Haemoglobin(βK120C)–albumin trimer as an artificial O2 carrier with sufficient haemoglobin allostery†
Received
29th April 2020
, Accepted 25th June 2020
First published on 13th July 2020
Abstract
The allosteric O2 release of haemoglobin (Hb) allows for efficient O2 delivery from the lungs to the tissues. However, allostery is weakened in Hb-based O2 carriers because the chemical modifications of the Lys- and Cys-β93 residues prevent the quaternary transition of Hb. In this paper, we describe the synthesis and O2 binding properties of a recombinant Hb [rHb(βK120C)]–albumin heterotrimer that maintains sufficient Hb allostery. The rHb(βK120C) core, with two additional cysteine residues at the symmetrical positions on its protein surface, was expressed using yeast cells. The mutations did not influence either the O2 binding characteristics or the quaternary transition of Hb. Maleimide-activated human serum albumins (HSAs) were coupled with rHb(βK120C) at the two Cys-β120 positions, yielding the rHb(βK120C)–HSA2 trimer, in which the Cys-β93 residues were unreacted. Molecular dynamics simulation demonstrated that the HSA moiety does not interact with the amino acid residues around the haem pockets and the α1β2 surfaces of the rHb(βK120C) core, the alteration of which retards Hb allostery. Circular dichroism spectroscopy demonstrated that the quaternary transition between the relaxed (R) state and the tense (T) state of the Hb core occurred upon both the association and dissociation of O2. In phosphate-buffered saline solution (pH 7.4) at 37 °C, the rHb(βK120C)–HSA2 trimer exhibited a sigmoidal O2 equilibrium curve with the O2 affinity and cooperativity identical to those of native Hb (p50 = 12 Torr, n = 2.4). Moreover, we observed an equal Bohr effect and 2,3-diphosphoglycerate response in the rHb(βK120C)–HSA2 trimer compared with naked Hb.
Introduction
Allosteric regulation plays a crucial role in controlling the protein function in biological systems through the binding of a small-molecule effector that induces a conformational change. Haemoglobin (Hb) is a well-known allosteric protein encapsulated in red blood cells (RBCs),1–5 and its allostery has been extensively studied to understand its mechanism.6–12 O2 binding converts the quaternary structure of Hb from a deoxy tense (T) state with a low O2 affinity to a relaxed (R) state with a high O2 affinity.1–4 The homotropic allosteric effect, which is known as cooperativity, allows Hb to efficiently transport O2 from the lungs to the peripheral tissues. As a heterotropic allosteric effect, 2,3-diphospoglycerate (DPG) stabilizes the T-state conformation by creating salt bridges between the two β subunits to reduce O2 affinity.13,14 Furthermore, O2 affinity decreases with decreasing pH (the Bohr effect), shifting the equilibrium toward the T state.11,15,16
Artificial O2 carriers are currently required as RBC substitutes for transfusion therapy because of worldwide shortages in blood supply.17,18 However, the stroma-free Hb splits to an αβ dimer (α2β2 → 2αβ) in the bloodstream and is immediately expelled into urine. Moreover, Hb diffuses into the interstitial spaces between the endothelial cells and smooth muscle cells, causing hypertension by scavenging nitric oxide (NO; endothelial-derived vasodilator).19,20 Therefore, naked Hb is not available for use in transfusion therapy. Since the 1980s, several types of Hb-based O2 carriers have been developed, including cross-linked Hb,21,22 polymerized Hb,23,24 and PEGylated Hb.25–27 However, these chemical modifications have markedly reduced cooperativity, the Bohr effect, and the DPG effect,18 and a modified Hb that maintains the original allosteric effects of Hb has never been reported.18 We previously synthesized a protein cluster as an RBC substitute consisting of an Hb molecule wrapped covalently in human serum albumins (HSAs), called an Hb–HSAm cluster (m = 2–4, average m = 3.0 ± 0.2).28,29 This protein cluster shows a long circulation lifetime in the bloodstream and a superior biocompatibility.30,31 The quaternary structural analysis of the Hb–HSAm cluster revealed that the chemical modification of the Lys and Cys-β93 residues of Hb by N-succinimidyl 3-maleimidopropionate as a cross-linker prevents the R–T quaternary transition and maintains the Hb core close to the R state.32 Thus, the Hb–HSAm cluster demonstrated a low cooperativity, a low Bohr effect, and a low DPG effect. If the HSA shells are linked at appropriate positions on the Hb surface so that they do not affect the R–T quaternary motion, the resulting Hb–HSAm conjugate maintains the original O2 binding properties of the native Hb. The genetically introduced cysteine could act as a cross-linking point for maleimide-activated HSA (MA-HSA). We assume that the mutation of Hb residues, excluding the mutation of (i) the α1β2 interfaces, including the N- and C-terminals (which play important roles in the R–T quaternary transition), and (ii) the haem pockets (which directly affect O2 affinity), would allow the resulting Hb variant to maintain its original O2 binding capability. Based on this hypothesis, the Lys-β120 residues were chosen as the symmetrical mutation points (Fig. 1A). In this study, we describe a novel recombinant Hb variant, rHb(βK120C), in which Lys-β120 is replaced with Cys at symmetrical positions on the Hb surface, and we also present the rHb(βK120C)–HSA2 heterotrimer, in which MA-HSAs are linked at the Cys-β120 positions (Fig. 1B). Ultraviolet-Visible (UV-vis) and circular dichroism (CD) spectroscopies revealed that the quaternary structure of the rHb(βK120C)–HSA2 trimer is reversibly convertible between the R and T states. We also observed identical O2 affinity and allosteric effects in the rHb(βK120C)–HSA2 trimer compared with naked Hb.
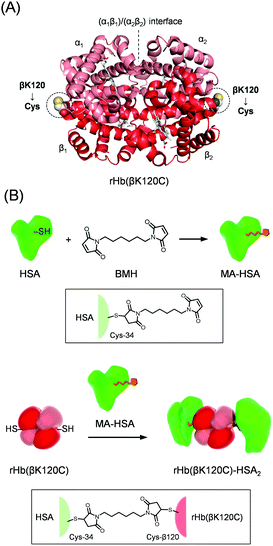 |
| Fig. 1 (A) Recombinant Hb variant [rHb(βK120C)] with Lys-β120 residues replaced by cysteines at the symmetrical positions. The mutation point was designed to avoid the haem pockets and α1β2 interfaces. (B) Synthetic routes of maleimide-activated HSA (MA-HSA) and the rHb(βK120C)–HSA2 trimer. The Cys-34 of the HSA was reacted with 1,6-bis(maleimido)hexane (BMH), resulting in MA-HSA. The two Cys-β120 residues at symmetrical positions were reacted with MA-HSA to yield the rHb(βK120C)–HSA2 trimer. | |
Results and discussion
Expression and physicochemical properties of rHb(βK120C)
We first expressed rHb(βK120C) using Pichia yeast (Pichia pastoris GS115) according to our previous reports.33,34 The expressed rHb(βK120C) was purified using cation- and anion-exchange chromatographies (CEC and AEC). The sodium dodecyl sulfate–polyacrylamide gel electrophoresis (SDS–PAGE) analysis of rHb(βK120C) depicted two clear bands corresponding to the α- and β-subunits (Fig. 2A). The α- and β-chain bands demonstrated near-identical mobility to those of native Hb. The matrix-assisted laser desorption/ionization-time of flight (MALDI-TOF) mass spectrum analysis of rHb(βK120C) exhibited molecular-related ion peaks at 15
122 and 15
841 Da. These were almost identical to the simulated masses of the amino acid sequences (15
127 Da for the α-chain and 15
842 Da for the β-chain), indicating that Lys-β120 was replaced with cysteine. The size-exclusion chromatography (SEC) chromatogram of rHb(βK120C) exhibited a peak with the same elution volume as that of native Hb, indicating that the tetramer (α1α2β1β2) was formed in the rHb(βK120C) (Fig. 2B; elution volume = 1.9 mL). Fortunately, the oligomeric rHb(βK120C) species created via the disulfide bonds of Cys-β120 was not observed in the SEC chromatogram. The fact that rHb(βK120C) and native Hb had identical CD spectra (λ = 200–250 nm) indicates that their secondary structures were equivalent (Fig. 2C). The isoelectric point (pI) value of rHb(βK120C) was determined to be 6.8, which was slightly lower than that of native Hb (pI = 6.9) because the mutation (Lys-β120 → Cys) had negatively shifted the net surface charge (Fig. S1, ESI†). The results of the sulfhydryl group assay using 4,4′-dithiodipyridine (4,4′-DTP)35 revealed the number of reactive sulfhydryl groups in rHb(βK120C) and native Hb as 4.0 and 2.0, respectively. This result strongly indicates that the two Lys-β120 residues of the β1 and β2 chains were replaced by Cys and that the introduced Cys-β120 residues were reactive with 4,4′-DTP, as they are located at the molecular surface of rHb(βK120C).
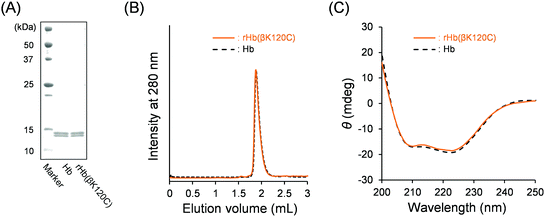 |
| Fig. 2 (A) SDS–PAGE results of native Hb and rHb(βK120C). (B) SEC profiles of rHb(βK120C) and native Hb. (C) CD spectra of the rHb(βK120C) and native Hb in PBS at 25 °C ([protein] = 0.2 μM). | |
Preparation of the rHb(βK120C)–HSA2 trimer
To prepare MA-HSA, we used 1,6-bis(maleimido)hexane (BMH) as the cross-linking agent. One of the two maleimide groups in BMH was reacted with the free sulfhydryl group of the Cys-34 in HSA (which is the only reduced-form of cysteine in HSA), yielding MA-HSA. The formation of the HSA dimer was not observed because of electrostatic repulsion between the negatively charged HSA surfaces present under our experimental conditions. The sulfhydryl group assay using 4,4′-DTP revealed the complete disappearance of the reduced form.
Capping of the Cys-β93 residues of native Hb with maleimide derivatives causes a decline in O2 binding cooperativity, the Bohr effect, and the influence of the allosteric effectors. The Cys-β93 residues of native Hb were able to react with maleimide derivatives, such as N-ethyl maleimide (NEM) and N-succinimidyl 3-maleimidopropionate.32,36 We found that the Cys-β93 residues had not reacted with MA-HSA because of the steric hindrance between the native Hb and MA-HSA. In contrast, genetically introduced Cys-β120 located at the molecular surface of rHb(βK120C) had reacted with MA-HSA. The SEC chromatogram of the reaction mixture [rHb(βK120C) + MA-HSA] revealed a new peak in the high-molecular-weight region (Fig. 3A; elution volume = 1.4 mL), and native PAGE analysis also revealed a new band above that of rHb(βK120C) (Fig. 3B). The high-molecular-weight component was purified using AEC followed by gel filtration chromatography (GFC). The ion peak of the β-chain at 15.8 kDa in the MALDI-TOF MS disappeared after the reaction of rHb(βK120C) with MA-HSA. A new peak then appeared at 82.5 kDa, a value almost identical to the simulated mass of a β-chain + MA-HSA (82.3 kDa), indicating that the HSA moiety was covalently linked to the β-chain of rHb(βK120C). Based on the [total protein]/[Hb unit] assays, the average HSA/Hb ratio of the product was determined to be 2.0. The heme loss was not observed during the preparation. We denoted this hybrid protein as rHb(βK120C)–HSA2 (Mw: 198 kDa). The CD spectrum of the rHb(βK120C)–HSA2 trimer coincided perfectly with the sum of the rHb(βK120C) spectrum and a two-times-enlarged HSA spectrum (Fig. 3C). This result demonstrates that the secondary structures of the individual proteins were unaltered by the protein coupling. The dynamic light scattering measurements revealed that the hydrodynamic diameter of the rHb(βK120C)–HSA2 trimer (12.4 ± 2.8 nm, P.I. = 0.010) was significantly larger than that of rHb(βK120C) (6.9 nm ± 1.9 nm, P.I. = 0.004). The pI value of the trimer (pI = 5.4) was close to that of HSA (pI = 4.9), which further indicates the coupling of rHb(βK120C) (pI = 6.7) with HSAs (Fig. S1, ESI†). The net negative surface charge and the large molecular size could allow the rHb(βK120C)–HSA2 trimer to circulate in the bloodstream for long periods of time and prevent the undesirable vasopressor response through NO depletion.19,20 Furthermore, the sulfhydryl group assay using 4,4′-DTP35 demonstrated that the number of reactive sulfhydryl groups in the rHb(βK120C)–HSA2 trimer was 1.8, indicating that the Hb core maintained the free Cys-β93 residues that are important for O2 binding ability, as mentioned above.36 We could not observe the dissociation of the α2β2 tetramer into two αβ dimers during the synthesis, purification, and measurements of rHb(βK120C)–HSA2, implying that the α2β2 tetramer was sufficiently stable the same as native Hb under our experimental conditions.
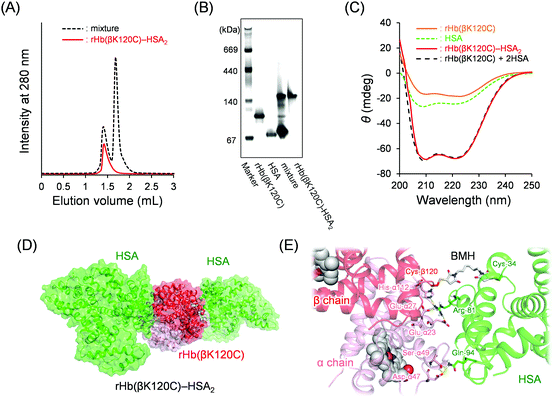 |
| Fig. 3 (A) SEC profiles of the resultant reaction mixture [rHb(βK120C) + MA-HSA] and the purified rHb(βK120C)–HSA2 trimer. (B) Native PAGE patterns of rHb(βK120C), HSA, the reaction mixture, and the purified rHb(βK120C)–HSA2 trimer. (C) CD spectra of rHb(βK120C), HSA, rHb(βK120C), and rHb(βK120C)–HSA in PBS at 25 °C ([protein] = 0.2 μM). (D and E) The energy-minimized structure of the rHb(βK120C)–HSA2 trimer obtained by a molecular dynamics (MD) calculation using the Desmond software. | |
An MD simulation was used to predict the interactions between the rHb(βK120C) and HSA moieties. The Cys-β1120 and Cys-β2120 residues exist at symmetrical positions of the core Hb; therefore, a sufficient distance is maintained between the two HSA parts in the rHb(βK120C)–HSA2 trimer. We performed MD simulation using a half model [αβ(K120C)–HSA] constructed using the crystal structures of native Hb (PDB ID: 2DN1)37 and HSA (PDB ID: 1AO6)38 (Fig. S2, ESI†). The main chains (Cα and amide atoms) of the αβ(K120C) moiety were frozen for the duration of a 50 ns simulation. The root-mean-square deviation (RSMD) and the distances of the salt bridges and hydrogen bonds between the αβ(K120C) and HSA residues reached a plateau within 25 ns (Fig. S3, ESI†). The MD simulation structure was represented in the rHb(βK120C)–HSA2 form which was prepared by overlapping the two αβ(K120C) moieties of the models with the crystal structure of native Hb (α1β1α2β2; Fig. 3D and Fig. S2B, ESI†). The distance between the sulfide atoms of Cys-β120 [rHb(βK120C)] and Cys-34 (HSA) was measured to be 13.8 Å, meaning that the BMH was long enough to cross-link the Cys residues (Fig. 3E). We found that the following salt bridges and hydrogen bonding interactions between rHb(βK120C) and HSA helped stabilize the conformation of the rHb(βK120C)–HSA2 trimer: (i) Glu-α23, Glu-α27, and His-α112 of rHb(βK120C)—Arg-81 of HSA and (ii) Ser-α49 and Asp-α47 of rHb(βK120C)—Gln-94 of HSA. The HSA moiety did not interact with the amino acid residues around the haem pockets and the α1β2 surfaces of the rHb(βK120C) core.
Quaternary structure analysis of the Hb core
We investigated the quaternary structure of the Hb core using UV-vis absorption and CD spectroscopies. The UV-vis spectral patterns and the absorbances of the oxy, deoxy, and carbonyl rHb(βK120C) as well as the rHb(βK120C)–HSA2 trimer were indistinguishable from those of native Hb in PBS solution (pH 7.4; Fig. 4A and Table S1, ESI†). Generally, the chemical modification of the Lys and Cys-β93 residues of Hb decreases the Soret band absorption of the deoxy form, because the deoxy Hb remains near the R-state quaternary structure.32 In contrast, the deoxy rHb(βK120C) and the rHb(βK120C)–HSA2 trimer revealed the same Soret band absorption as that of deoxy native Hb, indicating that the identical T-state quaternary structures were formed. CD spectroscopy was also used to analyse the quaternary structure of the Hb core in the rHb(βK120C)–HSA2 trimer. Generally, deoxy Hb (T state) exhibits a negative CD band at 287 nm, which is known as the T-state marker.39–41 At the same time, this band is not usually observed in the R-state quaternary structure of oxy Hb. The CD spectral patterns and intensities of oxy and deoxy rHb(βK120C) both resembled those of native Hb, implying that the oxy and deoxy forms of these proteins are comparable (Fig. 4B).39 CD also revealed similar spectral changes in the rHb(βK120C)–HSA2 trimer upon deoxygenation. CD spectra for the oxy and deoxy Hb cores in the rHb(βK120C)–HSA2 trimer were obtained by subtracting the two-times-enlarged HSA spectrum from the corresponding rHb(βK120C)–HSA2 trimer spectra (Fig. 4B). The CD spectra of the oxy and deoxy Hb cores coincided with those of rHb(βK120C). These spectral analyses revealed that the mutation Lys-β120 → Cys and coupling of HSA at the Cys-β120 positions did not influence the quaternary structural transition between the R and T states.
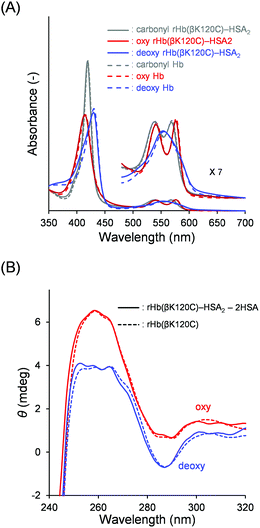 |
| Fig. 4 (A) UV-vis absorption spectra of the carbonyl (gray), oxy (red), and deoxy (blue) rHb(βK120C)–HSA2 trimer (solid line) and native Hb (dotted line). (B) CD spectra (240–320 nm) of the oxy (red) and deoxy (blue) Hb components of the rHb(βK120C)–HSA2 trimer [rHb(βK120C)–HSA2 − twofold HSA] (solid line) and rHb(βK120C) (dotted line). The measurements were performed in PBS solution (pH 7.4) at 25 °C ([protein] = 3 μM). | |
O2 binding affinity and cooperativity
Hb binds with O2 in the lungs, where there is a high O2 partial pressure (pO2), and releases O2 into the peripheral tissues, where there is a low pO2. To evaluate the O2 binding properties, O2 affinity (p50; pO2 where Hb is half-saturated with O2) and the Hill coefficient (n; degree of cooperativity) of Hb were determined from the O2 equilibrium curve (OEC). As shown in Fig. 5A, a sigmoidal OEC was observed for native Hb in PBS solution (pH 7.4) at 37 °C (p50 = 12 Torr; n = 2.4). Upon the release of O2, the quaternary structure of Hb converts from the R state with a high O2 affinity to the T state with a low O2 affinity. The cooperative O2 release allows Hb to efficiently deliver O2 from the lungs to the tissues. Nevertheless, chemically modified Hbs have demonstrated a low cooperativity because the quaternary transition of the Hb core is prevented.32 Such modified Hbs include PEG-Hb (71% reduction),18,42 polymerized Hb (41% reduction),18,42 and Hb–HSAm clusters (71% reduction).29,42 In contrast, the OECs of rHb(βK120C) and the rHb(βK120C)–HSA2 trimer were found to have a sigmoidal shape completely consistent with that of native Hb (Fig. 5A). The O2 binding parameters of rHb(βK120C) (p50 = 12 Torr, n = 2.3) and the rHb(βK120C)–HSA2 trimer (p50 = 12 Torr, n = 2.4) were also equivalent to those of native Hb (Table 1). Both rHb(βK120C) and the rHb(βK120C)–HSA2 trimer were able to maintain identical O2 affinity and cooperativity because the quaternary structure of the Hb units was interconvertible between the R and T states upon O2 association and dissociation, as in native Hb. The capping of Cys-β93 with NEM in native Hb (NEM-Hb) reduced the p50 and n values (p50 = 8 Torr, n = 1.9). The NEM-rHb(βK120C)–HSA2 trimer demonstrated O2 binding parameters similar to those of NEM-Hb, clearly indicating that the Cys-β93 residues in the rHb(βK120C)–HSA2 trimer were in a reduced form. This result is supported by the sulfhydryl group assay mentioned above. We concluded that the selective coupling of MA-HSA occurred at the two Cys-β120 positions of the rHb(βK120C), whereas it did not take place at the Cys-β93 residues because of steric hindrance. On the basis of these findings, we also concluded that the Lys-β120 → Cys mutation and the selective conjugation of MA-HSA at the Cys-β120 positions were impervious to O2 affinity and cooperativity. To the best of our knowledge, this is the first example of a chemically modified Hb showing an identical O2 affinity and cooperativity to those of native Hb.18
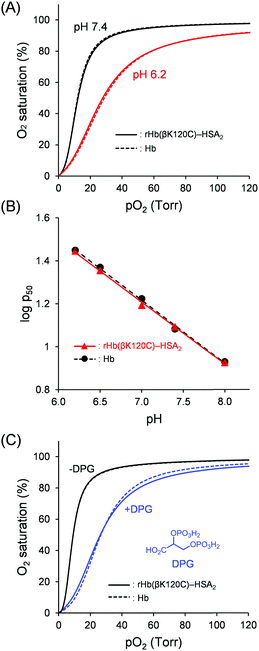 |
| Fig. 5 OECs and Bohr plots of Hb (dotted line) and the rHb(βK120C)–HSA2 trimer (solid line) at 37 °C ([protein] = 5 μM). (A) OECs in PBS solutions at pH 7.4 (black) and pH 6.2 (red). (B) The Bohr plots of Hb (black circle) and the rHb(βK120C)–HSA2 trimer (red triangle) in PBS solutions (pH 6.2, 6.5, 7.0, 7.4, and 8.0). (C) OECs in 50 mM Tris–HCl buffer solution with 1 mM DPG (blue) and without DPG (black). | |
Table 1 O2 binding parameters and Bohr coefficients of haemoproteins in PBS solution at 37 °C
Haemoprotein |
pH 7.4 |
pH 6.2 |
Bohr coefficienta |
p
50 (Torr) |
n (—) |
p
50 (Torr) |
n (—) |
The Bohr coefficients were derived from the slopes obtained from plotting −Δlog p50/ΔpH between pH 6.2 and 8.0 (Fig. 5B).
|
Hb |
12 |
2.4 |
28 |
1.9 |
0.29 |
rHb(βK120C) |
12 |
2.3 |
29 |
1.9 |
0.29 |
rHb(βK120C)–HSA2 |
12 |
2.4 |
28 |
1.9 |
0.29 |
The autoxidation rate constant (kox) of the oxygenated Hb core in the rHb(βK120C)–HSA2 trimer was measured in PBS solution (pH 7.4) at 37 °C. The kox value of the rHb(βK120C)–HSA2 trimer was ascertained as 0.027 h−1, which was almost similar to the data of native Hb (0.020 h−1). The oxy form of the internal Hb maintains good stability even after being covered with HSAs.
Bohr effect on rHb(βK120C)–HSA2 trimer
A high metabolic activity leads to an increase in the concentration of CO2 and a decrease in the pH value within the tissues. Under low-pH conditions, the protonation of Hb occurs and the T-state quaternary structure is stabilized, reducing O2 affinity (the Bohr effect). Thus, the OEC of native Hb was right-shifted to a p50 value of 28 Torr at pH 6.2 compared with p50 = 12 Torr at pH 7.4 (Table 1 and Fig. 5A). The Bohr effect allows for Hb high O2-transport efficiency to the tissues. However, chemical modifications have tended to notably reduce the Bohr effect, such as in PEG-Hb (87% reduction)18 and polymerized Hb (75% reduction).18 We observed that the O2 affinities of rHb(βK120C) and the rHb(βK120C)–HSA2 trimer were reduced under lower pH conditions, similar to native Hb (p50: 12 → 29 Torr (pH 7.4 → 6.2)). The p50 and n values of both rHb(βK120C) and the rHb(βK120C)–HSA2 trimer were nearly identical to those of native Hb at pH 6.2 (Table 1). The Bohr coefficients were derived from the slopes obtained from plotting −Δlog
p50/ΔpH between pH 6.2 and 8.0 (Fig. 5B and Table S2, ESI†). The Bohr coefficients of both rHb(βK120C) and the rHb(βK120C)–HSA2 trimer were identical to those of native Hb (Table 1). The value measured for native Hb, however, was somewhat weaker than the result reported by Ho et al.43 This difference is probably attributable to several factors, such as the difference in temperature (37 °C vs. 29 °C) and the difference in solvents (PBS with low phosphate and high NaCl concentrations vs. 0.1 M sodium phosphate buffer (PB)). Altogether, we conclude that neither the Lys-β120 → Cys mutation nor the HSA conjugation affected the Bohr effect.
Effects of DPG on O2 binding affinity
Allosteric effectors bind to proteins, altering their activity. The most important allosteric regulator of Hb in RBCs is DPG, which reduces the O2 binding affinity (the DPG effect). An X-ray crystallography analysis has previously revealed that a single DPG molecule binds to the cleft of Hb β chains, known as the DPG binding site.13,14 The anionic groups of DPG form salt bridges with the cationic groups of the β-chains, including the His-β2, Lys-β82, and His-β143 residues, stabilizing the T-state conformation and reducing O2 affinity. The T → R conformational change induced by oxygenation leads to the closure of the binding site and weakens the DPG binding affinity to oxy Hb. This DPG response allows Hb to efficiently deliver O2 from the lungs to the tissues. We measured the OEC of native Hb in PBS (pH 7.4) solution containing 1 mM DPG at 37 °C, but a reduction in O2 affinity was not observed because of the fact that phosphate anions in the PBS solution interrupt the binding of DPG to Hb. Thus, we used 0.1 M Tris–HCl buffer for DPG response experiments. Indeed, native Hb in Tris–HCl buffer demonstrated a higher O2 affinity (p50 = 9 Torr) than in PBS solution because of the exclusion of the Hb–phosphate anion interactions (Table 2). The O2 affinity of the native Hb was also reduced upon the addition of DPG to Tris–HCl buffer. Interestingly, rHb(βK120C) (p50 = 26 Torr) and the rHb(βK120C)–HSA2 trimer (p50 = 26 Torr) demonstrated identical DPG responses to native Hb (p50 = 27 Torr; Fig. 5C). These results indicate that the rHb(βK120C)–HSA2 trimer maintained a DPG response similar to that of native Hb and that its O2 affinity is controllable via the allosteric effector.
Table 2 Effect of DPG on hemoproteins in 50 mM Tris–HCl buffer solution (pH 7.4) at 37 °C containing 1 mM DPG
Hemoprotein |
Without DPG |
With DPG |
p
50 (Torr) |
n (—) |
p
50 (Torr) |
n (—) |
Hb |
9 |
2.3 |
27 |
2.4 |
rHb(βK120C) |
9 |
2.3 |
26 |
2.1 |
rHb(βK120C)–HSA2 |
9 |
2.3 |
26 |
2.1 |
Conclusions
A novel Hb variant (Lys-β120 → Cys) was designed to prepare the rHb(βK120C)–HSA2 trimer as an artificial O2 carrier. The mutation did not affect the O2 binding properties of Hb. Two MA-HSA molecules were selectively linked to Cys-β120 in rHb(βK120C), resulting in the rHb(βK120C)–HSA2 trimer in which Cys-β93 remained free because of steric repulsion between the rHb(βK120C) core and the MA-HSA shell. The CD spectra revealed that the R–T quaternary motion of the Hb core in the rHb(βK120C)–HSA2 trimer occurred upon O2 association and dissociation. Thus, the rHb(βK120C)–HSA2 trimer demonstrated sufficient allosteric O2 binding properties with respect to cooperativity, the Bohr effect, and the DPG effect. The rHb(βK120C)–HSA2 trimer is a unique artificial O2 carrier with sufficient allostery to be an RBC substitute.
Materials and methods
Materials and apparatus
1,6-Bis(maleimido)hexane (BMH) was purchased from Tokyo Chemical Industry Co., Ltd. Human serum albumin (HSA, albumin 25%, Benesis) was purchased from the Japan Blood Products Organization. The other special-grade chemicals were used without additional purification unless otherwise noted. Water was deionized (18.2 MΩ cm) using two water purification systems (Elix UV and Milli Q Reference; Millipore Corp.). The SDS–PAGE and native PAGE analyses were performed using a 15% or 5–12% poly(acrylamide) precast gel (SuperSep Ace 15%; SuperSep Ace 5–12%; Fujifilm Wako Pure Chemical Corp.). Isoelectric focusing (IEF) was performed using a pH 3–10 IEF protein gel (Novex; Thermo Fischer Scientific Inc.). The UV-vis absorption spectra were obtained using a UV-Visible spectrophotometer (8543; Agilent Technologies Inc. or V-650; Jasco Corp.). CD spectra were recorded using a CD spectrometer (J-820; Jasco Corp.) at 25 °C.
Expression and preparation of rHb(βK120C)
The expression plasmid for the rHb(βK120C) variant [pHIL-D2-rHb(βK120C)] was constructed according to the standard protocol of the QuickChange XL Site-Directed Mutagenesis Kit (Agilent Technologies Inc.) using a pHIL-D2-rHb(α- and β-chains) vector34 and an oligonucleotide primer set (forward [5′-CTCGCGCATCACTTCGGC![[T with combining low line]](https://www.rsc.org/images/entities/char_0054_0332.gif)
![[G with combining low line]](https://www.rsc.org/images/entities/char_0047_0332.gif)
GAGTTTACACCGCCAGTTC-3′] and reverse [5′-GAACTGGCGGTGTAAACTC![[A with combining low line]](https://www.rsc.org/images/entities/char_0041_0332.gif)
![[C with combining low line]](https://www.rsc.org/images/entities/char_0043_0332.gif)
GCCGAAGTGATGCGCGAG-3′]). The obtained pHIL-D2-rHb(βK120C) vector was linearized with SalI and used to transform the GS115-strain Pichia pastoris (Thermo Fisher Scientific K.K.) via electroporation.
Transformed clone cells were grown in a buffered mineral glycerol complex (BMGY) medium (4 L total) in a shaking incubator (Bio-Shaker G·BR-200; Taitec Corp.; 200 rpm, 30 °C) and subsequently in a buffered mineral methanol complex (BMMY) medium (1.6 L total) containing 0.3 mM hemin for 5 days. During cultivation, 100% methanol at 1.5% of the medium volume was added every 24 h. The cells were harvested by centrifugation at 3000g for 10 min. The obtained cells were then washed with water (300 mL × 2) and resuspended in 100 mL of sodium PB solution (10 mM, pH 6.0) containing 1 mM phenylmethanesulfonyl fluoride. After the addition of glass beads (150 mL, d = 0.5 mm), the cells were lysed using a BeadBeater (Biospec Products, Inc.) with four cycles of disruption (2 min) and incubated by cooling on ice (2 min). After centrifugation at 12
000g for 1 h, the supernatant was equilibrated with CO atmosphere. The solution was loaded onto a cation exchange chromatography column (SP Sepharose Fast Flow, GE Healthcare UK Ltd), which was equilibrated in 10 mM PB (pH 6.0). After washing with the same buffer solution, the target protein was eluted using 20 mM Tris–HCl buffer solution (pH 8.0). Next, the resulting protein solution was subjected to AEC using a Q Sepharose Fast Flow column (GE Healthcare UK Ltd) with 20 mM Tris–HCl (pH 8.0) as the running buffer. After washing with 20 mM Tris–HCl (pH 8.0), rHb(βK120C) was eluted using PBS solution (pH 7.4). The purity was checked using SDS–PAGE analysis and SEC on a high-performance liquid chromatography (HPLC) system (AKTA purify; GE Healthcare UK Ltd). The system was equipped with an SEC column (Superdex 200 Increase, 5/150 GL; GE Healthcare UK Ltd), and PBS solution (pH 7.4) was used as the mobile phase. The concentration of rHb(βK120C) was measured using a protein assay kit (Pierce 660 nm; Thermo Fisher Scientific K.K.). The obtained rHb(βK120C) had a concentration of approximately 100 mg L−1 of media. The sulfhydryl group assay of rHb(βK120C) was conducted by reaction with 4,4′-dithiodipyridine (4,4′-DTP).35
Preparation of MA-HSA
The 25% HSA solution (4 mL) was diluted with PBS solution (14 mL, pH 7.4). A DMSO solution of BMH (7.5 mM, 2 mL) was then added dropwise to the HSA solution (8.4 mM, 18 mL). After stirring for 3 h at 25 °C, the reactant was subjected to a GFC column (Sephadex G25 superfine; GE Healthcare UK Ltd) to remove the unreacted cross-linker. The protein concentration was measured using a protein assay kit (Pierce 660 nm), whereas the sulfhydryl group assay of MA-HSA was conducted by reaction with 4,4′-DTP. The MA-HSA solution was stored at −80 °C.
Preparation of rHb(βK120C)–HSA2
The MA-HSA and rHb(βK120C) solutions were mixed and concentrated to 6 mL ([MA-HSA] = 0.4 mM, [rHb(βK120C)] = 0.1 mM). The reactant was stirred under dark conditions for 24 h at 4 °C. An aliquot of the reaction mixture was analysed using SEC on an HPLC system (AKTA purify; GE Healthcare UK Ltd) equipped with an SEC column (Superdex 200 Increase, 5/150 GL; GE Healthcare UK Ltd) and using PBS solution (pH 7.4) as the mobile phase. The reaction mixture was purified using AEC using a Q Sepharose Fast Flow column (GE Healthcare UK Ltd). The mixture solution was diluted with the same volume of water and loaded onto the column equilibrated with PBS solution. The column was then washed using 10 mM PB (pH 7.4) solution. The rHb(βK120C)–HSA2 trimer was eluted with a 10 mM PB (pH 6.0) + 120 mM NaCl solution. Under this condition, the HSA dimer remained in the column. The collected solution was concentrated and subjected to GFC using a Superdex 200 pg column (GE Healthcare UK Ltd) to isolate the rHb(βK120C)–HSA2 trimer (yield: 50%). The total protein and Hb concentrations were measured using a protein assay kit (Pierce 660 nm; Thermo Fischer Scientific Inc.) and the extinction coefficient of cyano-metHb (ε541 = 4.4 × 104 M−1 cm−1), respectively. The average [HSA]/[rHb(βK120C) unit] ratio of the product was estimated to be 2.0. The sulfhydryl group assay of MA-HSA was conducted via the reaction with 4,4′-DTP.
Molecular dynamics simulations
MD simulations were performed using the Desmond application implemented in a Maestro graphical interface.44,45 We used the optimized potentials for liquid simulations (OPLS_2005) force field implemented in the Desmond software for all molecules in the system.46 We constructed a half model of rHb(βK120C) [αβ(K120C)–HSA model] because rHb(βK120C)–HSA2 has a C2 symmetry, and there has to be a sufficient distance between the HSAs for their interactions to be negligible. The crystal structure of native Hb (PDB ID: 2DN1)37 was used for the αβ(K120C) model. Lys-β120 was replaced with Cys using the PyMOL software (PyMOL Molecular Graphics System Version 2.0, Schrödinger, LLC.). For the HSA moiety, we used the crystal structure of HSA (PDB ID: 1AO6).38 The missing atoms of the side chains in the HSA were added to the model using the PyMOL software. The water molecules in the model were removed. The bond orders were assigned, and the hydrogen atoms were added to the models using the Protein Preparation Wizard tool47 in the Maestro software. The αβ(K120C) and HSA models were covalently linked using the BMH cross-linker [Cys-β120(αβ)-BMH-Cys-34(HSA)] and arranged so that sufficient distance was maintained between them (Fig. S2A, ESI†). The Cys-β120(Hb), Cys-34(HSA), and BMH moieties were minimized using the 3D builder in the Maestro software. The hydrogen networks and the protonated states were optimized to pH 7 using the Protein Preparation Wizard for which the pKa values of the protein residues were predicted using PROPKA.48 The αβ(K120C)–HSA model was solvated using explicit TIP3P water49 with a buffering distance of 10 Å in an octahedral box. Sodium counterions were added to neutralize the charges. The model was relaxed using a default relaxation protocol implemented in the Desmond software. An isothermal–isobaric MD simulation was performed for 50 ns to maintain the 300 K temperature and 1.01325 bar pressure. The main chains (Cα and amide atoms) of the αβ(K120C) moiety were frozen during the simulation. The time step was 2 fs, and the information for analysis was printed every 100 ps. A 10 Å cut off was used for non-bonded interactions.
Preparation of oxy and deoxy forms of the rHb(βK120C)–HSA2 trimer
The oxy (O2 complex) rHb(βK120C)–HSA2 trimer solution (PBS, pH 7.4, 3 μM, 3 mL) was prepared using our previously reported technique.29 The solution was transferred to an optical quartz cuvette (10 mm path length) with a rubber septum cap. N2 gas was blown into the oxy-form solution to yield the deoxy rHb(βK120C)–HSA2 trimer. The UV-vis absorption and CD spectra of these species were recorded at 25 °C.
O2 binding parameters
The O2 affinity (p50; O2 partial pressure where Hb is half-saturated with O2) and Hill coefficient (n) were determined using an automatic recording system for the O2 equilibrium curve (Hemox Analyzer; TCS Scientific Corp.) at 37 °C. The oxy-form of the rHb(βK120C)–HSA2 trimer solution (PBS, pH 7.4, approximately 5 μM, 4 mL) was used for the measurements. The trimers in PBS solution (pH 7.4) were deoxygenated by flushing with N2 and oxygenated by increasing the O2 partial pressure.
O2 complex stability
The oxy form stability of the rHb(βK120C)–HSA2 trimer was assessed using the first-order autoxidation rate constant (kox) of the core Hb using our earlier described procedures.50
Conflicts of interest
There are no conflicts to declare.
Acknowledgements
This work was supported by a Grant-in-Aid for Challenging Research (Exploratory) (No. 18K19007) and Early-Career Scientists (No. 19K20699) from JSPS, the Science Research Promotion Fund from Promotion and Mutual Aid Corporation for Private Schools of Japan, a Joint Research Grant from the Institute of Science and Engineering, Chuo University, and a Research Grant from Naito Foundation.
Notes and references
- M. F. Perutz, H. Muirhead, J. M. Cox and L. C. G. Goaman, Nature, 1968, 219, 131–139 CrossRef CAS PubMed.
- M. F. Perutz, Nature, 1970, 228, 726–734 CrossRef CAS PubMed.
- J. Baldwin and C. Chothia, J. Mol. Biol., 1979, 129, 175 CrossRef CAS PubMed.
- G. Fermi, M. F. Perutz, B. Shaanan and R. Fourme, J. Mol. Biol., 1984, 175, 159 CrossRef CAS PubMed.
- W. A. Eaton, E. R. Henry, J. Hofrichter and A. Mozzarelli, Nat. Biol., 1999, 6, 351–358 CrossRef CAS PubMed.
- S. Adachi, S. Y. Park, J. R. H. Tame, Y. Shiro and N. Shibayama, Proc. Natl. Acad. Sci. U. S. A., 2003, 100, 7039–7044 CrossRef CAS PubMed.
- E. M. Jones, G. Balakrishnan and T. G. Spiro, J. Am. Chem. Soc., 2012, 134, 3461–3471 CrossRef CAS PubMed.
- C. Viappiani, S. Abbruzzetti, L. Ronda, S. Bettati, E. R. Henry, A. Mozzarelli and W. A. Eaton, Proc. Natl. Acad. Sci. U. S. A., 2014, 111, 12758–12763 CrossRef CAS PubMed.
- N. Shibayama, K. Sugiyama, J. R. Tame and S. Y. Park, J. Am. Chem. Soc., 2014, 136, 5097–5105 CrossRef CAS PubMed.
- E. M. Jones, E. Monza, G. Balakrishnan, G. C. Blouin, P. J. Mak, Q. H. Zhu, J. R. Kincaid, V. Guallar and T. G. Spiro, J. Am. Chem. Soc., 2014, 136, 10325–10339 CrossRef CAS PubMed.
- Y. Yuan, M. F. Tam, V. Simplaceanu and C. Ho, Chem. Rev., 2015, 115, 1702–1724 CrossRef CAS PubMed.
- N. Shibayama, A. Sato-Tomita, M. Ohki, K. Ichiyanagi and S. Y. Parkc, Proc. Natl. Acad. Sci. U. S. A., 2020, 117, 4741–4748 CrossRef CAS PubMed.
- A. Arnone, Nature, 1972, 237, 146–149 CrossRef CAS PubMed.
- V. Richard, G. G. Dodson and Y. Mauguen, J. Mol. Biol., 1993, 233, 270–274 CrossRef CAS PubMed.
- C. Ho and I. M. Russu, Biochemistry, 1987, 26, 6299–6305 CrossRef CAS PubMed.
- T.-Y. Fang, M. Zou, V. Simplaceanu, N. T. Ho and C. Ho, Biochemistry, 1999, 38, 13423–13432 CrossRef CAS PubMed.
- A. Mozzarelli, L. Ronda, S. Faggiano, S. Bettati and S. Bruno, Blood Transfus., 2010, 8, s59–s68 Search PubMed.
- F. Meng, T. Kassa, S. Jana, F. Wood, X. Zhang, Y. Jia, F. D’Agnillo and A. I. Alayash, Bioconjugate Chem., 2018, 29, 1560–1575 CrossRef CAS PubMed.
- S. C. Schultz, B. Grady, F. Cole, I. Hamilton and K. Burhop, J. Lab. Clin. Med., 1993, 122, 301–308 CAS.
- D. H. Doherty, M. P. Doyle, S. R. Curry, R. J. Vali, T. J. Fattor, J. S. Olson and D. D. Lemon, Nat. Biotechnol., 1998, 16, 672–676 CrossRef CAS PubMed.
- R. Chatterjee, E. V. Welty, R. Y. Walder, S. L. Pruitt, P. H. Rogers, A. Arnone and J. A. Walder, J. Biol. Chem., 1986, 261, 9929–9937 CAS.
- E. Nagababu, S. Ramasamy, J. M. Rifkind, Y. Jia and A. I. Alayash, Biochemistry, 2002, 41, 7407–7415 CrossRef CAS PubMed.
- J. S. Jahr, M. Moallempour and J. C. Lim, Expert Opin. Biol. Ther., 2008, 8, 1425–1433 CrossRef CAS PubMed.
- R. Kluger, J. S. Foot and A. A. Vandersteen, Chem. Commun., 2010, 46, 1194–1202 RSC.
- K. D. Vandegriff, A. Malavalli, J. Wooldridge, J. Lohman and R. M. Winslow, Transfusion, 2003, 43, 509–516 CrossRef CAS PubMed.
- B. M. Manjula, A. Tsai, R. Upadhya, K. Perumalsamy, P. K. Smith, A. Malavalli, K. Vandegriff, R. M. Winslow, M. Intaglietta, M. Prabhakaran, J. M. Friedman and A. S. Acharya, Bioconjugate Chem., 2003, 14, 464–472 CrossRef CAS PubMed.
- D. Li, T. Hu, B. N. Manjula and S. A. Acharya, Bioconjugate Chem., 2009, 20, 2062–2070 CrossRef CAS PubMed.
- D. Tomita, T. Kimura, H. Hosaka, Y. Daijima, R. Haruki, K. Ludwig, C. Böttcher and T. Komatsu, Biomacromolecules, 2013, 14, 1816–1825 CrossRef CAS PubMed.
- R. Funaki, T. Kashima, W. Okamoto, S. Sakata, Y. Morita, M. Sakata and T. Komatsu, ACS Omega, 2019, 4, 3228–3233 CrossRef CAS.
- R. Haruki, T. Kimura, H. Iwasaki, K. Yamada, I. Kamiyama, M. Kohno, K. Taguchi, S. Nagao, T. Maruyama, M. Otagiri and T. Komatsu, Sci. Rep., 2015, 5, 12778 CrossRef CAS PubMed.
- H. Iwasaki, K. Yokomaku, M. Kureishi, K. Igarashi, R. Hashimoto, M. Kohno, M. Iwazaki, R. Haruki, M. Akiyama, K. Asai, Y. Nakamura, R. Funaki, Y. Morita and T. Komatsu, Artif. Cells, Nanomed., Biotechnol., 2018, 46, S621–S629 CrossRef PubMed.
- Y. Morita, T. Yamada, M. Kureishi, K. Kihira and T. Komatsu, J. Phys. Chem. B, 2018, 122, 12031–12039 CrossRef CAS PubMed.
- Y. Morita, K. Igarashi, R. Funaki and T. Komatsu, ChemBioChem, 2019, 20, 1684–1687 CrossRef CAS PubMed.
- R. Funaki, W. Okamoto, C. Endo, Y. Morita, K. Kihira and T. Komatsu, J. Mater. Chem. B, 2020, 8, 1139–1145 RSC.
- D. R. Grassetti and J. F. Murry Jr., Arch. Biochem. Biophys., 1967, 119, 41–49 CrossRef CAS PubMed.
- Y. Cheng, T. J. Shen, V. Simplaceanu and C. Ho, Biochemistry, 2002, 41, 11901–11913 CrossRef CAS PubMed.
- S. Y. Park, T. Yokoyama, N. Shibayama, Y. Shiro and J. R. Tame, J. Mol. Biol., 2006, 360, 690–701 CrossRef CAS PubMed.
- S. Sugio, A. Kashima, S. Mochizuki, M. Noda and K. Kobayashi, Protein Eng., 1999, 12, 439–446 CrossRef CAS PubMed.
- M. F. Perutz, J. E. Ladner, S. R. Simon and C. Ho, Biochemistry, 1974, 13, 2163–2173 CrossRef CAS PubMed.
-
Y. Aki-Jin, Y. Nagai, K. Imai and M. Nagai, in New Approaches in Biomedical Spectroscopy, ed. K. Kneipp, R. Aroca, H. Kneipp and E. Wentrup-Byrne, American Chemical Society, Washington, DC, 1st edn, 2007, ch. 19, vol. 963, pp. 297–311 Search PubMed.
- M. Nagai, S. Nagatomo, Y. Nagai, K. Ohkubo, K. Imai and T. Kitagawa, Biochemistry, 2012, 51, 5932–5941 CrossRef CAS PubMed.
- The cooperativity reduction was determined using the following equation, cooperativity reduction (%) = (nnative
Hb − n)/(nnative
Hb − 1.0) × 100, where nnative
Hb and n are Hill coefficient of native Hb and chemically modified Hb, respectively, and are measured in the same conditions. When the cooperativity is completely lost, the Hill coefficient becomes 1.0 and the cooperativity reduction reaches 100%.
- M. E. Wiltrout, J. L. Giovannelli, V. Simplaceanu, J. A. Lukin, N. T. Ho and C. Ho, Biochemistry, 2005, 44, 7207–72017 CrossRef CAS PubMed.
-
Desmond Molecular Dynamics System, D. E. Shaw Research, Maestro-Desmond Interoperability Tools, Schrödinger LLC, New York, NY, 2016.
-
Maestro, Schrödinger Release 2017-3, Schrödinger LLC, New York, NY, 2017.
- J. L. Banks, H. S. Beard, Y. Cao, A. E. Cho, W. Damm, R. Farid, A. K. Felts, T. A. Halgren, D. T. Mainz, J. R. Maple, R. Murphy, D. M. Philipp, M. P. Repasky, L. Y. Zhang, B. J. Berne, R. A. Friesner, E. Gallicchio and R. M. Levy, J. Comput. Chem., 2005, 26, 1752–1780 CrossRef CAS PubMed.
- Schrödinger Suite 2012 Protein Preparation Wizard, LLC, New York, NY, 2012; Impact version 5.8, Schrödinger, LLC, New York, NY, 2012.
- M. H. M. Olsson, C. R. Søndergard, M. Rostkowski and J. H. Jensen, J. Chem. Theory Comput., 2011, 7, 525–537 CrossRef CAS PubMed.
- W. L. Jorgensen, J. Chandrasekhar, J. D. Madura, R. W. Impey and M. L. Klein, J. Chem. Phys., 1983, 79, 926–935 CrossRef CAS.
- K. Yamada, K. Yokomaku, M. Kureishi, M. Akiyama, K. Kihira and T. Komatsu, Sci. Rep., 2016, 6, 36782 CrossRef CAS PubMed.
Footnote |
† Electronic supplementary information (ESI) available. See DOI: 10.1039/d0cb00056f |
|
This journal is © The Royal Society of Chemistry 2020 |
Click here to see how this site uses Cookies. View our privacy policy here.