysts is crucial in both synthetic and applied chemistry. Traditionally, chemists develop catalysts to improve the yields, rates, and/or enantioselectivity of the desired transformation. However, unlike traditional catalysts that initiate a reaction only in the presence of reactants, “smart” catalysts can be developed by incorporating extra control parameters, including condition-selective receptors, thus enabling activity only under specific conditions. Recently, this type of smart catalyst, so-called latent catalysts, have demonstrated “off–on” functionality under variable temperature conditions,35 the addition of a chemical agent,36 light irradiation,37 or mechanical forces.7b Although these result in interesting reaction outcomes, such latent catalysts have exhibited considerable constraints in terms of their molecular synthesis and monotonic on–off switching function. In this section, a new latent catalytic design composed of a metallic inhibitor and a metallic pre-catalyst, referred to the so-called catalyst-displacement assay (CDA), will be discussed. The use of BmDAE aims to relieve synthetic organic constraints during the synthesis of catalysts, while the CDA design not only renders them off–on latent catalysts, but also acts as a threshold switch for catalysis initiation once a reagent reaches a specific concentration (Scheme 4).
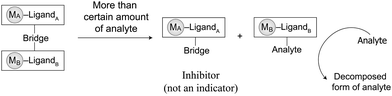 |
| Scheme 4 BmDAEs for the “threshold-controlled off–on” degradation of analytes through the CDA. | |
As mentioned in Sections 3.1–3.3, complex 14 was synthesized for the detection and degradation of cyanide via the ICDA (Fig. 8). The addition of cyanide to the ensemble initially led to dissociation of [CuII(dien)]2+ from the colored [FeII(tBubpy)(CN)4]2− unit, producing the [CuII(dien)(CN)2] adduct which was then used for degradation. In order to understand the CDA, a new FeII–CuII-based BmDAE, FeII(CN)6–[CuII(dien)(H2O)]2 (20), in which the ferrocyanide unit can only provide its inhibiting functionality but not a signal-transducing function, was synthesized.38 Complex 20 is a stable BmDAE owing to the four negative charges in its ferrocyanide donating unit (ΔG° = −30.4 kJ mol−1). The [FeII(CN)6]4− unit in 20 uses two of its cyano-units to bridge with two CuII receptors, as verified by ESI-MS and FTIR.
Upon addition of cyanide to 20, the displacement of [CuII(dien)]2+ from [FeII(CN)6]4− took place, and formation of the [CuII(dien)(CN)2] adduct with a ΔG° of −37.9 kJ mol−1 occurred. Interestingly, complex 20 did not exhibit catalytic properties for cyanide concentrations below 200 μM (Fig. 12). To understand this observation, the cleavage rate constant for the cyano-bridges by free cyanide was studied and found to be 18.8 M−1 s−1. This result shows that, despite the favorable thermodynamics of dissociation of 20 by cyanide with concomitant formation of the more stable [CuII(dien)(CN)2] adduct, the bridge cleavage by cyanide is limited by a kinetic factor. Therefore, the cleavage of 20 is triggered once the initial cyanide concentration exceeds 200 μM, subsequently releasing [CuII(dien)(CN)2] for further catalytic cyanide oxidation. Through the CDA, the BmDAEs functioned as “threshold-controlled” latent catalysts.
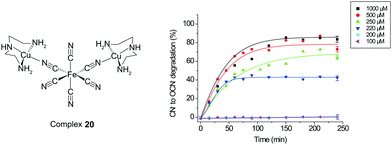 |
| Fig. 12 The “threshold-controlled” latent catalyst 20 operates via the CDA mechanism to degrade cyanide. | |
Finally, through the BmDAE based CDA, the new, less strongly bound BmDAE {[FeII(tBubpy)2(CN)2]2–[CuII(dien)]}Cl2 (21, ΔG° = −15.1 kJ mol−1 with cleavage rate constant of 58.3 M−1 s−1) which is the analogue of 12, was synthesized. With regard to its structure, the CuII receptor is cyano-bridged with two neutral FeII(tBubpy)2(CN)2 units. In this BmDAE, the catalytic oxidation of cyanide requires a threshold concentration of 0.50 mg L−1, which is the suggested maximum allowed level of cyanide in wastewater by the European International organization (Fig. 13).39
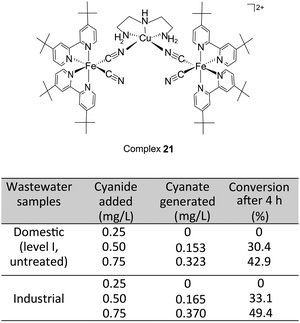 |
| Fig. 13 Results of cyanide degradation by 21 in domestic (level I, untreated) and industrial wastewater samples. | |
In further studies, both domestic and industrial real wastewater samples were collected and spiked with cyanide to achieve concentrations of 0.25 mg L−1, 0.50 mg L−1, or 0.75 mg L−1. The degradation/oxidation of cyanide in these samples was analyzed at 298 K with complex 21 in the presence of H2O2. The results revealed that the oxidation of CN− was initiated from 0.50 mg L−1, resulting in 30.4–33.1% OCN− content (Fig. 13). However, in samples with a below-threshold cyanide concentration of 0.25 mg L−1, neither the oxidation of CN− nor the formation of OCN− were detected throughout the duration of the test. The overall results indicate that the CDA concept is applicable to real wastewater samples, even in the presence of organic and inorganic residues.
6. Conclusion
The disassembly is an important process in supramolecular chemistry, through which numerous catalysts and molecular detectors have been developed. This review discussed the use of BmDAEs as the supramolecular systems to study the disassembly between metallic donors and acceptors. Four different displacement assays, IDA, ICDA, CIDA, and CDA, have been developed with the incorporation of signal transducers, receptors, inhibitors, catalysts, and pre-catalysts in the donor/acceptor pair of BmDAEs for pollutant degradation and detection. Importantly, the control of supramolecular dissociation sequence is the key to these systems. Moving forward, the exchange of molecular units must be further studied to demonstrate the adaptability and evolution of supramolecular systems in response to environmental changes. Reversibility and dynamics are important characteristics that should be taken into account for the future development of related functional materials.
Conflicts of interest
There are no conflicts to declare.
Acknowledgements
CF is grateful for the Croucher Fellowship (2005–2007) from The Croucher Foundation and guidance from Prof Jean Marie Lehn during his post-doc training. The authors would also like to thank The Education University of Hong Kong and the Research Grants Council of Hong Kong SAR, China for financial support.
References
-
(a) J. M. Lehn, Toward self-organization and complex matter, Science, 2002, 295, 2400–2403 CrossRef CAS PubMed;
(b)
J. M. Lehn, in Supramolecular science: where it is and where it is going, ed. R. Ungaro and E. Dalcanale, Kluwer, Dordrecht, 1999, pp. 287–304 Search PubMed.
- C. Bohne, Supramolecular dynamics, Chem. Soc. Rev., 2014, 43, 4037–4050 RSC.
-
(a) S. Wang, W. Gao, X.-Y. Hu, Y.-Z. Shen and L. Wang, Supramolecular strategy for smart windows, Chem. Commun., 2019, 55, 4137–4149 RSC;
(b) M. Raynal, P. Ballester, A. Vidal-Ferran and P. W. N. M. van Leeuwen, Supramolecular catalysis. Part 1: non-covalent interactions as a tool for building and modifying homogeneous catalysts, Chem. Soc. Rev., 2014, 43, 1660–1733 RSC;
(c) I. V. Kolesnichenko and E. V. Anslyn, Practical applications of supramolecular chemistry, Chem. Soc. Rev., 2017, 46, 2385–2390 RSC;
(d) Z. Feng, T. Zhang, H. Wang and B. Xu, Supramolecular catalysis and dynamic assemblies for medicine, Chem. Soc. Rev., 2017, 46, 6470–6479 RSC;
(e) J. W. Fredy, A. Méndez-Ardoy, S. Kwangmettatam, D. Bochicchio, B. Matt, M. C. A. Stuart, J. Huskens, N. Katsonis, G. M. Pavan and T. Kudernac, Molecular photoswitches mediating the strain-driven disassembly of supramolecular tubules, Proc. Natl. Acad. Sci. U. S. A., 2017, 114, 11850–11855 CrossRef CAS.
-
(a) N. Hafezi and J. M. Lehn, Adaptation of dynamic covalent systems of imine constituents to medium change by component redistribution under reversible phase separation, J. Am. Chem. Soc., 2012, 134, 12861–12868 CrossRef CAS;
(b) V. Berl, I. Huc, R. G. Khoury, M. J. Krische and J. M. Lehn, Interconversion of single and double helices formed from synthetic molecular strands, Nature, 2000, 407, 720–723 CrossRef CAS PubMed.
- T. D. Pollard and J. A. Cooper, Actin, a central player in cell shape and movement, Science, 2009, 326, 1208–1212 CrossRef CAS PubMed.
-
(a) Y.-F. Li, Y.-M. Sun, R. C. Beier, H.-T. Lei, S. Gee, B. D. Hammock, H. Wang, Z. Wang, X. Sun, Y.-D. Shen and Z.-L. Xu, Immunochemical techniques for multianalyte analysis of chemical residues in food and the environment: A review, TrAC, Trends Anal. Chem., 2017, 88, 25–40 CrossRef CAS;
(b) S. Y. Toh, M. Citartan, S. C. B. Gopinath and T.-H. Tang, Aptamers as a replacement for antibodies in enzyme-linked immunosorbent assay, Biosens. Bioelectron., 2015, 64, 392–403 CrossRef CAS PubMed;
(c) S. L. Wiskur, H. Ait-Haddou, J. J. Lavigne and E. V. Anslyn, Teaching old indicators new tricks, Acc. Chem. Res., 2001, 34, 963–972 CrossRef CAS PubMed.
-
(a) S. Monsaert, A. L. Vila, R. Drozdzak, P. Van der Voort and F. Verpoort, Latent olefin metathesis catalysts, Chem. Soc. Rev., 2009, 38, 3360–3372 RSC;
(b) A. Piermattei, S. Karthikeyan and R. P. Sijbesma, Activating catalysts with mechanical force, Nat. Chem., 2009, 1, 133 CrossRef CAS.
-
(a) E. J. O’Neil and B. D. Smith, Anion recognition using dimetallic coordination complexes, Coord. Chem. Rev., 2006, 250, 3068–3080 CrossRef;
(b) N. Wheatley and P. Kalck, Structure and reactivity of early−late heterobimetallic complexes, Chem. Rev., 1999, 99, 3379 CrossRef CAS PubMed;
(c) E. L. Dias and R. H. Grubbs, Synthesis and investigation of homo-and heterobimetallic ruthenium olefin metathesis catalysts exhibiting increased activities, Organometallics, 1998, 17, 2758 CrossRef CAS;
(d) C. F. Chow, S. Fujii and J. M. Lehn, Metallodynamers: neutral dynamic metallosupramolecular
polymers displaying transformation of mechanical and optical properties on constitutional exchange, Angew. Chem., Int. Ed., 2007, 46, 5007 CrossRef CAS PubMed;
(e) C. F. Chow, S. Fujii and J. M. Lehn, Metallodynamers: Neutral Double-Dynamic Metallosupramolecular Polymers, Chem. – Asian J., 2008, 3, 1324 CrossRef CAS PubMed;
(f) M. Shibasaki, H. Sasai and T. Arai, Asymmetric catalysis with heterobimetallic compounds, Angew. Chem., Int. Ed. Engl., 1997, 36, 1237 CrossRef CAS;
(g) C. J. Shorrock, B. Y. Xue, P. B. Kim, R. J. Batchelor, B. O. Patrick and D. B. Leznoff, Heterobimetallic Coordination Polymers Incorporating [M(CN)2]− (M= Cu, Ag) and [Ag2(CN)3]− Units: Increasing Structural Dimensionality via M−M’ and M⋯NC Interactions, Inorg. Chem., 2002, 41, 6743 CrossRef CAS PubMed.
-
(a) L. Zhu and E. V. Anslyn, Signal amplification by allosteric catalysis, Angew. Chem., Int. Ed., 2006, 45, 1190–1196 CrossRef CAS PubMed;
(b) J. M. Lehn, Perspectives in chemistry—aspects of adaptive chemistry and materials, Angew. Chem., Int. Ed., 2015, 54, 3276–3289 CrossRef CAS PubMed.
- I. V. Kolesnichenko and E. V. Anslyn, Practical applications of supramolecular chemistry, Chem. Soc. Rev., 2017, 46, 2385–2390 RSC.
-
(a) F. Y. Thanzeel, A. Sripada and C. Wolf, Quantitative Chiroptical Sensing of Free Amino Acids, Biothiols, Amines, and Amino Alcohols with an Aryl Fluoride Probe, J. Am. Chem. Soc., 2019, 141, 16382–16387 CrossRef PubMed;
(b) X. Liu, D. G. Smith and K. A. Jolliffe, Are two better than one? Comparing intermolecular and intramolecular indicator displacement assays in pyrophosphate sensors, Chem. Commun., 2016, 52, 8463–8466 RSC;
(c) Y. Sasaki, É. Leclerc, V. Hamedpour, R. Kubota, S. Takizawa, Y. Sakai and T. Minami, Simplest Chemosensor Array for Phosphorylated Saccharides, Anal. Chem., 2019, 91, 15570–15576 CrossRef CAS PubMed.
-
(a) S. H. Kim, H. S. Choi, J. Kim, S. J. Lee, D. T. Quang and J. S. Kim, Novel optical/electrochemical selective 1,2,3-triazole ring-appended chemosensor for the Al3+ ion, Org. Lett., 2010, 12, 560–563 CrossRef CAS PubMed;
(b) R. Kaushik, R. Sakla, A. Ghosh, S. Dama, A. Mittal and D. A. Jose, Copper Complex-Embedded Vesicular Receptor for Selective Detection of Cyanide Ion and Colorimetric Monitoring of Enzymatic Reaction, ACS Appl. Mater. Interfaces, 2019, 11, 47587–47595 CrossRef CAS PubMed;
(c) M. A. Beatty, A. J. Selinger, Y. Q. Li and F. Hof, Parallel Synthesis and Screening of Supramolecular Chemosensors that Achieve Fluorescent Turn-On Detection of Drugs in Saliva, J. Am. Chem. Soc., 2019, 141, 16763–16771 CrossRef CAS PubMed.
-
A. W. Czarnik, Fluorescent chemosensors of ion and molecule recognition, ACS Press, Washington, D. C., 1992 Search PubMed.
-
(a) M. A. Hortala, L. Fabbrizzi, N. Marcotte, F. Stomeó and A. Taglietti, Designing the selectivity of the fluorescent detection of amino acids: a chemosensing ensemble for histidine, J. Am. Chem. Soc., 2003, 125, 20 CrossRef CAS PubMed;
(b) L.-X. Huang, Q. Guo, Y. Chen, P. Verwilst, S. Son, J.-B. Wu, Q.-Y. Cao and J. S. Kim, Nanomolar detection of adenosine triphosphate (ATP) using a nanostructured fluorescent chemosensing ensemble, Chem. Commun., 2019, 55, 14135–14138 RSC;
(c) D. Jimenez, R. Martinez-Manez, F. Sancenon, J. V. RosLis, A. Benito and J. Soto, A new chromo-chemodosimeter selective for sulfide anion, J. Am. Chem. Soc., 2003, 125, 9000 CrossRef CAS PubMed;
(d) M. Comes, G. Rodriguez-Lopez, M. D. Marcos, R. Martinez-Manez, F. Sancenon, J. Soto, L. A. Villaescusa, P. Amoros and D. Beltran, Host Solids Containing Nanoscale Anion-Binding Pockets and Their Use in Selective Sensing Displacement Assays, Angew. Chem., Int. Ed., 2005, 44, 2918 CrossRef CAS PubMed;
(e) Y. Sasaki, Z. Zhang and T. Minami, A Saccharide Chemosensor Array Developed Based on an Indicator Displacement Assay Using a Combination of Commercially Available Reagents, Front. Chem., 2019, 7, 49 CrossRef CAS PubMed.
-
(a) C. F. Chow, B. K. W. Chiu, M. H. W. Lam and W. Y. Wong, A trinuclear heterobimetallic Ru(II)/Pt(II) complex as a chemodosimeter selective for sulfhydryl-containing amino acids and peptides, J. Am. Chem. Soc., 2003, 125, 7802–7803 CrossRef CAS PubMed;
(b) C. F. Chow, M. H. W. Lam and W. Y. Wong, Design and synthesis of heterobimetallic donor–acceptor chemodosimetric ensembles for the detection of sulfhydryl-containing amino acids and peptides, Dalton Trans., 2005, 475–484 RSC;
(c) C. F. Chow, M. H. W. Lam and W. Y. Wong, A heterobimetallic ruthenium(II)−copper(II) donor−acceptor complex as a chemodosimetric ensemble for selective cyanide detection, Inorg. Chem., 2004, 43, 8387 CrossRef CAS PubMed;
(d) C. K. Koo, C. F. Chow, B. K. W. Chiu, M. H. W. Lam and W. Y. Wong, A Pair of Coordination Donor–Acceptor Ensembles for the Detection of Tartrate in Aqueous Media, Eur. J. Inorg. Chem., 2008, 1318 CrossRef CAS;
(e) C. F. Chow, H. K. Kong, S. W. Leung, B. K. W. Chiu, C. K. Koo, E. N. Y. Lei, M. H. W. Lam, W. T. Wong and W. Y. Wong, Heterobimetallic Ru(II)−Eu(III) complex as chemodosimeter for selective biogenic amine odorants detection in fish sample, Anal. Chem., 2011, 83, 289 CrossRef CAS PubMed;
(f) C. F. Chow, M. H. W. Lam and W. Y. Wong, Design and synthesis of heterobimetallic Ru(II)–Ln(III) complexes as chemodosimetric ensembles for the detection of biogenic amine odorants, Anal. Chem., 2013, 85, 8246–8253 CrossRef CAS PubMed;
(g) C. F. Chow, K. Y. F. Ho and C. B. Gong, Synthesis of a new bimetallic Re(I)–NCS–Pt(II) complex as chemodosimetric ensemble for the selective detection of mercapto-containing pesticides, Anal. Chem., 2015, 87, 6112–6118 CrossRef CAS PubMed;
(h) C. F. Chow, F. W. Gong and C. B. Gong, Chemodosimetric analysis in food-safety monitoring: design, synthesis, and application of a bimetallic Re(I)–Pt(II) complex for detection of dimethyl sulfide in foods, Analyst, 2014, 139, 4532–4537 RSC;
(i) C. F. Chow, P. Y. Ho, Y. J. Lu, W. L. Wong, Q. Tang and C. B. Gong, Development of sensitive and selective food sensors using new Re(I)–Pt(II) bimetallic complexes to detect volatile biogenic sulfides formed by meat spoilage, Food Chem., 2017, 216, 382–389 CrossRef CAS PubMed;
(j) C. F. Chow, S. Liu, C. W. Chan, Y. J. Lu, W. L. Wong, Q. Tang and C. B. Gong, A bimetallic Re(I)-NCS-Pt(II) solid-support chemosensor for the selective detection of dimethyl sulfide in spoiled meat, Sens. Actuators, B, 2018, 255, 2298–2305 CrossRef CAS.
-
(a)
M. E. Howe-Grant and S. J. Lippard, in Metal Ions in Biological Systems, ed. H. Siegel, Dekker, New York, 1980, vol. 11, pp. 63–125 Search PubMed;
(b) S. Swavey, Z. Fang and K. J. Brewer, Mixed-metal supramolecular complexes coupling phosphine-containing Ru(II) light absorbers to a reactive Pt(II) through polyazine, bridging ligands, Inorg. Chem., 2002, 41, 2598 CrossRef CAS PubMed;
(c) C. A. Bignozzi and F. Scandola, Cyano-bridged ruthenium(II)/platinum(II) complexes: synthesis, photophysical properties, and excited-state redox behaviour, Inorg. Chem., 1984, 23, 1540 CrossRef CAS;
(d) N. Kitamura, M. Sato, H. B. Kim, R. Obata and S. Tazuke, Solvatochromism in the excited state of the cis-dicyanobis (1,10-phenanthroline)ruthenium(II) complex, Inorg. Chem., 1988, 27, 651 CrossRef CAS.
- A. Hubaux and G. Vos, Decision and detection limits for calibration curves, Anal. Chem., 1970, 42, 849–855 CrossRef CAS.
-
J. L. Sessler, P. A. Gale and W. S. Cho, Anion Receptor Chemistry, RSC Press, London, 2006, ch. 7, pp. 63–125 Search PubMed.
-
(a)
G. C. Miller and C. A. Pritsos, Cyanide: Soc., Ind. Econ.
Aspects, Proc. Symp. Annu. Meet. TMS, 2001, 73;
(b) D. Shan, C. Mousty and S. Cosnier, Subnanomolar cyanide detection at polyphenol oxidase/clay biosensors, Anal. Chem., 2004, 76, 178 CrossRef CAS PubMed;
(c) C. O. Ikediobi, L. Wen and L. M. Latinwo, Pesticide analysis by solid-phase microextraction, Am. Environ. Lab., 1997, 9, 20 Search PubMed;
(d)
E. Gail, S. Gos, R. Kulzer, J. Lorösch, A. Rubo, M. Sauer, R. Kellens, J. Reddy, N. Steier and W. Hasenpusch, Cyano Compounds, Inorganic, in Ullmann's Encyclopedia of Industrial Chemistry, Wiley-VCH Verlag GmbH & Co. KGaA, Weinheim, Germany, 2011 Search PubMed;
(e) X.-X. Ou, Y.-L. Jin, X.-Q. Chen, C. B. Gong, X.-B. Ma, Y.-S. Wang, C. F. Chow and Q. Tang, Colorimetric test paper for cyanide ion determination in real-time, Anal. Methods, 2015, 7, 5239–5244 RSC.
-
(a) Z. Xu, X. Chen, H. N. Kim and J. Yoon, Sensors for the optical detection of cyanide ion, Chem. Soc. Rev., 2010, 39, 127–137 RSC;
(b) F. Wang, L. Wang, X. Chen and J. Yoon, Recent progress in the development of fluorometric and colorimetric chemosensors for detection of cyanide ions, Chem. Soc. Rev., 2014, 43, 4312–4324 RSC.
-
(a) S. L. Taylor, Histamine food poisoning: toxicology and clinical aspects, CRC Crit. Rev. Toxicol., 1986, 17, 91 CrossRef CAS PubMed;
(b) J. D. Morrow, G. R. Margolies, J. Rowland and L. J. N. Roberts, Evidence that histamine is the causative toxin of scombroid-fish poisoning, Engl. J. Med., 1991, 324, 716 CrossRef CAS PubMed;
(c) R. M. Wachter and G. S. Kaveh, The unintended consequences of measuring quality on the quality of medical care, Engl. J. Med., 2000, 342, 520 CrossRef CAS PubMed;
(d) M. A. Banchaabouchi, B. Marescau, R. D’Hooge, S. Engelborgh and P. P. De Deyn, Consequences of renal mass reduction on amino acid and biogenic amine levels in nephrectomized mice, Amino Acids, 2000, 18, 265 CrossRef CAS PubMed;
(e) Decomposition & Histamine in Albacore, Skipjack, and Yellowfin Tuna, FDA/ORA Compliance Policy Guide, 2004 revision, Subchapter 540.525; FDA: Washington, DC, 2004.
-
(a) H. Zhou, L. Baldini, J. Hong, A. J. Wilson and A. D. Hamilton, Pattern recognition of proteins based on an array of functionalized porphyrins, J. Am. Chem. Soc., 2006, 128, 2421–2425 CrossRef CAS PubMed;
(b) N. A. Rakow, A. Sen, M. C. Janzen, J. B. Ponder and K. S. Suslick, Molecular recognition and discrimination of amines with a colorimetric array, Angew. Chem., Int. Ed., 2005, 44, 4528–4532 CrossRef CAS PubMed;
(c) G. A. Sotzing, J. N. Phend, R. H. Grubbs and N. S. Lewis, Highly sensitive detection and discrimination of biogenic amines utilizing arrays of polyaniline/carbon black composite vapor detectors, Chem. Mater., 2000, 12, 593–595 CrossRef CAS.
-
(a) R. T. Marsili, SPME-MS-MVA as an Electronic Nose for the Study of Off-Flavors in Milk, J. Agric. Food Chem., 1999, 47, 648 CrossRef CAS;
(b) V. Varlet and X. Fernandez, Sulfur-containing volatile compounds in seafood: occurrence, odorant properties and mechanisms of formation, Food Sci. Technol. Int., 2010, 16, 463 CrossRef CAS PubMed;
(c) A. Isogal, R. Kanda, Y. Hiraga, T. Nishimura, H. Iwata and N. Goto-Yamamoto, Screening and identification of precursor compounds of dimethyl trisulfide (DMTS) in Japanese sake, J. Agric. Food Chem., 2009, 57, 189 CrossRef PubMed;
(d) T. M. Lovestead and T. J. Bruno, Detection of poultry spoilage markers from headspace analysis with cryoadsorption on a short alumina PLOT column, Food Chem., 2010, 121, 1274 CrossRef CAS;
(e) Scientifc Opinion on Flavouring Group Evaluation 74, Revision 1 (FGE.74Rev1), 2009.
- C. F. Chow, P. Y. Ho and C. B. Gong, An Ru(II)–Fe(III) bimetallic complex as a multifunctional device for detecting, signal amplifying, and degrading oxalate, Analyst, 2014, 139, 4256–4263 RSC.
- C. F. Chow, P. Y. Ho, W. L. Wong and C. B. Gong, A Multifunctional Bimetallic Molecular Device for Ultrasensitive Detection, Naked-Eye Recognition, and Elimination of Cyanide Ions, Eur. J. Chem., 2015, 21, 12984–12990 CrossRef CAS PubMed.
-
(a)
H. Cheung, R. S. Tanke and G. P. Torrence, Ullmann's Encyclopedia of Industrial Chemistry, 2000;
(b) A. Sjode, S. Winestrand, N. O. Nilvebrant and L. J. Jonsson, Enzyme-based control of oxalic acid in the pulp and paper industry, Enzyme Microbiol. Technol., 2008, 43, 78 CrossRef;
(c) G. P. Kasidas and G. A. Rose, Measurement of plasma oxalate in healthy subjects and in patients with chronic renal failure using immobilised oxalate oxidase, Clin. Chim. Acta, 1986, 154, 49 CrossRef CAS PubMed.
-
(a) C. Walling and K. Amarnath, Oxidation of mandelic acid by Fenton's reagent, J. Am. Chem. Soc., 1982, 104, 1185 CrossRef CAS;
(b) H. Lim, J. Lee, S. Jin, J. Kim, J. Yoon and T. Hyeon, Highly active heterogeneous Fenton catalyst using iron oxide nanoparticles immobilized in alumina coated mesoporous silica, Chem. Commun., 2006, 463 RSC.
- Q. Wu and E. V. Anslyn, Catalytic signal amplification using a Heck reaction. An example in the fluorescence sensing of Cu(II), J. Am. Chem. Soc., 2004, 126, 14682–14683 CrossRef CAS PubMed.
-
(a) P. Scrimin and L. J. Prins, Sensing through signal amplification, Chem. Soc. Rev., 2011, 40, 4488–4505 RSC;
(b) R. Bonomi, A. Cazzolaro, A. Sansone, P. Scrimin and L. J. Prins, Detection of enzyme activity through catalytic signal amplification with functionalized gold nanoparticles, Angew. Chem., Int. Ed., 2011, 50, 2307 CrossRef CAS PubMed.
-
(a) N. Graf and R. Kramer, Enzymatic amplification in a bioinspired, autonomous signal cascade, Chem. Commun., 2006, 4375 RSC;
(b) M. S. Baker and S. T. Phillips, A two-component small molecule system for activity-based detection and signal amplification: application to the visual detection of threshold levels of Pd(II), J. Am. Chem. Soc., 2011, 133, 5170 CrossRef CAS PubMed;
(c) M. A. Swiderska and J.-L. Reymond, Analytical chemistry: a dendritic signal amplifier, Nat. Chem., 2009, 1, 527 CrossRef CAS PubMed;
(d) N. Graf, M. Goritz and R. Kramer, A metal-ion-releasing probe for DNA detection by catalytic signal amplification, Angew. Chem., Int. Ed., 2006, 45, 4013 CrossRef CAS PubMed;
(e) M. S. Baker and S. T. Phillips, A small molecule sensor for fluoride based on an autoinductive, colorimetric signal amplification reaction, Org. Biomol. Chem., 2012, 10, 3595 RSC;
(f) M. S. Masar III, N. C. Gianneschi, C. G. Oliveri, C. L. Stern, S. T. Nguyen and C. A. Mirkin, Allosterically regulated supramolecular catalysis of acyl transfer reactions for signal amplification and detection of small molecules, J. Am. Chem. Soc., 2007, 129, 10149 CrossRef PubMed.
-
(a) C. Shen, Q. Tang, C. B. Gong and C. F. Chow, Catalyst + Chemodosimeter → Chemosensor: incorporation of a catalytic functionality in an indicator displacement assay to realize reversible chemosensing detection, J. Mater. Chem. C, 2020 10.1039/C9TC06308K;
(b) M. T. Beck, Critical survey of stability constants of cyano complexes, Pure Appl. Chem., 1987, 59, 1703 CAS.
- C. Shen, A. Zheng, M. Huang, Q. Tang, C. B. Gong and C. F. Chow, Indicator/catalyst displacement assay: design of a latent catalyst for the selective detection and degradation of cyanide by Prussian blue analog-modified TiO2 nanoparticles, J. Mater. Chem. C, 2019, 7, 8585 RSC.
- A. Zheng, C. Shen, Q. Tang, C. B. Gong and C. F. Chow, Catalytic chemosensing assay for selective detection of methyl parathion organophosphate pesticide, Eur. J. Chem., 2019, 25, 9643–9649 CrossRef CAS PubMed.
- J. S. Yadav, R. K. Mehrotra and G. Srivastava, Metal and organometal complexes of oxy-and thiophosphorus acids. VIII. Reactions of o,o-diisopropyl and o,o-alkylene dithiophosphoric acidsh titanium and tin tetrachloride, Phosphorus, Sulfur Silicon Relat. Elem., 1991, 62, 169 CrossRef CAS.
- T. Ung, A. Hejl, R. H. Grubbs and Y. Schrodi, Latent ruthenium olefin metathesis catalysts that contain an N-heterocyclic carbene ligand, Organometallics, 2004, 23, 5399–5401 CrossRef CAS.
- B. K. Keitz and R. H. Grubbs, A tandem approach to photoactivated olefin metathesis: combining a photoacid generator with an acid activated catalyst, J. Am. Chem. Soc., 2009, 131, 2038–2039 CrossRef CAS PubMed.
- A. Ben-Asuly, A. Aharoni, C. E. Diesendruck, Y. Vidavsky, I. Goldberg, B. F. Straub and N. G. Lemcoff, Photoactivation of ruthenium olefin metathesis initiators, Organometallics, 2009, 28, 4652–4655 CrossRef CAS.
- C. F. Chow, P. Y. Ho, W. L. Wong, Y. J. Lu, Q. Tang and C. B. Gong, Catalyst displacement assay: a supramolecular approach for the design of smart latent catalysts for pollutant monitoring and removal, Chem. Sci., 2017, 8, 3812–3820 RSC.
- R. R. Dash, A. Gaur and C. Balomajumder, Cyanide in industrial wastewaters and its removal: a review on biotreatment, J. Hazard. Mater., 2009, 163, 1–11 CrossRef CAS PubMed.
Footnote |
† Dedicated to Professor Jean Marie Lehn on the occasion of his 80th birthday. |
|
This journal is © the Partner Organisations 2020 |
Click here to see how this site uses Cookies. View our privacy policy here.