Impacts of copper oxide nanoparticles on bell pepper (Capsicum annum L.) plants: a full life cycle study†
Received
1st August 2017
, Accepted 19th October 2017
First published on 19th October 2017
Abstract
Several studies have explored the effects of copper nanoparticles (NPs) on different edible plants. However, no studies on bell pepper (Capsicum annum L.) plants have been reported. In this study, plants were grown for a full life-cycle assessment (90 days of exposure) in natural soil amended with nano CuO (nCuO), bulk CuO (bCuO), and ionic copper (CuCl2) at 0, 125, 250, and 500 mg kg−1. Based on our experimental findings, none of the treatments significantly affected stem elongation, plant dry biomass, foliar area, leaf chlorophyll content, and fruit productivity of bell pepper. However, ionic copper significantly decreased the gas exchange parameters, evapotranspiration, stomatal conductance, and photosynthesis by an average of 41%, 59%, and 38%, respectively, compared to the other treatments at select concentrations (p ≤ 0.05). The ICP-OES data showed that, except for bCuO at 500 mg kg−1, at 250 mg kg−1 and above, the three compounds significantly increased root Cu (196%, 184%, and 184%) with respect to the control. Only at 500 mg kg−1, ionic Cu gave significantly higher root Cu compared to the other Cu treatments. Additionally, at 125 mg kg−1, leaf P was 41% lower for nCuO, compared to the bCuO treatment. At 500 mg kg−1, nCuO reduced Zn by 55% in leaves and 47% in fruits, compared to the control (p ≤ 0.05); however, it is premature to assert that the reduction in fruit Zn compromises the nutritional quality of bell pepper. Overall, this investigation showed that, at the concentrations tested, nCuO presented low toxicity to bell pepper, with rare differences between nano and bulk treatment responses.
Environmental significance
A clear understanding of the interaction of engineered nano-particles (NPs) with soil grown agricultural plants and their effect on food quality is incomplete. This study was undertaken to expand the library of qualitative and quantitative outcomes of terrestrial plants exposed to Cu-based NPs. The study is especially important since the number of full life cycle studies are limited and because it shows data from real agricultural soil. In the real world scenario, agricultural fields are amended with bio solids and effluents from wastewater treatment plants that likely carry nCuO. These NPs can be taken up by plants and possibly affect the quality of produce. The data collected showed that nCuO reduced several elements in chili fruits, which could degrade the fruit nutritional content.
|
Introduction
Engineered nanomaterials (ENMs) are one important class of chemicals being studied due to their effects on ecology and human health. ENMs have an ever rising application in industry for their unique technical properties.1 Among these, Cu-based nanoparticles (NPs) are used as additives in lubricants and printer ink, in the manufacture of semiconductors and lithium ion batteries, as heat insulators, and as catalysts.2–5 Recent estimates indicate that the world production of Cu-based NPs has reached 200 tons per year.6 Leaching and abrasion from various consumer products could make Cu-based NPs ubiquitous contaminants and present unknown risks of exposure and toxicity. Cu-based NPs also have agricultural applications due to their antimicrobial properties.4,7 In addition, the application of biosolids, wastewater, and sewage sludge to agricultural fields, as bio-fertilizers, presents a possible route of exposure to plants.8
There are limited number of studies on Cu-based NPs in plants. CuO NPs (100–2000 mg L−1) have shown toxicity to seed germination and root elongation in lettuce, radish, and cucumber.9 Another study illustrated reduced root length, moisture content, and dry biomass in lettuce plants exposed to 10 and 20 mg L−1 nano Cu/nano CuO (nCu/nCuO) in hydroponics.10 Zuverza-Mena et al.5 reported that nCuO, bulk/micro CuO (bCuO) and copper chloride (CuCl2) reduced cilantro (Coriandrum sativum) germination at both 20 and 80 mg of Cu per kg of soil but only bCuO reduced shoot elongation (at both concentrations) and chlorophyll content (at 20 mg kg−1). Shi et al.11 (at 1 mg L−1) and Lalau et al.12 (at 0.1, 1, and 10 g L−1) found similar results regarding inhibition of chlorophyll and plant growth in duckweed (Landoltia punctata) with nCuO. Studies conducted at the initial growth stage of different plant species grown under nCuO exposure (variable concentrations) indicated possible degradation of DNA and excessive production of reactive oxygen species (ROS).13–17 Hong et al.18 reported that nCuO reduced the root length at 5, 10, and 20 mg L−1 dosage and adversely affected the nutrient content and enzyme activity in alfalfa and cucumber plants.
Bell pepper (Capsicum annum L.) is a warm weather crop widely consumed all over the world. A phenolic compound, capsaicin (8-methyl-N-vanillyl-6-noneamide), gives it its pungent and chili flavor.19 Bell peppers are rich in carotenoids, vitamin C, and antioxidants. Through 2007–2011, California was the biggest producer (51%) of bell peppers in the US, followed by Florida (26%).20 The world production and hence the consumption of fresh bell peppers (along with Capsicum frutescen and Pimenta officinalis) has consistently risen at a rate of about 3% every year over the last decade, with world production of about 32 million tons in 2014.21
A limited number of studies have evaluated the full life cycle NP exposure of plants. To the authors' best knowledge, no phytotoxicological studies have been conducted with chili or bell pepper plants, especially in natural soil. This life cycle study was aimed to differentiate the physiological and agronomical effects of different particle size and species of Cu based chemicals on bell pepper plants. Treatment effects on ionome homeostasis and physiological and agronomical parameters were evaluated using biochemical and spectroscopic techniques. Through this research we intend to contribute to the existing body of work on food safety about the possible influences of engineered nanoparticles on the nutritional quality of produce.
Method and materials
Characterization of copper-based chemicals
Nano-particulate copper oxide, bulk copper oxide, and reagent grade copper chloride salt were obtained from Sigma Aldrich (St. Louis, MO). The physico-chemical properties, zeta potential, hydrodynamic diameter, copper content by weight, morphology and additional properties are shown in Table 1.
Table 1 Characterization of nCuO and bCuO particles used in this study18,81
Property |
nCuO |
bCuO |
Measurement was performed at pH 7.
|
Primary particle size (nm) |
20–100 |
200–2000 |
Hydrodynamic diametera (nm) |
280 ± 15 |
376 ± 26 |
Zeta potential (mV)a |
−34.4 ± 0.5 |
−42.7 ± 0.153 |
Cu content (wt%) |
74.3 |
79.7 |
Purity (%) |
88.3 ± 1.3 |
92.8 ± 1.1 |
Other elements present |
O, C |
O |
Shape, morphology |
Rhombus, irregular |
Prism, irregular |
Main copper phase |
CuO |
CuO |
Crystal structure |
Monoclinic |
Monoclinic |
Soil and treatments
The plants were grown in agricultural soil collected at a field in Socorro, TX (latitude: N 31°41′ 30.705′′ and longitude: W 106°17′ 13.936′′, elevation: 1115 m asl). A Malvern Mastersizer Hybrid 2000G (Malvern, Worcestershire, UK) was used to characterize the soil, which was silty loam (65% silt, 20% sand, and 15% clay).22 The soil pH in DI water and in a CaCl2 solution slurry was 7.4 and 7.5, respectively. The pots for transplantation were prepared by amending the soil with nCuO, bCuO, and CuCl2 at 0 mg kg−1 (used as control), 62.5, 125, 250 and 500 mg of Cu per kg of soil, four replicates each and one plant per pot. Each pot contained 5 kg natural soil homogenized with an aqueous suspension/solution of the copper-based chemicals, prepared by stirring in DI water for 30 min. The copper chemicals were suspended/diluted in enough water to have the soil at about 60% of the field capacity. While preparing the substrate, the contaminant homogeneity was ensured by thorough manual mixing of the soil with the chemical suspension for 20 min.
The concentration range 62.5–500 mg kg−1 was selected for its environmental relevance. As per USGS,23 the average copper concentration in various soils is about 30 mg kg−1 dry weight. The Cu concentration in pesticides is up to 50 mg kg−1, about 300 mg kg−1 in fertilizers, and up to 3000 ppm dry weight in sludge. Fly ash has Cu concentrations ranging from 300 mg kg−1 to about 2000 mg kg−1.24 The concentration range most pertinent to agricultural fields was picked. Additionally, similar concentrations have been used in previous investigations.
Copper release in soil solution
Copper concentration in soil solution was determined for nCuO and bCuO species. Copper chloride was not considered in the analysis because it is readily soluble in water. Three replicates each with 20 g of the experimental soil mixed with the chemical and 30 mL water to make a 500 mg kg−1 aqueous suspension were shaken for 12, 24, 36, and 48 h. The samples were stationed upright for 4 h after rocking. Thereafter, 12 mL of the supernatant was separated and centrifuged at 5000 rpm for 15 min (Sorvall, Legend XR1 centrifuge, Thermo Fisher Scientific, Waltham, MA, USA). Ten milliliters (10 mL) of the subsequent supernatant was decanted and centrifuged again at 5000 rpm for 30 min. Another round of centrifugation was carried out with 8 mL of the resultant supernatant at 15
000 rpm for 30 minutes. The process was repeated thrice in order to filter out the particles in suspension and contain just the dissolved chemical (ions) in solution. The final 5 mL of the soil solution was analyzed by ICP-OES for the concentration of dissolved copper in each sample.14,25,26
Standard reference material 1570a from the National Institute of Standards and Technology was used to validate the digestion method, obtaining a recovery of 100% ± 5.0%. For quality control of the ICP readings, every 25 samples, a blank and a spiked sample containing Cu 10 mg L−1 were read. The average reading for Cu in the spiked sample was 9.8 ± 0.40 mg L−1. The ICP-OES parameters used were as follows: nebulizer flow, 0.80 L min−1; power, 1400 W; peristaltic pump rate, 1.5 mL min−1; flush time, 20 s; delay time, 20 s; read time, 10 s; and wash time, 60 s.
Plant culture at the greenhouse
Bell pepper seeds (California wonder 300 TMR) were planted in a Sunshine Ready Earth REPS soil mix (55–65% Canadian sphagnum peat moss, vermiculite, dolomite, lime). Thirty (30) days after growth in a controlled greenhouse environment, the seedlings were transferred to pots with copper treated soil. Throughout the growth period, the plants were regularly watered with a nutrient solution containing NPK in the ratio 15–5–15 at 1 g L−1; the pH of the solution was maintained at 5.8 and the electrical conductivity (EC) at 1.0 mS cm−1. The plants were treated with the pesticide Avid 0.15 EC, whose active ingredient is abamectin (2% v/v), to protect the plant against aphid and white fly infestation. Throughout the experiment, the average day temperature at the green house was recorded to be 27.2 ± 1.6 °C and the average night temperature to be 25 ± 2.1 °C. The average daily light integral was recorded to be 10.1 ± 3.1 mol m−2 d−1. The relative humidity at the greenhouse was recorded to be 47.1 ± 10.6% for the experiment.
Gas exchange and chlorophyll measurement
The gas exchange data was collected by using a CIRAS-3 portable photosynthesis system (PP Systems, Amesbury, Massachusetts, USA) just prior to harvest at 90 days post transplantation. The photosynthetic rate, stomatal conductance, and evapotranspiration were measured in the newest fully expanded leaf of each plant. The plants were well-hydrated before measurements were taken between 1000 to 1400 h on sunny days, ensuring no cloud cover that could influence the readings.27 In addition, the relative chlorophyll content was determined using a hand held single photon avalanche diode chlorophyll meter (SPAD, Minolta Camera, Japan) by taking the average value across ten leaves per plant.
Harvest and elemental analysis
Ninety (90) days post transplantation, the plants were mature at the fruiting stage. The total foliar area, total number of fruits, and their total weight were recorded for each plant. The total foliar area was measured using a LI-3100C area meter (LI-COR Biosciences, Lincoln, Nebraska, USA). The separated samples were washed in 0.01 M HNO3 followed by DI water. After air drying, the fresh samples were oven dried at 60–70 °C for 72 h.
The dried plant samples were weighed to determine the dry biomass. Samples were ground and the powders were digested in a digiPrep hot block (SCP Science, Quebec, Canada) for further analysis. The Environmental Protection Agency (EPA) method 3051 was employed for digestion using plasma pure nitric acid (65%) and hydrogen peroxide (30%) (1
:
4). Inductively coupled plasma-optical emission spectroscopy (ICP-OES, Perkin-Elmer Optima 4300 DV, Shelton, CT) was used to determine the macro and micro element (Ca, K, Mg, P, S, Fe, Zn, Cu, Mn, Ni, Mo) concentrations in the digested samples.5 Three blank sample carriers, two carriers with spinach as the standard reference material (NIST 1570a, Gaithersburg, MD) and three with samples spiked with 20 mg L−1 Cu NPs were all digested and included in the analytical process as part of QA/QC.18
Statistical analysis
All the data collected were analyzed using the SPSS (IMB) program. The mean values from the controls and the treatments were compared among each other using one-way ANOVA followed by the Tukey–Kramer multiple comparison test (SPSS 19.0 package, Chicago, IL). Results are presented as mean ± standard error and were observed for statistically significant differences at p ≤ 0.05.
Results and discussion
Soil dissolution study
The dissolution characteristics of nano and bulk species of CuO in soil solution are shown in Fig. 1. The pristine Cu content present in the agricultural soil (obtained from control) has been deducted off of the measured values from the treatments to give us the absolute Cu ion concentrations (Fig. 1). As seen in the figure, nCuO particles favored Cu dissolution in the soil environment over the bulk species. The ionic Cu content in soil solution from nCuO was 388%, 230%, 152%, and 188%, greater than bCuO at 12, 24, 36, and 48 h, respectively (p ≤ 0.05) (Fig. 1). The concentration of ionic Cu consistently increased over time for the two chemicals, more rapidly for nCuO than for bCuO. The Cu concentration in soil solution from nCuO at 48 h was 20% greater than that at 36 h, which in turn, was 31% greater than that at 24 h (p ≤ 0.05). The Cu concentration in soil solution from bCuO at 48 h was 81% higher than that at 24 h, and at 36 h, it was 219% greater than that at 12 h of dissolution (p ≤ 0.05). Some studies have shown the dissolution of Cu from bCuO and nCuO in different media. For instance, Dimkpa et al.14 reported no differences in Cu released from both CuO compounds suspended in deionized water and added to sand for a period of 14 days. In another similar study, Gunawan et al.28 found that in Luria-Bertani medium, there was a significantly higher leaching of Cu from nCuO, compared to bCuO. Gunawan et al.28 suggested that the curvature of nCuO favored the Cu dissolution. The fact that there are very few such studies in soil solution makes comparison difficult. However, McShane et al.29 reported that in two different soils, nCuO showed significantly higher Cu activity than bCuO, which in some ways supports the results found in the current study.
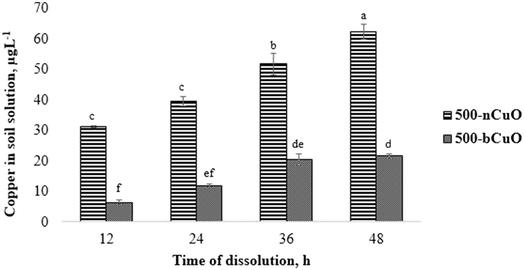 |
| Fig. 1 Relative dissolution of nCuO and bCuO in soil solution in DI water, deducting the pristine copper present in natural soil. Data are averages of 3 replicates ± SE. Averages with a common letter are not significantly different with respect to each other, as per one-way ANOVA and the Tukey test (p ≤ 0.05). | |
Referring to Table 1, we see that there is a difference of orders of magnitude between the nCuO and bCuO primary particle size, with nCuO being much smaller. A smaller particle size offers a much larger specific surface area for contact with water.30 This could be a reason for observing the difference in the Cu dissolvability between the two Cu species (Fig. 1). Similarly, the hydrodynamic diameter was relatively smaller for nCuO against bCuO, which may influence Cu dissolution.
Chlorophyll content
The chlorophyll measurements were made just prior to harvest. The differences from SPAD measurements were not statistically significant, indicating that the chemical treatments had no significant influence on the chlorophyll content in bell pepper plants.
Gas exchange
The gas exchange data including evapotranspiration (E), stomatal conductance (gs), and photosynthesis (Pn) are shown in Fig. 2. All the three markers of gas exchange displayed similar results and none of the treatments produced significant differences with respect to control. However, a comparison between compounds showed that ionic Cu displayed significant reduction in E, gs, and Pn, compared to the other treatments. As shown in Fig. 2(a–c), at 62.5 mg kg−1, ionic Cu reduced Pn by 38%, and E by 44%, compared with nCuO, and by 39%, compared with bCuO (p ≤ 0.05). While at 125 and 500 mg kg−1, it reduced E by about 40% and gs by 58% and 59%, respectively, compared to bCuO (p ≤ 0.05). Moreover, at 62.5 and 250 mg kg−1, ionic Cu reduced gs by 65% and 55%, compared with nCuO (p ≤ 0.05).
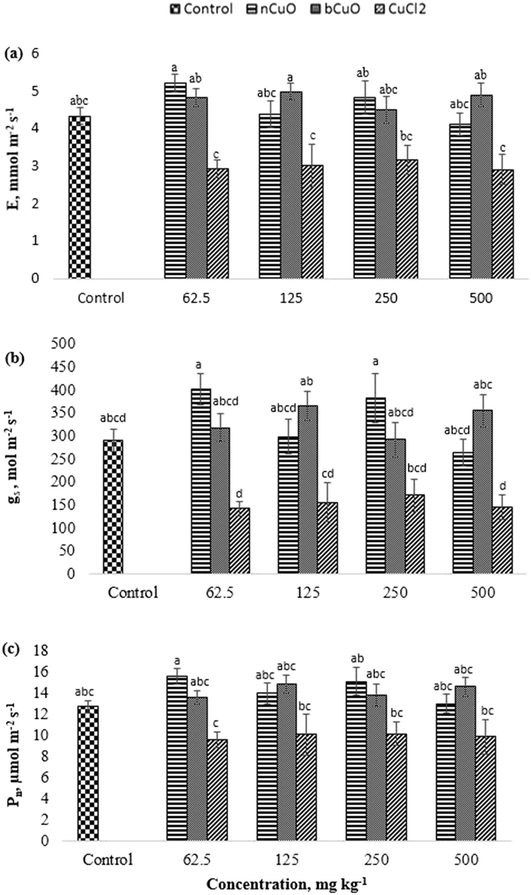 |
| Fig. 2 Comparison of the gas exchange parameters (a) evapotranspiration, (b) stomatal conductance, and (c) photosynthesis in bell pepper plants cultivated for 90 days in natural soil amended with nCuO, bCuO, and CuCl2 at 0, 62.5, 125, 250, and 500 mg Cu per kg. Data are averages of 4 replicates ± SE. Averages with a common letter are not significantly different with respect to each other, as per one-way ANOVA and the Tukey test (p ≤ 0.05). | |
The negative responses observed in ionic treatments, mainly at 500 mg kg−1, could be due to the higher uptake and translocation of Cu to the leaves (Fig. 2). The response at lower concentrations could be due to the rapid interaction of Cu+/Cu++ or Cl− with the plants, since both ions were available for plant uptake at a very early growth stage. Chloride has been shown to be toxic to several plants including tomatoes, beans, and barley, among others.31 On the other hand, bulk- and nano-CuO exhibited a slower release of copper ions into soil (Fig. 1), which likely led to slower Cu uptake and translocation.32 Julich and Gäth33 conducted a sorption study to compare the behavior of CuO NPs vs. ionic Cu in different soil types. They observed that nCuO had a much stronger tendency to bind with different soil fractions, compared to ionic Cu species. In the current study, the concentration of Cu ions released from bCuO was significantly different than that from nCuO, per the soil dissolution study (Fig. 1); however, they had similar effects on the gas exchange parameters. The gas exchange measurement was taken just one time over the growth period of the plant, just prior to harvest. The results could be the cumulative effect of the exposure to the chemicals over the growth period, especially to the ionic Cu treatment.
Sekine et al.34 reported that Cu-based NPs dissolve in soil, and independent of the Cu form added to the soil, in the long term, the Cu species from dissolved Cu and Cu-based NPs were the same in different soils. This could have happened in our work, since the effects of the Cu form on most measured parameters were low.
Copper uptake and translocation
Copper uptake.
The experimental soil had 17.34 ± 0.53 mg of total natural Cu per kg soil (Table S6, ESI†). The concentration of Cu in bell pepper roots is shown in Fig. 3(c). All Cu treated plants had significantly more Cu in the root, compared with the control. Similar results have been reported in previous studies with Bromus carinatus (Hook. and Arn.), corn, cucumber, radish, rapeseed, ryegrass, wheat, mung bean, duckweeds, and lettuce.10,11,35–37 In bell pepper, however, the differences were statistically significant only at higher concentrations such as 250 and 500 mg kg−1, except for bCuO at 500 mg kg−1. Increases at 250 mg kg−1 were about 196, 184, and 186%, respectively, for nCuO, bCuO, and ionic Cu, compared with control (p ≤ 0.05). Interestingly, there were no differences between compounds at 250 and 500 mg kg−1, except for ionic copper at 500 mg kg−1. At this concentration, roots exposed to ionic copper had about 88% more Cu than that at 250 mg kg−1. At 500 mg kg−1 nCuO and ionic Cu, root Cu was higher by 239% and 437%, with respect to the control (p ≤ 0.05).
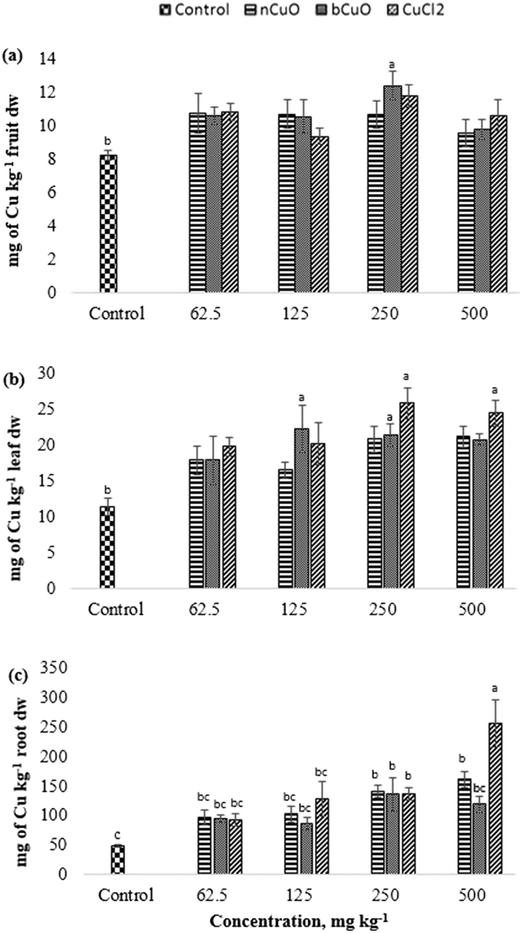 |
| Fig. 3 Copper concentration in the (a) fruit, (b) leaf and (c) root of bell pepper plants cultivated for 90 days in soil amended with nCuO, bCuO, and CuCl2 at 0 (control)–500 mg of Cu per kg of soil. Data are averages of 4 replicates ± SE. Averages with a common letter are not significantly different with respect to each other, as per one-way ANOVA and the Tukey test (p ≤ 0.05). | |
Several factors determine the accumulation of Cu in roots including the added concentration, soil properties, plant species, and root morphology.38 Metallothionein and phytochelatins are important organic complexes likely formed in plant root cells because of which, Cu, especially from nCuO, is retained in the root.39 Our observations of the Cu in roots are contrary to what has been reported from sand grown wheat and soil grown cilantro.5,14 The authors apparently did not see a significant variation in Cu concentration in root samples under nCuO treatment. It is very likely that variations in the response are due to differences pertaining to the plant species and the experimental design. Additionally, soft metal cation NPs are strongly affected by inorganic and organic ligands40 that are abundant in agricultural soils such as the one used in this study.
Copper translocation to leaves and fruits.
The concentration of Cu in leaves and fruits are shown in Fig. 3(a and b), respectively. No significant differences were observed between the responses from nCuO and bCuO treatments, at any concentration. At 125 and 250 mg kg−1, bCuO showed higher leaf Cu concentration than the control (97% and 89%), while ionic copper showed increased leaf Cu at 250 and 500 mg kg−1 (129% and 116%), compared to the control (p ≤ 0.05). In fruits, only bCuO at 250 mg kg−1 showed higher Cu concentration (51%) compared with the control. The result observed with bCuO was probably due to its larger negative zeta potential, compared to nCuO (Table 1). The soil predominantly had a negative charge as did the CuO particles. The apparent repulsion between the two negative charges probably fueled the translocation of bCuO at certain concentrations.41 Moreover, nCuO probably underwent aggregation in the rhizosphere and root tissues, thus resulting in a different behaviour than bCuO.42–45 Under the same pH conditions, aggregation is higher for smaller particles.43
The translocation factors for Cu (TF, ratio of the elemental concentrations between leaf and root) are shown in Table S7, ESI.† Among all the Cu treatments, 125 mg kg−1 bCuO gave the highest TF, followed by the control and the lowest TF was given by 500 mg kg−1 CuCl2. Bulk CuO allowed faster Cu translocation to fruits, apparently because of its relatively high negative charge (Table 1) and the soil negativity.41 A low TF for 500 mg kg−1 CuCl2 needs further work for an explanation. Cu is an element with low mobility in soil,46 but forms very strongly bound chelates.34,47 The later fact tends to magnify for the nano species, since they tend to homoaggregate, along with heteroaggregation.40 Further translocation of Cu takes place from the root to the fruit. Since none of the treatments affected fruit Cu (8–12 mg kg−1) and the Cu dietary reference intake is 700–1000 μg per day (Food and Nutrition Board, Institute of Medicine),48 this suggests that none of the treatments compromised Cu nutrition through bell peppers exposed to nCuO, at the concentration tested, in a similar soil like the one used in this study.
The gas exchange responses from treatments (Fig. 2) did not align with the leaf Cu accumulation (Fig. 3b). Leaf Cu concentration from both the bCuO and ionic Cu treatments significantly increased, at varying concentrations. On the contrary, under similar Cu content from varying concentrations, bCuO/nCuO gave significantly lower gas exchange compared to the ionic treatment. This probably occurred because gas exchange is influenced by other factors, besides Cu concentration. Zhao et al.27 observed no significant changes in gas exchange in CeO2 treated cucumber plants. They conjectured that the chemical adsorbed on the root surface exerted influence on water transport through the plant system. It is possible that similar phenomena occurred in bell pepper. Nano CuO aggregates in the soil rhizosphere.34,40,49
Absorption of essential elements
Out of all the elements analyzed from root, leaf, and fruit samples, only those with significant differences (p ≤ 0.05), compared with the control and among treatments, are discussed. The three Cu compounds were observed to have varying effects on the level of nutrients in plant tissues.
Root elemental content
Fig. 4 shows the concentration of elements that were altered in roots including phosphorus, sulfur, selenium, and molybdenum. Table S6 of the ESI† gives the background concentration of all the elements in native soil for reference.
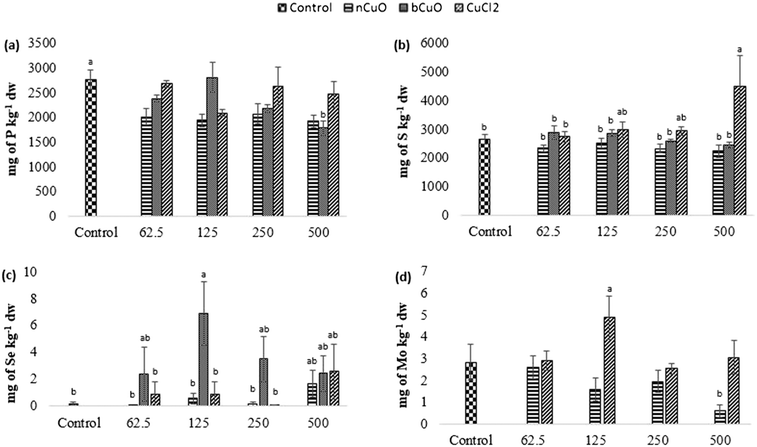 |
| Fig. 4 Concentration of (a) P, (b) S, (c) Se, and (d) Mo in root samples of bell pepper plants cultivated for 90 days in natural soil amended with nCuO, bCuO, and CuCl2 at 0 (control)–500 mg of Cu per kg of soil. Data are averages of 4 replicates ± SE. Averages with a common letter are not significantly different with respect to each other, as per one-way ANOVA and the Tukey test (p ≤ 0.05). | |
Phosphorus.
As shown in Fig. 4(a), only bCuO at 500 mg kg−1 altered P absorption in the root, which was reduced by 36%, with respect to the control (p ≤ 0.05). As explained before, the negativity of soil particles41 could force the movement of the negative bCuO to the roots, which can physically block the uptake of some elements. In addition, slowly released Cu ions from bCuO14 at the root–surface interface could be complexed with phosphate ions (H2PO4−, HPO42−), making P non-bioavailable for the plants.5 Hong et al.18 reported a reduction of root P in lettuce and alfalfa plants exposed to bCuO and nCuO. Ali et al.50 had similar observations for the roots of maize plants exposed to Cu. Safaya51 found an antagonistic effect between copper and phosphorus as nutrient elements in corn plants.
Sulfur.
The sulfur concentration is shown in Fig. 4(b). As shown in this figure, only ionic copper at the highest concentration (500 mg kg−1) altered S in root tissues, which increased by 71% with respect to the control (p ≤ 0.05). Sulfate transporters Sultr 1;1 and Sultr 1;2 were likely expressed in the root at 500 mg kg−1 ionic copper.52,53 Sulfur is the main component of organic protein complexes, like metallothionein and phytochelatins in plant systems. These complexes are the plant's response to metal toxicity and play a vital role in cellular detoxification and metabolism.54 Cu from ionic copper most likely formed copper sulfate or organic complexes in the soil,31 which were taken up by the roots and translocated further to the leaves and fruits.55
Selenium.
The concentration of Se in the bell pepper root is shown in Fig. 4(c). As illustrated in Fig. 4(c), there was a great variability in Se accumulation; however, only bCuO at 125 mg kg−1 showed statistically significant differences, compared to the other treatments. Root Se showed an inverted U shape profile, but in all cases, there were large error bars, which overlapped the response with the other treatments. Se in root samples at 125 mg kg−1 bCuO was higher by 3469%, compared with control, and by 1048%, compared with nCuO at the same concentration (p ≤ 0.05). Se is a non-essential element for plants.56 It is chemically very similar to sulfur; hence, they share metabolic pathways and transporters.57
Molybdenum.
The concentration of Mo in roots of bell pepper are shown in Fig. 4(d). As shown in this figure, Mo was not detected in plants exposed to bCuO, at all concentrations (IDL: 37.8 μg kg−1). Mo is taken by plants as MoO42−,58 and bCuO had a high negative charge (−42.7 mV) that, very likely, repelled molybdate from the root surface. This could also have happened with nCuO at 500 mg kg−1, which significantly reduced (about 88%) Mo uptake, compared with the 125 mg kg−1 of ionic Cu treatment. Additionally, Mo is said to share some transporters with S and P.58 Mo uptake and transport at 125 mg kg−1 ionic copper treatment was probably supported by the P transporters because the P transport for the same treatment was not significant in the root.59,60
Leaf and fruit section
The concentration of elements altered in leaves and fruits is shown in Fig. 5 and 6 including the macro elements calcium, magnesium, phosphorus, and sulfur and the microelements nickel and zinc.
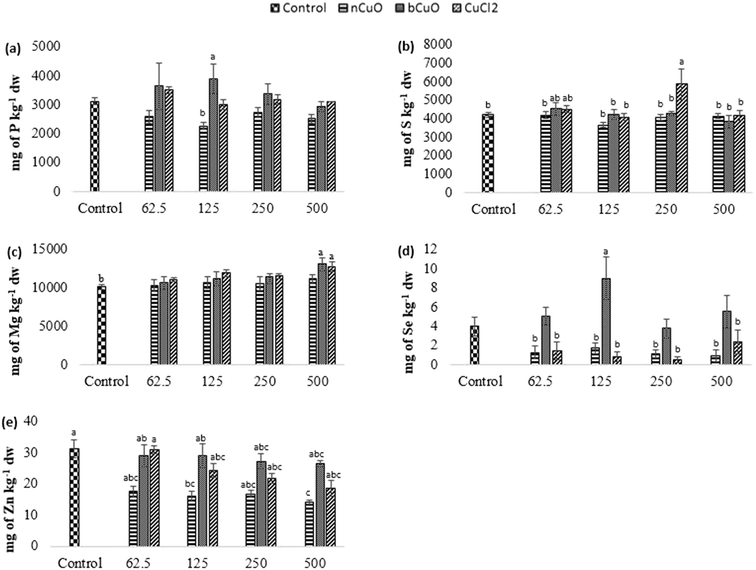 |
| Fig. 5 Concentration of (a) P, (b) S, (c) Mg, (d) Se, and (e) Zn in leaf samples of bell pepper plants cultivated for 90 days in natural soil amended with nCuO, bCuO, and CuCl2 at 0 (control)–500 mg of Cu per kg of soil. Data are averages of 4 replicates ± SE. Averages with a common letter are not significantly different with respect to each other, as per one-way ANOVA and the Tukey test (p ≤ 0.05). | |
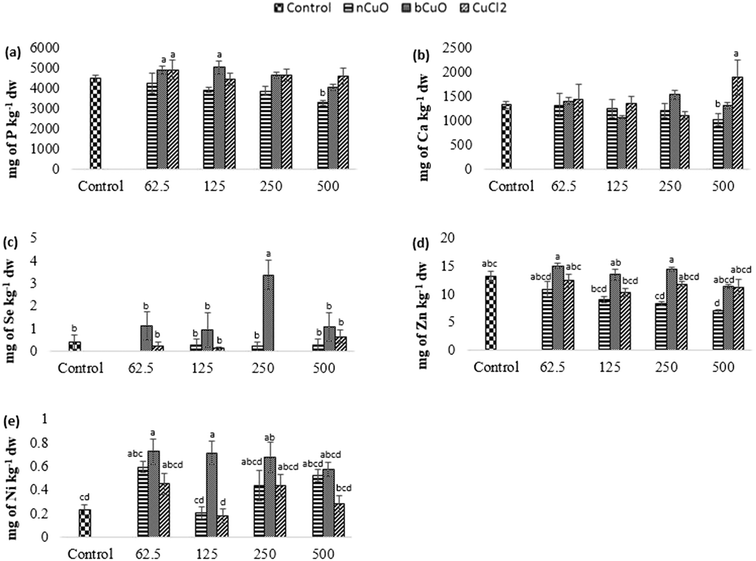 |
| Fig. 6 Concentration of (a) P, (b) Ca, (c) Se, (d) Zn, and (e) Ni in fruit samples of bell pepper plants cultivated for 90 days in natural soil amended with nCuO, bCuO, and CuCl2 at 0 (control)–500 mg of Cu per kg of soil. Data are averages of 4 replicates ± SE. Averages with a common letter are not significantly different with respect to each other, as per one-way ANOVA and the Tukey test (p ≤ 0.05). | |
Calcium.
The concentrations of Ca2+ in the fruit samples are shown in Fig. 6(b). As illustrated in the figure, no significant differences were observed at any of the treatment concentrations with respect to control. However, the Cu compounds affected in a different way the concentration of Ca and other elements in the aboveground plant parts. At 500 mg kg−1, the Ca2+ content in the fruit samples was found to be significantly higher (85%) with ionic Cu, compared to nCuO treatment (p ≤ 0.05). Copper apparently shares transporters with Ca2+ that fall under heavy metal ATPases family.61 Lysosomal cystine transporter (LCT)-1 of the ABC transporters was found to transport Ca2+ along with heavy metals in wheat plants.62 A study conducted to assess the interaction between Cu and Ca2+ in soil grown Norway spruce seedlings had results contrary to ours. Ionic copper treated plants had lower Ca2+ content in the root and bark of the plants compared to the control.63 Also, ionic copper treated sand grown cucumber plants64 and Cu treated hydroponically grown lettuce10 had lower Ca2+ in the leaf compared to the control. However, in the current study, ionic copper showed much Ca2+ in fruits, compared to nCuO, which suggest an effect of particles.
Magnesium.
Accumulation of Mg2+ in leaves is shown in Fig. 5(c). Bulk CuO and ionic copper significantly increased leaf Mg. At 500 mg kg−1 bCuO and ionic copper treatments, leaf Mg was elevated by 30% and 26%, compared with control (p ≤ 0.05). No response was observed from nCuO treatments. Alaoui-Sossé et al.64 and Trujillo-Reyes et al.10 found converse responses in sand grown cucumber and hydroponically grown lettuce. These researchers reported lower Mg2+ in plant leaves, compared with controls. It has been reported that Mg2+ counters Cu toxicity in grapevine, wheat, barley, and cowpea by likely taking up the Cu binding sites.65,66 On the other hand, Cu treated oregano plants were found to have higher Mg2+ than the control in the leaves,67 coinciding with our results. Magnesium has a role in maintaining the permeability of cell membranes.68 At high concentrations, Cu probably disturbed cell membranes and caused an influx of Mg2+ within the plant cells.69
The cation exchange capacity of the experimental soil was estimated to be between 15–30 meq per 100 g, since it was silty loam, with a pH of 7.4. This cation exchange capacity could be affecting the uptake and translocation of Ca2+ as well as Mg2+,70 under Cu treatment. Cu has a very high affinity for organic matter, clay, and sulfurous sites in soil or sediments.40–55 Copper tends to replace the adsorption positions taken up by Ca2+ and Mg2+, especially in organic matter, and the two ions are released into the soil solution. This is apparently why there was (Fig. 5(c)) significantly high leaf Mg at 500 mg kg−1 bCuO and ionic Cu treatments, compared with control. Fig. 6(b) shows similar results with higher fruit Ca2+ for 500 mg kg−1 ionic Cu treatment, compared to nCuO.
Phosphorus.
The phosphorus levels in the leaf and fruit tissues of bell pepper plants are given in Fig. 5(a) and 6(a). As shown in the figures, leaf and fruit P contents were not significantly different with respect to the control (p ≤ 0.05). However, at 125 mg kg−1, the P concentration in leaves was lower for nCuO by 42%, compared with bCuO (p ≤ 0.05) (Fig. 5(a)). In fruit samples, the P concentration at 500 mg kg−1 nCuO was found to be significantly lower than bCuO at 62.5 and 125 mg kg−1 (33%, 35%) and lower than ionic Cu at 62.5 mg kg−1 (33%) (p ≤ 0.05) (Fig. 6(a)). Bulk CuO markedly reduced the uptake of P in the root at the highest concentration, but it was apparently not that inhibitory at the lower concentrations, which enabled P translocation to leaves and fruits. Conversely, nCuO had a significantly inhibitory effect on P translocation up to the leaf and fruit. This was probably because of its aggregation characteristics40–42 and comparatively better Cu2+ ion release in soil solution, compared to bCuO, as mentioned in the particle dissolution study (Fig. 1). The finding for P in the leaf coincides with that of Hong et al.18 for P in hydroponically grown alfalfa shoots, where they saw a dose dependent increase in the P concentration in bCuO treated samples. The Pht2 transporter is likely responsible for the translocation of P from the root up through the stem to the leaf and fruit.18 Therefore, it seems that 125 mg kg−1 bCuO and nCuO altered the gene expression for the P transporter; bCuO increased it, while nCuO reduced it.
Additionally, the ionic Cu treatment did not affect P uptake and translocation at any concentrations. Most likely, the instant formation of Cu ions in solution in the ionic treatment initiated its binding with the available inorganic phosphate in soil. Cu3 (PO4)2 is an insoluble compound that occludes the available phosphate in alkaline soil (the pH of the experimental soil is 7.4, Table S5†).71
Sulfur.
The concentrations of S in bell pepper leaf samples are shown in Fig. 5(b). As seen in this figure, ionic copper at 250 mg kg−1 increased S by 40%, with respect to the control and by 45% with respect to 250 mg kg−1 nCuO treatment (p ≤ 0.05) (Fig. 5(b)). Ionic copper treatment was more responsive since the ionic form is more easily bioavailable to the plant.31 At 250 mg kg−1 ionic Cu, S was significantly translocated to the leaf, compared to 500 mg kg−1 in the root. Sulfur was probably elevated at 250 mg kg−1 ionic Cu even in the root, but not significantly. Sulfate transporters Sultr 2;1 and Sultr 3;5 are responsible for sulfate translocation from the root to the aerial parts.53–72 Shahbaz et al.73 observed an increased sulfate content in the shoot of the Chinese cabbage plant at high ionic copper concentration with a similar transporter effect. It could be possible that Cu ions bonded with sulfate in the soil and were translocated as CuSO4 from the root to the leaves, thus, elevating the S content.
Selenium.
The Se concentration in leaf and fruit samples are shown in Fig. 5(d) and 6(c). There were no significant differences observed for any of the treatments in the leaf Se concentrations, compared with the control. However, there were significant differences between treatments. At 125 mg kg−1, Se from bCuO treatment was significantly higher by 431%, with respect to that from nCuO (p ≤ 0.05) (Fig. 5(d)). On the other hand, there were no significant responses from ionic Cu or nCuO treatments, individually, both in leaves and fruits. However, at 250 mg kg−1, fruit Se in bCuO treatment was higher by 1570% with respect to the corresponding nCuO treatment and was higher than the control by 731% (p ≤ 0.05) (Fig. 6(c)). As mentioned before, bCuO has a larger negativity, compared with the other two treatments (Table 1). The negatively charged soil could be repelling the compound promoting its uptake and translocation through the plant,41 thus, affecting Se translocation. The Sultr1 transporter is apparently responsible for the translocation of the absorbed selenate ions from the root through the stem to the leaves and to the fruit.72
Nickel.
The fruit Ni concentrations for the different treatments are shown in Fig. 6(e). The concentrations of Ni2+ at 62.5, 125 and 250 mg kg−1 bCuO treatments were significantly higher than the control by 159%, 136%, and 202%, respectively (p ≤ 0.05). There were no changes observed in fruit Ni2+ concentration under nCuO and ionic Cu treatments individually. An important observation was made at 125 mg kg−1, where the level of nickel in the fruit samples was significantly higher in bCuO treatment than in nCuO by 95% (p ≤ 0.05) (Fig. 6(e)). Cataldo et al.74 pointed out that Ni2+ tends to form organic complexes once it enters the plant system; the complexes are carried through the xylem and deposited in the leaf and the seed of the soybean plant. This coincides with our finding where the presence of Ni2+ was observed in the fruit only.
Zinc.
The concentrations of Zn in leaf and fruit samples are shown in Fig. 5(e) and 6(d). A distinct antagonistic effect between Zn and Cu was observed in fruit and leaf samples. The Zn content in the leaf samples at 500 mg kg−1 nCuO was found to be significantly lower than those of the control (55%) and bCuO treatments at 62.5 and 125 mg kg−1 (52% each), and lower than that of ionic Cu at 62.5 mg kg−1 (55%) (p ≤ 0.05) (Fig. 5(e)). Moreover, in the fruit samples Zn levels for 500 mg kg−1 nCuO treatment were significantly lower than that of control (47%), lower than those of 62.5, 125 and 250 mg kg−1 bCuO (54%, 48%, 51%) and lower than that of 62.5 mg kg−1 ionic Cu treatment (44%) (p ≤ 0.05). (Fig. 6(d)). Once again, we observed a nutrient inhibitory effect under nCuO exposure and a nutrient promoting effect under bCuO and ionic Cu exposure. These observations in shoots were in agreement with studies conducted by Kabata-Pendias and Pendias,75 Lastra et al.,76 Beckett and Davis,77 and Safaya.51 In a study conducted to assess the fertilization qualities of Zn in different rice varieties, it was observed that Cu and Zn had an antagonistic effect on each other, especially at the primary absorption site, negatively affecting Zn's translocation.78 Cu treatment has been found to reduce Zn uptake in wheat and rice.79,80 Zuverza-Mena et al.5 reported that Zn was hampered under high nCuO concentrations in cilantro shoots.
The recommended dietary intake for Zn, according to the Food and Nutrition Board, Institute of Medicine,48 is 8–11 mg per day. For the fruit data, the lowest observed value under the treatments was 7 mg kg−1 (Fig. 6(d)). This could indicate a low Zn content; however, it is premature to assure that the reduction in fruit Zn compromises bell pepper nutritional quality.
Effect of treatments in fruit production
The total mass of the fruit collected per plant, average weight of fruits per plant, the total number of fruits collected per plant and their averages per treatment were determined and analyzed. None of the fruit production parameters showed a statistically significant difference at any concentration (p ≤ 0.05).
Dry biomass production and foliar area
The root, stem, and leaf dry biomass data are shown in the ESI† (Fig. S1). There were no statistically significant differences in the dry biomass collected and the foliar area (Fig. S2†) observed from the different treatments (p < 0.05).
Conclusions
Although none of the treatments affected leaf chlorophyll, the gas exchange results showed that the ionic copper treatment had a significant deteriorating effect on evapotranspiration, photosynthesis, and stomatal conductance. As in the previous studies, Cu tends to remain in bell pepper roots, independent of the compound; however, there were variable effects on nutrient accumulation. Bulk CuO treatment bolstered the nutrient uptake and translocation at certain concentrations of most elements in the plant system, while nCuO mostly had an inhibitory effect. Interestingly, under ionic Cu treatment, the plant response to nutrient uptake was almost the same as that under bCuO treatment. The plant biomass and fruit production data did not show any significant variations under the three treatments. With the exception of nCuO at 500 mg kg−1 that reduced Zn, compared with the control, none of the concentrations reduced the absorption of essential elements in fruits. Overall, nCuO had an inhibitory effect on the uptake of important elements but did not show visible signs of toxicity or effects in plant productivity.
Conflicts of interest
There are no conflicts to declare.
Acknowledgements
This material is based upon work supported by the National Science Foundation and the Environmental Protection Agency under Cooperative Agreement Number DBI-1266377. Any opinions, findings, and conclusions or recommendations expressed in this material are those of the author(s) and do not necessarily reflect the views of the National Science Foundation or the Environmental Protection Agency. This work has not been subjected to EPA review and no official endorsement should be inferred. The authors also acknowledge the USDA grant 2016-67021-24985 and the NSF Grants EEC-1449500, CHE-0840525, DBI-1429708, and USDA Hatch project TEX090450. Partial funding was provided by the NSF ERC on Nanotechnology-Enabled Water Treatment (EEC-1449500). This work was also supported by Grant 2G12MD007592 from the National Institutes on Minority Health and Health Disparities (NIMHD), a component of the National Institutes of Health (NIH). J. L. Gardea-Torresdey acknowledges the Dudley family for the Endowed Research Professorship, the Academy of Applied Science/US Army Research Office, Research and Engineering Apprenticeship program (REAP) at UTEP, grant #W11NF-10-2-0076, sub-grant 13-7, and the LEER and STARs programs of the University of Texas System.
References
- J. R. Peralta-Videa, L. Zhao, M. L. Lopez-Moreno, G. de la Rosa, J. Hong and J. L. Gardea-Torresdey, J. Hazard. Mater., 2011, 186, 1 CrossRef CAS PubMed.
- Z. Chen, H. Meng, G. Xing, C. Chen, Y. Zhao, G. Jia, T. Wang, H. Yuan, C. Ye, F. Zhao, Z. Chai, C. Zhu, X. Fang, B. Ma and L. Wan, Toxicol. Lett., 2006, 163, 109 CrossRef CAS PubMed.
- Z. Wang, X. Xie, J. Zhao, X. Liu, W. Feng, J. C. White and B. Xing, Environ. Sci. Technol., 2012, 46, 4434 CrossRef CAS PubMed.
- O. Bondarenko, K. Juganson, A. Ivask, K. Kasemets, M. Mortimer and A. Kahru, Arch. Toxicol., 2013, 87, 1181 CrossRef CAS PubMed.
- N. Zuverza-Mena, I. A. Medina-Velo, A. C. Barrios, W. Tan, J. R. Peralta-Videa and J. L. Gardea-Torresdey, Environ. Sci.: Processes Impacts, 2015, 17, 1783 CAS.
- A. A. Keller, S. McFerran, A. Lazareva and S. Suh, J. Nanopart. Res., 2013, 15, 1 CrossRef.
-
H. Cheng, K. Klasson, T. Asakura and Q. Wu, in Nanotechnology: Delivering on the Promise Volume 2, ed. anonymous, ACS Publications, 2016, p. 233 Search PubMed.
- N. Zuverza-Mena, D. Martínez-Fernández, W. Du, J. A. Hernandez-Viezcas, N. Bonilla-Bird, M. L. López-Moreno, M. Komárek, J. R. Peralta-Videa and J. L. Gardea-Torresdey, Plant Physiol. Biochem., 2017, 110, 236 CrossRef CAS PubMed.
- S. Wu, L. Huang, J. Head, D. Chen, I. Kong and Y. Tang, J. Pet. Environ. Biotechnol., 2012, 3, 126 CAS.
- J. Trujillo-Reyes, S. Majumdar, C. Botez, J. Peralta-Videa and J. Gardea-Torresdey, J. Hazard. Mater., 2014, 267, 255 CrossRef CAS PubMed.
- J. Shi, A. D. Abid, I. M. Kennedy, K. R. Hristova and W. K. Silk, Environ. Pollut., 2011, 159, 1277 CrossRef CAS PubMed.
- C. M. Lalau, R. de Almeida Mohedano, É. C. Schmidt, Z. L. Bouzon, L. C. Ouriques, R. W. dos Santos, C. H. da Costa, D. S. Vicentini and W. G. Matias, Protoplasma, 2015, 252, 221 CrossRef CAS PubMed.
- D. H. Atha, H. Wang, E. J. Petersen, D. Cleveland, R. D. Holbrook, P. Jaruga, M. Dizdaroglu, B. Xing and B. C. Nelson, Environ. Sci. Technol., 2012, 46, 1819 CrossRef CAS PubMed.
- C. O. Dimkpa, J. E. McLean, D. E. Latta, E. Manangón, D. W. Britt, W. P. Johnson, M. I. Boyanov and A. J. Anderson, J. Nanopart. Res., 2012, 14, 1 CrossRef.
- S. Lee, H. Chung, S. Kim and I. Lee, Water, Air, Soil Pollut., 2013, 224, 1 CAS.
- P. M. G. Nair and I. M. Chung, Biol. Trace Elem. Res., 2014, 162, 342 CrossRef CAS PubMed.
- P. M. G. Nair, S. Kim and I. M. Chung, Acta Physiol. Plant., 2014, 36, 2947 CrossRef.
- J. Hong, C. M. Rico, L. Zhao, A. S. Adeleye, A. A. Keller, J. R. Peralta-Videa and J. L. Gardea-Torresdey, Environ. Sci.: Processes Impacts, 2015, 17, 177 CAS.
- T. R. Abu-Zahra, Middle East J. Sci. Res., 2012, 11, 1220 Search PubMed.
-
https://www.ers.usda.gov/webdocs/publications/39535/41021_vgs353sa1.pdf?v=42125, (accessed September 2017).
-
http://www.factfish.com/statistic-country/world/chillies%20and%20peppers%2C%20green%2C%20production%20quantity, (accessed June 2017).
- M. Ryżak and A. Bieganowski, J. Plant Nutr. Soil Sci., 2011, 174, 624 CrossRef.
-
https://pubs.usgs.gov/pp/1270/pdf/PP1270_508.pdf (accessed September 2017).
-
https://www.atsdr.cdc.gov/toxprofiles/tp.asp?id=206&tid=37 (accessed September 2017).
- S. Bandyopadhyay, G. Plascencia-Villa, A. Mukherjee, C. M. Rico, M. José-Yacamán, J. R. Peralta-Videa and J. L. Gardea-Torresdey, Sci. Total Environ., 2015, 515, 60 CrossRef PubMed.
- A. Mukherjee, Y. Sun, E. Morelius, C. Tamez, S. Bandyopadhyay, G. Niu, J. C. White, J. R. Peralta-Videa and J. L. Gardea-Torresdey, Front. Plant Sci., 2015, 6, 1242 Search PubMed.
- L. Zhao, Y. Sun, J. A. Hernandez-Viezcas, A. D. Servin, J. Hong, G. Niu, J. R. Peralta-Videa, M. Duarte-Gardea and J. L. Gardea-Torresdey, J. Agric. Food Chem., 2013, 61, 11945 CrossRef CAS PubMed.
- C. Gunawan, W. Y. Teoh, C. P. Marquis and R. Amal, ACS Nano, 2011, 5, 7214 CrossRef CAS PubMed.
- H. V. McShane, G. I. Sunahara, J. K. Whalen and W. H. Hendershot, Environ. Sci. Technol., 2014, 48, 8135 CrossRef CAS PubMed.
-
R. Bawa, G. F. Audette and I. Rubinstein, Handbook of Clinical Nanomedicine: Nanoparticles, Imaging, Therapy, and Clinical Applications, CRC Press, 2016, p. 154 Search PubMed.
- F. M. Eaton, J. Agric. Res., 1942, 64, 357 CAS.
- A. A. Keller, A. S. Adeleye, J. R. Conway, K. L. Garner, L. Zhao, G. N. Cherr, J. Hong, J. L. Gardea-Torresdey, H. A. Godwin, S. Hanna, Z. Ji, C. Kaweeteerawat, S. Lin, H. S. Lenihan, R. J. Miller, A. E. Nel, J. R. Peralta-Videa, S. L. Walker, A. A. Taylor, C. Torres-Duarte, J. I. Zink and N. Zuverza-Mena, NanoImpact, 2017, 7, 28 CrossRef.
- D. Julich and S. Gäth, Geoderma, 2014, 235–236, 127 CrossRef CAS.
- R. Sekine, E. R. Marzouk, M. Khaksar, K. G. Scheckel, J. P. Stegemeier, G. V. Lowry, E. Donner and E. Lombi, J. Environ. Qual., 2017 DOI:10.2134/jeq2016.12.0485.
- R. O'Dell, W. Silk, P. Green and V. Claassen, Environ. Pollut., 2007, 148 Search PubMed , 1, 115.
- D. Lin and B. Xing, Environ. Pollut., 2007, 150, 243 CrossRef CAS PubMed.
- W. Lee, Y. An, H. Yoon and H. Kweon, Environ. Toxicol. Chem., 2008, 27, 1915 CrossRef CAS PubMed.
- R. C. Monica and R. Cremonini, Caryologia, 2009, 62, 161 CrossRef.
- J. Fernandes and F. Henriques, Bot. Rev., 1991, 57, 246 CrossRef.
- G. V. Lowry, K. B. Gregory, S. C. Apte and J. R. Lead, Environ. Sci. Technol., 2012, 46, 6893 CrossRef CAS PubMed.
- D. Lin, X. Tian, F. Wu and B. Xing, J. Environ. Qual., 2010, 39, 1896 CrossRef PubMed.
- T. Karlsson, P. Persson and U. Skyllberg, Environ. Sci. Technol., 2006, 40, 2623 CrossRef CAS PubMed.
- M. Baalousha, A. Manciulea, S. Cumberland, K. Kendall and J. R. Lead, Environ. Toxicol. Chem., 2008, 27, 1875 CrossRef CAS PubMed.
- E. M. Hotze, T. Phenrat and G. V. Lowry, J. Environ. Qual., 2010, 39, 1909 CrossRef CAS PubMed.
- Y. T. He, J. Wan and T. Tokunaga, J. Nanopart. Res., 2008, 10, 321 CrossRef CAS.
- J. Nemecek, E. Podlesáková and R. Vácha, Rostl. Vyroba, 2002, 48(2), 45 CAS.
- G. S. Bañuelos and H. A. Ajwa, J. Environ. Sci. Health, Part A: Toxic/Hazard. Subst. Environ. Eng., 1999, 34, 951 CrossRef.
- Dietary Reference Intakes for Vitamin A, Vitamin K, Arsenic, Boron, Chromium, Copper, Iodine, Iron, Manganese, Molybdenum, Nickel, Silicon, Vanadium, and Zinc https://www.nap.edu/read/10026/chapter/1 (accessed September 2017).
- C. M. Rico, S. Majumdar, M. Duarte-Gardea, J. Peralta-Videa and J. Gardea-Torresdey, J. Agric. Food Chem., 2011, 59, 3485 CrossRef CAS PubMed.
- N. A. Ali, M. P. Bernal and M. Ater, Plant Soil, 2002, 239, 103 CrossRef CAS.
- N. M. Safaya, Soil Sci. Soc. Am. J., 1976, 40, 719 CrossRef CAS.
- H. Takahashi, A. Watanabe-Takahashi, F. W. Smith, M. Blake-Kalff, M. J. Hawkesford and K. Saito, Plant J., 2000, 23, 171 CrossRef CAS PubMed.
- T. Gigolashvili and S. Kopriva, Front. Plant Sci., 2014, 5, 442 Search PubMed.
-
C. S. Cobbett, in Sulphur in Plants, ed. Y. P. Abrol and A. Ahmad, Springer Netherlands, Dordrecht, 2003, p. 177 Search PubMed.
- C. Flemming and J. Trevors, Water, Air, Soil Pollut., 1989, 44, 143 CrossRef CAS.
-
E. A. Pilon-Smits, in Progress in botany, ed. anonymous, Springer, 2015, p. 93 Search PubMed.
- T. Sors, D. Ellis and D. Salt, Photosynth. Res., 2005, 86, 373 CrossRef CAS PubMed.
- Z. Nie, C. Hu, H. Liu, Q. Tan and X. Sun, Plant Physiol. Biochem., 2014, 82, 27 CrossRef CAS PubMed.
- H. Heuwinkel, E. Kirkby, J. L. Bot and H. Marschner, J. Plant Nutr., 1992, 15, 549 CrossRef CAS.
-
U. C. Gupta, Molybdenum in agriculture, Cambridge University Press, 1997 Search PubMed.
- J. Kudla, O. Batistic and K. Hashimoto, Plant Cell, 2010, 22, 541 CrossRef CAS PubMed.
- L. E. Williams, J. K. Pittman and J. Hall, Biochim. Biophys. Acta, 2000, 1465, 104 CrossRef CAS.
- A. H. Österås and M. Greger, Biol. Plant., 2006, 50, 647 CrossRef.
- P. Genet Alaoui-Sossé, F. Vinit-Dunand, M. Toussaint, D. Epron and P. Badot, Plant Sci., 2004, 166(5), 1213 CrossRef.
- K. Juang, Y. Lee, H. Lai and B. Chen, Ecotoxicol. Environ. Saf., 2014, 104, 36 CrossRef CAS PubMed.
- W. Guo, H. Nazim, Z. Liang and D. Yang, Crop J., 2016, 4(2), 83 CrossRef.
- H. Panou-Filotheou, A. Bosabalidis and S. Karataglis, Ann. Bot., 2001, 88, 207 CrossRef CAS.
-
L. Taiz and E. Zeiger, Plant Physiology, Sinauer Associates, Sunderland, MA, 3rd edn, 2002 Search PubMed.
- L. Ochoa, I. A. Medina-Velo, A. C. Barrios, N. J. Bonilla-Bird, J. A. Hernandez-Viezcas, J. R. Peralta-Videa and J. L. Gardea-Torresdey, Sci. Total Environ., 2017, 598, 513 CrossRef CAS PubMed.
-
http://extension.missouri.edu/p/MG4 (accessed September 2017).
-
R. Bardgett, The biology of soil: a community and ecosystem approach, Oxford University Press, 2005 Search PubMed.
- M. Gupta and S. Gupta, Front. Plant Sci., 2016, 7, 2074 Search PubMed.
- M. Shahbaz, S. Parmar, C. E. E. Stuivar, M. J. Hawkesford and L. J. D. Kok, Environ. Exp. Bot., 2013, 88, 60 CrossRef CAS.
- D. A. Cataldo, T. R. Garland and R. E. Wildung, Plant Physiol., 1978, 62, 563 CrossRef CAS PubMed.
-
A. Kabata-Pendias and H. Pendias, Trace elements in soils and plants, CRC press Boca Raton, 1984 Search PubMed.
- O. Lastra, A. Chueca, C. Gonzalez, M. Lachica and J. L. Gorge, An. Edafol. Agrobiol., 1987, 46, 1005 CAS.
- P. H. T. Beckett and R. D. Davis, New Phytol., 1978, 81, 155 CrossRef CAS.
- M. T. C. Cayton, E. D. Reyes and H. U. Neue, Plant Soil, 1985, 87, 319 CrossRef CAS.
- F. M. Chaudhry and S. F. Loneragan, Soil Sci. Soc. Am. Proc., 1972, 36, 323 CrossRef CAS.
- A. Rashid, F. M. Chaudhry and M. Sharif, Plant Soil, 1976, 45, 613 CrossRef CAS.
- S. Lin, A. A. Taylor, Z. Ji, C. H. Chang, N. M. Kinsinger, W. Ueng, S. L. Walker and A. E. Nel, ACS Nano, 2015, 9, 2038 CrossRef CAS PubMed.
Footnote |
† Electronic supplementary information (ESI) available. See DOI: 10.1039/c7en00697g |
|
This journal is © The Royal Society of Chemistry 2018 |
Click here to see how this site uses Cookies. View our privacy policy here.