DOI:
10.1039/C5RA17715D
(Review Article)
RSC Adv., 2015,
5, 93002-93026
New advances in non-fullerene acceptor based organic solar cells
Received
1st September 2015
, Accepted 1st October 2015
First published on 8th October 2015
Abstract
Non-fullerene organic solar cells (NF-OSCs), in which an n-type organic molecule instead of a fullerene derivative is utilized as the electron-acceptor material, have recently emerged as a new topic in the field of organic solar cells. Replacement of the traditional fullerene acceptor in the photoactive layer of a normal organic solar cell with the organic acceptor gives rise to several advantages, like light absorption and energy level tunability, diversity of donor-to-acceptor combination, and large-scale production of acceptor materials. Studies on NF-OSCs can be traced back to 1986, when the first bilayered organic solar cell was proposed. Unfortunately, they has been advancing very slowly and the power-conversion-efficiency (PCE) was only approaching or exceeding 2% up to 2012. Fast advances have been driven forward since 2013, when the PCE value first broke through 4%, and the reported PCE value has now reached about 8% after a short period of 3 years. If we turn to natural systems such as the photosynthesis systems I and II, in which Nature utilizes organic molecules to accomplish high-efficiency solar-to-chemical energy conversion through the cascade unidirectional electron–hole transfer paths, we can rationally expect an even higher PCE and a convincing future for NF-OSCs. In this review, we will address recent new progress in this sub-branch of organic solar cells.
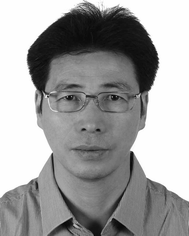 Chuanlang Zhan | Chuanlang Zhan is a professor of chemistry at the Institute of Chemistry, Chinese Academy of Sciences (CAS). His research activities focus on synthesis and self-assembly of functional molecular materials, solar energy conversion and solar cells. He received a PhD degree (2000) in Organic Chemistry from the Institute of Photographic Chemistry, CAS, under the supervision of Prof. Duoyuan Wang, and joined the Institute of Chemistry, CAS, as a postdoctoral research fellow at CAS Key Laboratory of Organic Solids, Institute of Chemistry, CAS, and later was promoted to Associate Professor at this institute. |
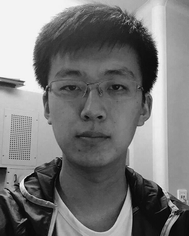 Xinliang Zhang | Xinliang Zhang received his BS degree from College of Chemistry and Molecular Engineering at Peking University in 2011. He is now a PhD student at the Institute of Chemistry, Chinese Academy of Sciences. His research interests include the synthesis and device engineering of organic solar cells. |
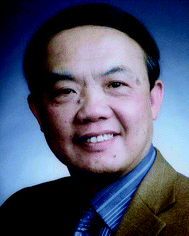 Jiannian Yao | Jiannian Yao received his PhD degree with Prof. Akira Fujishima at Tokyo University in 1993. Now he serves as a Professor of Chemistry at ICCAS, the president of the Chinese Chemical Society, and the vice president of the National Natural Science Foundation of China. His research interests are organic and inorganic optofunctional nanomaterials. |
1. Introduction
Energy consumption supports the economic development of the world, and currently fossil energy sources such as natural gas, petroleum and coal are the major sources. For example, fossil energy sources were used for 82.1% of total energy consumption in the U.S. in 2013.1 As we know, the reserves of fossil energy sources are limited and burning leads to the emission of CO2 and other pollutant chemicals. Therefore, the development of clean and renewable energy sources has become one of the major scientific and technological topics of the 21st century and clean, green and sustainable solar energy is one of these sources. Currently, inorganic photovoltaic technology, such as silicon or cadmium telluride based technology, holds the dominant position in industrial production, owing to its relatively mature technology and high power conversion efficiency (PCE) of over 20%.2 Alternatively, organic photovoltaic cells utilize synthesis-accessible organic molecules as the light-capturing materials. They have several distinct advantages such as low-cost, mechanical flexibility, light weight, color-tunability, and semi-transparency. Particularly, the optoelectronic properties of organic materials can be easily tuned via organic synthesis to improve their solar photon capturing capability. The development of the bulk heterojunction (BHJ) structure3 spans the gap between the short exciton diffusion length (typically, 5–20 nm) of organic semiconductors and the film thickness (typically 100 nm) required for efficiently capturing the solar photons. The solution-process technology endows bulk heterojunction organic solar cells (BHJ OSCs) with high potential for the mass production of flexible and cost-effective cells.
Since the first bilayered organic solar cell was proposed in 1986,4 organic solar cells have been studied for over 30 years. Their efficiency has been increased from the initial 1% to the current 10–12% through donor-material and cell-structure innovations.5,6 Recently, a new research topic has emerged in the field of organic solar cells: non-fullerene organic solar cells (NF-OSCs). Traditional OSCs utilize fullerene acceptors, typically [6,6]-phenyl-C61 (or C71)-butyric acid methyl ester (PC61BM or PC71BM) as the electron-acceptor materials, while n-type organic molecules are selected to replace fullerenes as the acceptor materials in NF-OSCs. In the past 3 years, NF-OSCs have been paid increasing attention and fast advances have been achieved. Several review papers were published addressing small-molecule and polymeric organic acceptors before the end of 2013.7–13 Several review papers have been recently published to address this research topic, typically from the molecular point of view.14–16 In addition, this topic has been included in several recent review papers.17–19 On the basis of the fast success in this newly emerging topic, we herein focus mainly on recent advances in the molecular design of organic acceptors, and, particularly, on the realization of efficient non-fullerene solar cells by addressing the donor-to-acceptor combinations, from the following three aspects: spectral coverage, energy level matching and film morphology.
In this review article, we first introduce “Concept, classification and recombination mechanisms” (part 2), which includes the subparts of “What are NF-OSCs”, “Why are NF-acceptors under consideration”, “Classification of NF-OSCs”, and “Recombination loss in a cell device” which is a key issue in developing an efficient NF-OSC as well as “Design principles for efficient organic acceptors”. Then, we will go into the recent advances in both small-molecule (part 3) and polymer (part 4) acceptors and the challenges in designing high-efficiency NF-OSCs (part 5). The final part is about the conclusions and outlook (part 6).
2. Concept, classification and recombination mechanisms
2.1. What are NF-OSCs?
For a typical organic solar cell, the photoactive organic layer is sandwiched by a transparent and back electrode, respectively, forming a single-junction cell device. A single-junction cell device can be fabricated in either a conventional or an inverted configuration, depending on the flow direction of the mobile charge carriers (Fig. 1A). The free holes are collected by the transparent electrode and the mobile electrons are gathered by the back electrode in the convention-structured solar cell, while the holes and electrons are collected in an opposite manner in the inverted solar cell. Modification of the electrode surface by using an interlayer with an appropriate work function is necessary to form an Ohmic contact and energy level alignment between the inorganic electrode and the photoactive organic layer, allowing selective collection of the mobile charge carriers on the right electrode.20,21 For example, the work function of PEDOT:PSS (poly(3,4-ethylenedioxythiophene):poly(styrenesulfonate)) is about 5.0 eV. Modification of the ITO surface with PEDOT:PSS can selectively allow the efficient transport of the mobile holes, down to the ITO electrode in a normal cell structure, while MoOx is a commonly used hole transporting layer in an inverted configuration (Fig. 1B).
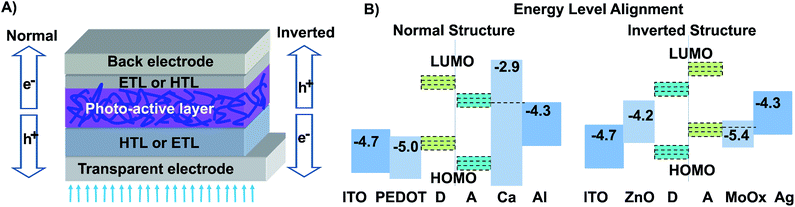 |
| Fig. 1 (A) Depiction of a typical conventional and inverted device structure, in which a hole transporting layer (HTL) and an electron transporting layer (ETL) are selectively utilized to modify the transparent and back electrode, respectively, to allow the selective collection of the mobile holes and electrons. (B) Schematic models of energy level alignments in the conventional and inverted cell. | |
In BHJ OSCs, the photoactive layer comprises electron-donor (D) and electron-acceptor (A) materials, blended in a BHJ structure. The donor materials can be the polymer or small molecule and the acceptors can be the fullerene derivatives, i.e. PC61BM or PC71BM or n-type organic molecules. On the basis of the kind of acceptor material used in an OSC, either fullerene or organic, the resulting cell is called a fullerene or non-fullerene organic solar cell. For fullerene organic solar cells, the well-known polymer organic solar cells (PSCs) and small-molecule organic solar cells (SM-OSCs) are fabricated using polymers and small-molecules as the donor materials, respectively.
Thanks to community scientists’ great efforts in the molecular design and synthesis of donor materials, either small-molecules or polymers, processing techniques for the optimization of organic blend film morphology, judicious interfacial engineering, and cell device structure, the PCE has been recently boosted to 10–11% for a single-junction state-of-the-art fullerene solar cell with small-molecules24,25 or polymers5,26–35 as the donor materials, and up to 11–12% for a tandem cell.6,36,37 Fig. 2 presents typical polymer and small-molecule donors from which over 10% PCE has been realized.
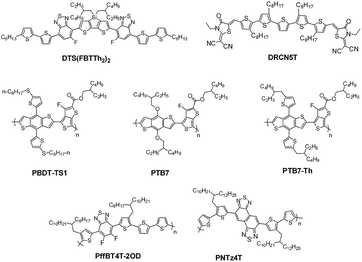 |
| Fig. 2 The molecular structures of typical small-molecule and polymer donors from which a PCE of 10–11% or 11–12% has been realized for single-junction or tandem solar cells. The molecules are reported as follows: PTB7 (Eoptg = 1.63 eV),22 PTB7-Th (1.58 eV),23 DTS(FBTTh2)2 (1.55 eV),24 DRCN5T (1.60 eV),25 PBDT-TS1 (1.51 eV),26 PffBT4T-2OD (1.55 eV),5 and PNTz4T (1.54 eV).34 | |
With respect to the fullerene counterpart, the NF-OSC is slow over a long period of time. The reported PCE value went below or above 1% before 2010 (Fig. 3). The PCE value first broke through 2% at the end of 2010 by using a blend of a 2-dimensional (2D) conjugated polymer donor38 and a perylene diimide (PDI) based polymer acceptor (with a PCE of 2.23%).39 A year later, another value of 2.54% was published for a naphthalimide-vinyl-benzothiadiazole based small molecule acceptor blended with poly(3-hexylthiophene) (P3HT).40 In 2013, the PCE value further broke through 4% by using a thienyl-bridged PDI dimer acceptor, so-called bis-PDI-T-EG, which was blended with the conjugated polymer of PBDTTT-C-T.41 In this year, there were reported two kinds of PDI dimer acceptors, in which two PDI chromophores were covalently linked through their imide positions via a single bond42 or through their bay positions via an aromatic unit.43–45 The former gave a PCE of 3.2% when blended with PBDTTT-C-T.42 The latter yielded a PCE of 0.3–2.5% when blended with P3HT, depending on the structure of the bridged aromatic unit43,44 and the number of the substituent groups on the bay region of the PDI dimer.45 Very recently, a PCE of close to 8% has been reported.46 From Fig. 3, one can see that NF-OSCs have been advancing faster and faster since 2013 thanks to the great efforts of the community in acceptor material design, selection of the donor-to-acceptor system, optimization of the film morphology, as well as interlayer engineering between the organic photoactive layer and the electrode, as addressed in parts 3 and 4 of this paper. In short, NF-OSCs have emerged as a new, growing and interesting sub-branch in the field of organic solar cells in recent years.
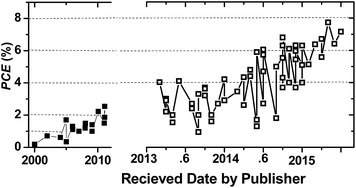 |
| Fig. 3 A representative depiction showing the key historical PCE values achieved from solution-processed BHJ NF-OSCs versus the dates of the papers received by the publishers. | |
2.2. Why are organic acceptors under consideration?
PCBM (including both PC61BM and PC71BM hereafter) is an excellent acceptor material, in particular, for utilization in organic solar cells. PCBM can not only finely intermix with donors to quench excitons but also forms nanoscale aggregate domains which, combined with its high electron affinity, benefits the acceptance of electrons from the donor semiconductor. Its spherical conjugated structure can not only greatly increase the chance of forming a beneficial alignment with the donor π-system in all directions for charge separation, but it also shows the capability of forming carrier-transport favourable nanoscale interpenetrating networks with the donor, which is helpful for charge transportation from the active layer to the electrode. Nevertheless, PCBM has several small drawbacks.47 For example:
(1) There are no hydrogen atoms covalently linked to the fullerene backbone and an adduction reaction is the most frequently used way to chemically modify the fullerene backbone. Following this reaction, the energy level of the lowest unoccupied molecular orbital (LUMO) is raised by breaking down the conjugation of the π-electrons,48 while it is difficult to establish conjugation between the π-electrons of the fullerene and those of the covalently attached functions, which limits the fine-tuning of its optoelectronic properties, shifting, for example, the absorption to the long wavelength range and further enhancing the absorptivity in the visible and near infrared (IR) region.
(2) The adduction reaction on the fullerene backbone is weakly selective and this not only lowers the yield of the target product but also magnifies the purification difficulty. Given that the impurity may be severely detrimental to the power conversion efficiency of PCBMs, specialist techniques, like high performance liquid chromatography (HPLC), are needed to produce high quality PCBMs,49 which then elevates the PCBMs’ price and limits the practical applications.
(3) As Fig. 4 displays, PC61BM has a very weak absorption beyond 425 nm in the solution state or beyond 545 nm in a thin-film, while PC71BM shows relatively stronger absorption in the visible region, typically before 750 nm. Therefore, the donor material in the fullerene solar cell plays an important role in absorbing the solar photons distributed in the visible and near infrared wavelength region. Thanks to the efforts of materials scientists, numerous donor materials have been successfully synthesized to harvest the solar photons at wavelengths longer than 500 nm. The absorption band position and shape, the bandgap or the absorption onset, and the LUMO/HOMO energy levels can be successfully fine-tuned with several synthetic strategies, such as the well-known and facile donor–acceptor (D–A) conjugation strategy and chemical modifications with polar heteroatoms, for example, for the synthesis of the high-efficiency small-molecule and polymer donor materials shown in Fig. 2. However, it is as yet a big challenge to synthesize a donor material that can absorb across a wide wavelength range, spanning, for example, 400 to 800 nm, and even up to 1000 nm, to capture solar photons as much as possible.
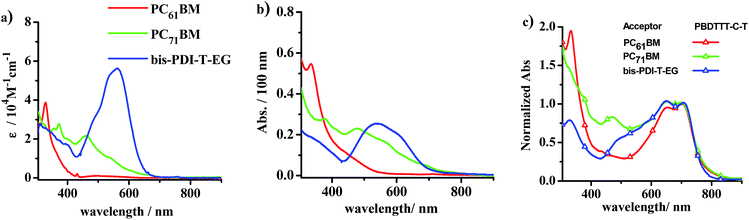 |
| Fig. 4 The absorption spectra of PC61BM (red), PC71BM (green) and bis-PDI-T-EG (blue) in (a) chloroform (obtained with a concentration of 1 × 10−5 M) and (b) pristine films (spun-cast from the corresponding chloroform solution). (c) The absorption spectra of PBDTTT-C-T:PC61BM (red + triangles), PBDTTT-C-T:PC71BM (green + triangles), PBDTTT-C-T:bis-PDI-T-EG (blue + triangles) blended films (1 : 1, wt/wt). The PBDTTT-CT:PC61BM and PBDTTT-CT:PC71BM blended films were spun-cast from the corresponding o-dichlorobenzene (o-DCB) solution with 1,8-diiodooctane (DIO, 3% v/v). The PBDTTT-CT:bis-PDI-T-EG blended films were spun-cast from o-dichlorobenzene (o-DCB) solution with DIO (2% v/v) and treated by solvent vapour annealing as shown in the reported literature.50 Each spectrum was obtained by averaging the data of five parallel experiments. The spectra in (c) are normalized to see the contribution of the acceptor materials to the absorption of the blend film. | |
In striking contrast to fullerene acceptors, the chemical structure of non-fullerene organic acceptors can be more easily modified and their source materials are much more readily available. Their spectral coverage can be more easily tuned through chemical modification of the π-system. Therefore, the full coverage of the solar spectrum can become more easily attainable by judicious design of the acceptor molecules and then selection of an organic-donor-to-organic-acceptor combination, by combining, for example, a narrow bandgap donor material (Eg < 1.6 eV) with a medium bandgap acceptor material (1.6 < Eg < 2.2 eV), whose spectral coverage is complementary to that of the donor. For example, the PBDTTT-C-T:bis-PDI-T-EG system41 whose absorption spectra are shown in Fig. 4. Meanwhile, tunability of the LUMO and HOMO energy levels of the organic acceptor can allow us to not only match the LUMO energy but also the HOMO energy between the organic donor and non-fullerene acceptor, which guarantees efficient charge separation for the excitons generated by the donor and acceptor phases, which dissociate through the so-called electron and hole transfer paths, respectively.50
As shown in Fig. 4a, the absorptivity of the PC71BM solution is above the order of 1 × 104 M−1 cm−1 in the visible region, typically from 400 to 550 nm. This absorption coefficient is comparable to most organic chromophores such as the well-known PDI.19 Upon casting into a thin film, the absorbance of the PC71BM neat film is only slightly weaker, as the wavelength is beyond 500 nm, than that of a typical PDI dimer, bis-PDI-T-EG. From the blend films with PBDTTT-C-T as the donor material, for example, one can see that the PBDTTT-C-T film blended with bis-PDI-T-EG even has a relatively weaker absorption than the PC71BM blended film in the wavelength range before 500 nm, although a comparable absorption can be seen when the wavelength of the incident light is beyond 500 nm. Therefore, only the PC61BM blend film may have weaker absorption than organic acceptor blend, while the PC71BM blend normally absorbs the visible and near infrared solar photons in strength comparable to the organic acceptor blend with the same donor material.
Why are we interested in replacing the currently dominant and efficient fullerene acceptor with the n-type organic molecule? The reasons are related to, but not limited to, the above-mentioned drawbacks of PCBM over organic acceptors. Indeed, if we look to Nature, we can see that the natural photosynthetic systems I and II do utilize organic molecules to accomplish high-efficiency solar to chemical energy conversion, in which the photoinduced excitons can be efficiently separated and exploited with an internal quantum efficiency of nearly 100% through the cascade unidirectional electron–hole transfer paths. Accordingly, we reasonably believe that replacement of the fullerene acceptors with organic molecules should make sense. The big issue is which organic molecule can achieve high efficiency and how to realize it.
2.3. Classification of organic acceptors and NF-OSCs
Like donor materials, of which there are polymer and small-molecule types, organic acceptor materials can also be classified into small-molecule and polymer types. There are therefore four possible donor-to-acceptor combinations, from polymer donor and acceptor as well as small-molecule donor and acceptor, giving rise to four kinds of NF-OSCs, as Fig. 5 shows. Small-molecule acceptors have identical molecular structures and molecular weights, and high purity as well as batch-to-batch reproducibility. They are commonly synthesized from photoactive π-aromatics by stepwise coupling procedures. In contrast, polymer acceptors are normally synthesized by polymerization from photoactive π-aromatics and there is generally a distribution of molecular weight, as deduced from the weight-average molecular weight (Mw), number-average molecular weight (Mn), and polydispersity index (PDI). Studies have pointed out that different molecular weight distributions should lead to different cell performances.51 Therefore, the batch-to-batch difference in the cell performance of polymer-based solar cells is normally significant.
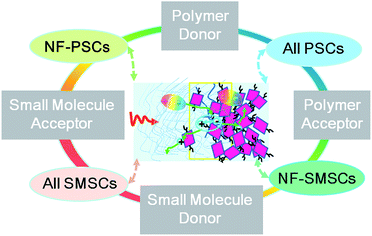 |
| Fig. 5 Four possible organic-donor-to-organic-acceptor combinations arising for non-fullerene solar cells: the combination of polymer donor to polymer acceptor gives rise to the so-called all polymer organic solar cells (all PSCs);52 the combination of small-molecule donor to small-molecule acceptor gives rise to the so-called all small-molecule organic solar cells (all SMSCs).47,53 Herein, we named the polymer donor to small-molecule acceptor and the small-molecule donor to polymer acceptor systems non-fullerene polymer organic solar cells (NF-PSCs) and non-fullerene small-molecule organic solar cells (NF-SMSCs), simply following the classifications of the fullerene based organic solar cells; i.e. polymer organic solar cells (PSCs) and small-molecule organic solar cells (SMSCs), respectively. | |
2.4. Recombination loss in OSCs
The PCE (%) of a solar cell device is defined by the equation PCE = JscVocFF/Pin, wherein Jsc is the short-circuit current-density in mA cm−2, Voc is the open-circuit voltage in V, FF is the fill-factor in %, and Pin is the incident light intensity in mW cm−2. Under the standard illumination conditions, the incident light intensity is 100 mW cm−2, which can be provided by an AAA-grade simulated AM 1.5G light source. The cell parameters of Jsc, Voc, and FF are derived from the experimental current-density–voltage (J–V) curve (Fig. 6). The FF is the graphic measurement from the J–V curve and is defined as FF = (VmppJmpp)/(VocJsc), where Vmpp and Jmpp are the J and V values at the maximum power point (mpp).
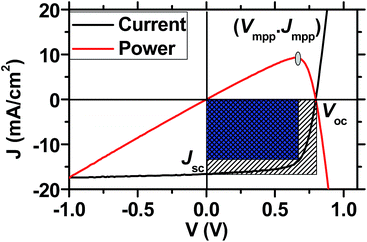 |
| Fig. 6 Typical current-density–voltage (J–V) characteristics (black) and the relative output electric power–voltage curve (red) from a BHJ solar cell under illumination by an AAA-grade AM1.5G simulator. | |
Today, the efficiency of NF-OSCs lags behind their fullerene counterparts. Because the LUMO energy level of organic acceptors can be tuned towards a higher-lying level than that of PCBMs, the Voc is normally higher than PCBM based solar cells when blended with the same donor material and using an identical cell structure. The lower efficiency is usually derived from the lower Jsc and FF, both of which are deeply related to the recombination loss and carrier mobility and lifetime. We herein paid attention to the recombination loss in a solar cell device.
The experimental current (J), as shown in the J–V characteristic curve, can be expressed by the formula J = neμE, where n is the photogenerated carrier concentration, e is the electric charge, μ is the carrier mobility and E is the electric field. The mobile carrier’s concentration and mobility are two experimental factors that we can manipulate through material design and film morphology control.
The mobile carriers are initially generated at the donor-to-acceptor interfaces and transport, respectively, through the interpenetrating acceptor and donor phases. Then they are collected by the right electrode. The ultimate concentration of the mobile carriers by the right electrode, n, is defined by exciton generation and recombination losses, and can be written as n = n0 − an0, where n0 is the total concentration of the excitons generated by both the blended donor and acceptor phases, a is a dimensionless parameter, as defined by a = (n0 − n)/n0, representing the recombination loss during the exciton diffusion and separation as well as mobile carrier drift and collection. Normally a ≤ 1. This is because recombination loss always exists in organic semiconductor films.
The recombination mechanisms in a BHJ solar cell device involve both the so-called geminate and non-geminate recombination losses. In the photoactive layer of a BHJ OSC device, the blended donor and acceptor materials can both absorb solar photons and generate excitons, the singlet excitons (Fig. 7A). The excitons’ concentration is dependent on the light-capturing ability of the photoactive layer. The excitons can be relaxed down to the ground state via both the radiative and nonradiative paths before they get to the donor-to-acceptor interface.
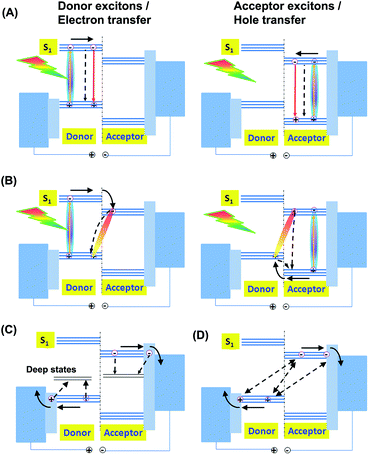 |
| Fig. 7 Schematic depictions of the generation of donor excitons and acceptor excitons, electron and hole transfer, and loss mechanisms via exciton decay (A), recombination of bound electron–hole pairs (B), and recombination of mobile electrons and holes as trapped by deep states (C) or in a bimolecular mechanism (D). | |
After accessing the donor-to-acceptor interface, the excitons are separated via the electron and hole transfer paths (Fig. 7B), respectively, forming mobile carriers, i.e. free electrons and holes. Current studies demonstrate that there exists an intermediate charge-transfer (CT) state associated with the exciton dissociation. For example, after the excited electron goes from the lowest unoccupied molecular orbital (LUMO) of the donor to that of the acceptor, the electron and hole are both bound together strongly by their mutual Coulombic force, forming a CT state.54 The bound electron–hole pairs are then spatially dissociated, driven by the internal field (Vint), forming free electrons and holes, i.e. free charge carriers.55 The Vint is defined by the equation Vint = Vbi − Vapp. Here Vbi is the build-in voltage defined as Jph is zero and Vapp is the applied bias. Other than the separation, the bound electron–hole pairs can be recombined to go back to the ground state (Fig. 7B).
The free electrons and holes, respectively, drift across the acceptor and donor phases, driven by the internal field, and are swept out down to the right electrode and then collected. Accompanying the carrier transportation and collection, the free carriers can be captured by traps (band tails) (Fig. 7C) or recombined between the mobile electron and hole pairs (Fig. 7D).
Taken together, the factor a is a product of the losses from exciton decay, recombination at the donor-to-acceptor interfaces and that from carrier transportation and collection. Nonradiative decay is an important way of inducing exciton loss before they move to the donor-to-acceptor interface (Fig. 7A). The effective exciton diffusion length is usually short, typically 5–20 nm, in organic semiconductor films and control of the phase size so that it is comparable to the exciton diffusion length is a prerequisite for realization of efficient BHJ OSCs. The exciton loss restricts the maximum generation rate of the obtainable bound electron–hole pairs (Ge–hmax, in m3 s−1)56,57 in a given cell device. The relationship between the Ge–hmax and the maximum generation rate of the excitons (Gexcitonmax, in m3 s−1) can be presented by an exciton loss factor of a1 according to Ge–hmax = (1 − a1)Gexcitonmax. The Ge–hmax is defined by the formula Ge–hmax = Jph,sat/eL,56,57 where L is the thickness of the active layer and Jph,sat is the reverse saturated photocurrent, Jph, which is related to the experimental light current JL and the dark diode current JD on the basis of Jph = JL − JD. In a state-of-the-art cell device, the phase size follows the length range of the effective exciton diffusion length and the exciton loss factor of a1 can be assumed to be close to one.
The recombination losses of the bound electron–hole pairs and the free electrons and holes include so-called monomolecular and bimolecular loss mechanisms. The monomolecular one refers to any first-order processes, involving the geminate losses of the bound electron–hole pairs, i.e. the CT states recombine before the bound electron–hole pairs separate (Fig. 7B), and the Shockley–Read–Hall (SRH) recombination induced by the deep states at the donor-to-acceptor interfaces, organic domain-to-domain interfaces and active layer-to-electrode interfaces and in the donor/acceptor domain phases (Fig. 7C). The geminate loss can be reflected by using femtosecond (fs) transient absorption pump-probe experiments.58 The bimolecular one is a second-order process, referring to the recombination of free electrons and holes (Fig. 7D). If we use a2, a3 and a4 to describe the loss following the geminate recombination of the CT states, SRH recombination and bimolecular mechanism of free electrons and holes, respectively, the total loss can be written as a = a1a2a3a4. However, it is as yet difficult to quantify these loss factors.
The recombination losses of the free electrons and holes can be qualitatively reflected by the incident light power dependent J–V characteristics, which are measured from a given cell device by varying the incident light power (P0). For example, from one sun down to 0.01 sun. At short-circuit where the Vint is large enough to efficiently sweep out the photogenerated free electrons and holes prior to recombination, the loss is dominated by the SRH recombination. This mechanism can be described using Jsc ∝ P0α.59 If all of the free electrons and holes can be collected under the short-circuit conditions, meaning the bimolecular loss is negligible, the Jsc should be linearly dependent on the incident light power with α = 1. When the fitting α value is smaller than one it suggests contribution from the bimolecular mechanism, and a smaller α value means that a more serious bimolecular loss is involved. At open-circuit the photogenerated free electrons and holes all recombine. The recombination mechanism can be reflected by the formula Voc ∝ nkT/q
ln(P),60 where k, T and q are the Boltzmann constant, the temperature in Kelvin and the elementary charge, respectively. Principally, if the bimolecular process dominates the recombination, the fitting n value should be one. As the SRH mechanism is involved, the recombination from shallow traps competes with the bimolecular and the fitting n value deviates from one: A larger n value indicates a more serious loss is involved for the SRH recombination.
In addition to manipulating the recombination loss by optimization of the film morphology and modifications of the electrode surface, enhancement of the light-capturing ability of the photoactive donor and acceptor materials can increase the maximum generation rate of the excitons. Hence, synthesizing donor and acceptor materials with a higher absorptivity, a wider solar spectrum coverage, and a lower bandgap is another goal, besides the reduction of the recombination loss, of materials scientists towards improving the current-density (Fig. 8A).
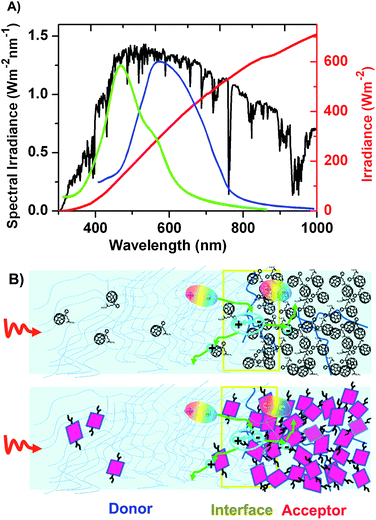 |
| Fig. 8 (A) The solar spectrum (black), integrated irradiance versus wavelength (red) and modelling absorption spectra (green and blue) of donor–acceptor materials. (B) Schematic depiction of phase-separated donor and acceptor rich phases and their interface. The acceptor molecules are shown by the spherical PCBM molecules (upper) or by planar rectangles representing organic molecules (lower), respectively. | |
The energy offset between the LUMOs of the donor and acceptor materials (ΔELUMO = ELUMO,donor − ELUMO,acceptor), and that between the highest occupied molecular orbitals (HOMOs) of the donor and acceptor materials (ΔEHOMO = EHOMO,donor − EHOMO,acceptor) are the driving forces for the singlet excitons to overcome their mutual Coulombic binding energy, driving the excited electron transfer from donor to acceptor and the hole transfer from acceptor to donor, respectively. It is normally accepted that the LUMO energy level of the donor should be at least 0.3 eV higher than that of the acceptor for efficiently driving the separation of the singlet donor excitons. The Voc is widely observed to correlate with the energy level difference between the donor HOMO and the acceptor LUMO, as defined by EDA = ELUMO,acceptor − EHOMO,donor. Upon photoexcitation, a CT state is formed at the donor-to-acceptor interface. A shift of the energy level of the CT state will lead to a change of Voc.61 Electronic coupling62 and composition63 between the donor and acceptor materials, and energy ordering64 are other factors that influence the Voc value. Since the energy level of the frontier molecular orbitals, i.e. the LUMO and HOMO, determine the material’s bandgap, judiciously engineering the energy levels of the frontier molecular orbitals and the absorption spectrum are both the most important issues, while it is as yet a big challenge to meet the criteria required for the synthesis of efficient donor–acceptor materials, i.e. simultaneously guaranteeing a narrow bandgap, an appropriate driving force for efficient exciton-separation, and a high Voc.
With respect to the understanding of Jsc and Voc, we have learned much less on FF as yet. The recombination loss significantly scales the FF value. As the bimolecular loss is reduced, the J–V curve will become steep near the open-circuit condition, and as the monomolecular loss becomes weak, the curve near the short-circuit condition will be flat. Generally, a higher FF value may be achieved through reduction of the recombination loss by optimizing the film morphology of the active layer, modification of the electrode-active layer interface, and an increase of the carrier mobility and lifetime. Nevertheless, achieving a high FF value is as yet a big challenge for NF-OSCs.
2.5. Design principles for efficient organic acceptors
Similar to the requirements for efficient donor materials, a proper efficient organic acceptor should meet the following prerequisites: solar photon capturing ability, electron affinity and mobility, and self-assembling and phase-separation ability related to the formation of nanoscale interpenetrating networks with the blended donor material.
The photoactive layer consists of the blended donor and acceptor materials. Absorptivity of an organic material determines how many solar photons at a given wavelength can be absorbed, and its absorption band shape and bandgap determines the wavelength region within which the solar photons can be captured. As we know, upon photoexcitation an organic molecule normally gives an absorption band in a certain wavelength region with an absorption peak and a high and low energy edge, respectively, as demonstrated by the well-known Jablonski diagram. Accordingly, one facile way to cover the solar spectrum as widely as possible is to complement the absorption spectra of the donor and acceptor. For example, the donor is arranged to absorb low energy solar photons, while the acceptor is arranged to absorb high energy photons. This requires that we must comprehensively consider the absorptivity, bandgap and energy levels of the frontier molecular orbitals to meet the requirements of light absorption and electron affinity when designing acceptor molecules.
Other than the absorption property and the energy levels, film morphology is another key factor, because efficient charge generation and transportation are both limited by the formation of an ideal film morphology, including the formation of nanoscale interpenetrating networks, which are beneficial for exciton-separation, and long-range backbone-to-backbone packing, which is helpful for mobile carrier transport to the right electrode. Organic acceptors comprise planar aromatic π-units. This character is distinctly different to the spherical shape of the fullerene π-system. It is to date a big challenge to achieve both nanoscale interpenetrating networks and long-range molecular ordering from an organic acceptor and an organic donor system. Besides this, the donor-to-acceptor interfacial structure (Fig. 8B) plays an important role in the charge separation,65–68 and hence, special considerations should be taken into account when designing organic acceptor molecules.
The fast development of modern physical and organic chemistry provides us the possibility to “design” the absorption band position and frontier molecular orbitals; for example, by carefully selecting and engineering the conjugation size of aromatic π-systems and electron-donating or accepting substituent groups on the π-systems. The rich means for carbon–carbon bond formation, as built by modern organic synthetic chemistry, for example the well-known and facile Stille and Suzuki coupling reactions, allows us to accomplish the synthesis of the designed organic molecules. With respect to knowledge of the optoelectronic properties of an organic molecule, the understanding of the molecular assembly is relatively poor and we cannot “predict” the aggregation behavior of an organic molecule and the underlying structure of the assembled aggregates. Moreover, we have not yet developed the powerful tools needed to “see” the intrinsic underlying structure of organic aggregates. Therefore, designing the film morphology is much more difficult than the prediction of the optoelectronic properties of an organic molecule system.
3. Small-molecule non-fullerene acceptors
3.1. PDI based small molecules
PDI is a traditional n-type semiconducting dye chromophore. Its absorption appears around 500 nm as a band in between 400 and 600 nm. Its absorptivity is in the order of 104 to 105 M−1 cm−1. Its LUMO energy is around −4 eV and its electron mobility can be over 1 cm2 V−1 s−1.69 Additionally, PDIs are stable to light, heat, and chemicals.19,70 These optoelectronic properties make PDI units a potential candidate for constructing organic acceptors. However, molecular self-assembly studies on PDI derivatives have clearly demonstrated that the large planar π-system of the PDI chromophore always leads to the formation of hundreds of crystalline aggregates.19,70–72 Accordingly, strong self-trapping of the excitons takes place within the large aggregates, which severely limits the exciton diffusion/separation efficiencies and further decreases the PCEs of the cell devices.73 So far, several chemical modification approaches have been approved as facile ways to reduce the aggregation tendency for PDI chromophores (vide post). These approaches normally proceed at the nitrogen position, on the bay region (1, 6, 7 and 12-positions), or even on the 2, 5, 8 and 11-positions (headland positions) (Fig. 9A), including imidization using soluble side chains and/or aromatic moieties, or even the formation of twisted dimeric, trimeric and tetrameric conjugation backbones.
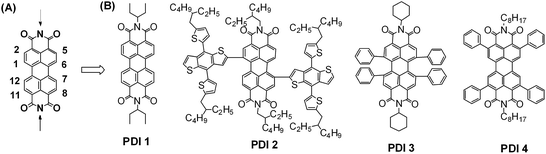 |
| Fig. 9 (A) Available chemical modification positions on the PDI core and (B) chemical structures of typical PDI monomer based small-molecule acceptors. | |
PDI monomer. The oldest strategy to synthesize soluble PDI derivatives is to introduce soluble alkyl chains on the nitrogen positions. One of the well-known PDI acceptors is the derivative whose nitrogen positions are functionalized with the 1-ethylpropyl group, giving PDI 1 (Fig. 9). This PDI acceptor normally had a PCE of <1% when blended with donor materials.19 A recent case was reported by Bazan and coworkers, in which PDI 1 was blended with the low bandgap small-molecule donor of (p-DTS(FBTTh2)2) in a 1
:
1 weight ratio, which gives a PCE of 3%.74 Zhan and coworkers revealed that a higher donor-to-acceptor weight ratio of 1.3
:
1 can improve the donor-to-acceptor compatibility and formation of smaller phase domains (20 vs. 40 nm), which led to more efficient quenching of the donor and acceptor fluorescent emission, generating a higher Ge–hmax value. As a result, a higher Jsc, a higher FF and a higher PCE of 5.1% were achieved (Table 1). The good correlation between the acceptor phase size and the experimental Jsc value indicates that the morphology of the acceptor domain plays an important role.75 Using the conjugated polymer of PBDTTT-C-T76 as the blended donor material with 70% PDI 1 (wt%), Keivanidis and coworkers demonstrated a PCE of 3.7% through the use of 0.4% 1.8-diiodooctane (DIO) as the solvent additive.77 Further investigations on the local structural order and molecular packing indicated that electronic connectivity between the large domains of PDI 1 reduced the electron mobility and the local disorder of the PDI domains was essential for efficient electron transport.78,79 From transient absorption pump-probe spectroscopy, Laquai and coworkers illustrated that the charge separation between PDI 1 and PBDTTT-C is strongly field-dependent. Upon excitation, both the PDI and polymer excitons undergo fast charge transfer on a time scale of several tens of picoseconds. However, the photoinduced electron transfer efficiency is only half of that in a polymer:fullerene blend, and a significant fraction of the charges generated at the interfaces from the PDI excitons are lost due to geminate recombination, both of which contribute to the low FF and Jsc. The authors speculated that only about 25% of the initial photoexcitations generates free charges.80 In contrast, Durrant and coworkers demonstrated that both charge separation and recombination dynamics from PDI:PBDTTT-C-T blend are remarkably similar to those from the PC71BM:PBDTTT-C-T system and they suggested that slower charge transport and stronger non-geminate recombination loss during charge collection are responsible for the inferior performance of the PDI based blend.81 In any case, recombination loss is likely a major role in an NF-OSC device, with respect to the fullerene solar cell.
Table 1 Optoelectronic properties, electron–hole mobilities, and cell parameters from PDI 1–4
PDI |
Donor |
Eoptga (eV) |
LUMO/HOMO (eV) |
μe/μh (×10−3 cm2 V−1 s−1) |
Jsc (mA cm−2) |
Voc (V) |
FF (%) |
PCEb (%) |
Ref. |
Optical bandgap obtained from the neat A and D film and presented in the manner of A/D. Under illumination with AM 1.5G, 100 mW cm−2. Calculated from the absorption edge (λabsedge) with the equation of Eoptg = 1240/λabsedge (eV). The value of λabsedge was obtained from the absorption spectrum presented in this reference. |
1 |
DTS(FBTTh2)2 |
—/1.55 |
|
0.17/0.07 |
7.4 |
0.78 |
52 |
3.0 |
74 |
1 |
DTS(FBTTh2)2 |
1.91/1.55 |
−3.82/−5.87 |
4.5/1610 |
9.84 ± 0.20 |
0.80 ± 0.00 |
64.1 ± 0.5 |
5.07 ± 0.10 |
75 |
1 |
PBDTTT-C-T |
—/1.58 |
|
0.006/0.08 |
8.10 ± 0.06 |
0.80 ± 0.01 |
51.9 ± 0.7 |
3.64 ± 0.10 |
76 |
2 |
P3HT |
1.63/2.0 |
−3.95/−5.57 |
0.02/— |
5.3 |
0.61 |
51 |
1.66 |
82 |
3 |
PTB7-Th |
1.85c/1.58 |
−3.82/−5.69 |
— |
10.1 |
0.87 |
46 |
4.1 |
83 |
4 |
PBTI3T |
2.01/1.75c |
−4.01/−6.02 |
2.4/— |
6.6 |
1.0 |
55 |
3.67 |
84 |
The LUMO and HOMO energy levels of PDI 1 are −3.82 and −5.87 eV, respectively.75 With the two aromatics of 4,8-bis(2-(2-ethylhexyl)thienyl)benzo[1,2-b:4,5-b′]dithiophene (2T-BDT) covalently conjugated onto the 1 and 7 positions of the PDI core, Zhan, Yao and coworkers reported a narrow bandgap acceptor molecule, PDI 2, with an optical bandgap of 1.6 eV. Its LUMO energy was reduced down to −3.95 eV, while the HOMO energy was raised to −5.57 eV. The steric effects between the BDT and PDI planes led to the formation of a twisted conformation, improving the solution-processability. When blended with the commercial poly(3-hexylthiophene) (P3HT), a PCE of 1.7% was obtained.82 Sun and coworkers recently reported that the neat film of 1,6,7,12-tetraphenyl substituted PDI 3 had an absorption spectrum very similar to that in solution because the aggregation tendency was significantly suppressed by the introduction of the cyclohexyl side-chains and the formation of a twisted conformation between the phenyl group and the PDI core. PDI 3 gave a PCE of 4% after it was blended with the efficient polymer donor of PTB7-Th and the use of 1% chloronaphthalene (CN) as the solvent additive.83 By introducing four phenyl groups at the 2, 5, 8 and 12-positions, Marks, Wasielewski and coworkers demonstrated that this different molecular strategy can promote the formation of slip-stacking of the PDI π-systems, which prevents the coupling necessary for rapid excimer formation. The resultant acceptor, PDI 4, was blended with the donor polymer PBTI3T, yielding a PCE of 3.7% with a top Voc of 1.0 V.84
PDI dimer. Construction of a dimeric PDI backbone is an alternative approach towards a twisted conformation, and hence, reduction of aggregation tendency, affording solution-processable PDI acceptors. The first reported example was PDI 5 (Fig. 10), in which two PDI units were linked through a single N–N bond. Narayan and coworkers illustrated that the use of PBDTTT-C-T as the blend donor material afforded a PCE of around 3% (Table 2).85 A further study indicated that both polymer and PDI excitons underwent fast dissociation with similar time scales of a few picoseconds at the donor–acceptor interface.42 A recent study by Wang, Hou and coworkers indicated that the replacement of C7H15 with C5H11 in PDI 5 and blending with another high-efficiency donor polymer of PBDT-TS1 gave an improved PCE of 5.58% with an increasing Jsc, Voc and even FF with an inverted cell structure.86 When the polymer PTB7-Th was selected to be blended with PDI 5b, a similar PCE of 6.41% was achieved.87
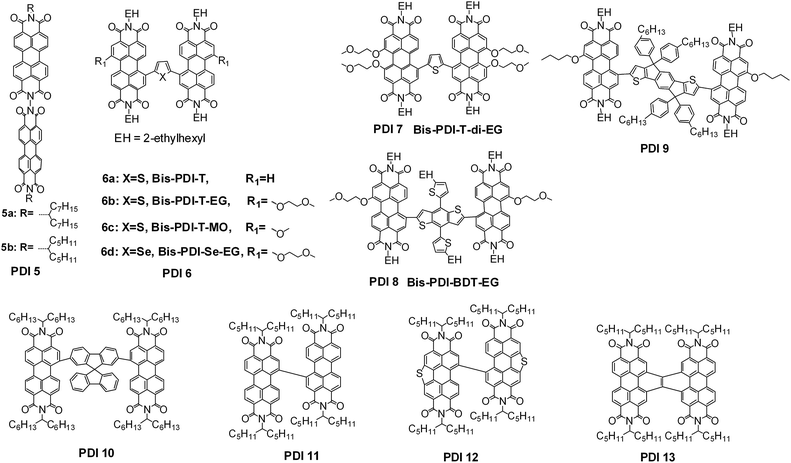 |
| Fig. 10 Chemical structures of several typical PDI dimer acceptors. | |
Table 2 Optoelectronic properties, electron–hole mobilities, and cell parameters from PDI 5–13
PDI |
Donor |
Eoptga (eV) |
LUMO/HOMO (eV) |
μe/μh (×10−3 cm2 V−1 s−1) |
Jsc (mA cm−2) |
Voc (V) |
FF (%) |
PCEb (%) |
Ref. |
Optical bandgap obtained from the neat A and D film and presented in the manner of A/D. Under illumination with AM 1.5G, 100 mW cm−2. Calculated from the absorption edge (λabsedge) with the equation of Eoptg = 1240/λabsedge (eV). The value of λabsedge was obtained from the absorption spectrum presented in this reference. |
5a |
PBDTTT-C-T |
—/1.58 |
−4.06/−6.02 |
0.15/0.27 |
9 |
0.77 |
0.46 |
3.2 |
42 |
5b |
PBDT-TS1 |
2.04/1.51 |
−4.06/−6.02 |
1.2/8.9 |
12.85 ± 0.23 |
0.80 ± 0.01 |
53 ± 2 |
5.45 ± 0.12 |
86 |
5b |
PTB7-Th |
—/1.58 |
−4.06/−6.02 |
1.2/8.9 |
13.12 ± 0.33 |
0.79 ± 0.00 |
60 ± 1 |
6.41 |
87 |
6a |
p-DTS(FBTTh2)2 |
1.82/1.55 |
−3.95/−5.83 |
0.46/230 |
7.31 ± 0.23 |
0.73 ± 0.00 |
65.3 ± 2.2 |
3.47 ± 0.13 |
90 |
6b |
PBDTTT-C-T |
1.76/1.58 |
−3.84/−5.65 |
6.1/10.3 |
12.54 ± 0.17 |
0.84 ± 0.01 |
56.67 ± 2. |
6.00 ± 0.06 |
91 |
6b |
BDT-T-DPP |
1.76/1.64 |
−3.84/−5.65 |
16/22 |
4.66 |
0.92 |
47 |
2.01 |
47 |
6c |
PBDTTT-C-T |
1.76/1.58 |
−3.84/−5.65 |
1.2/5.6 |
9.99 ± 0.18 |
0.78 ± 0.01 |
52.8 ± 2.0 |
4.22 ± 0.12 |
92 |
6d |
PBDTTT-C-T |
1.75/1.58 |
−3.84/−5.61 |
4.7/9.3 |
10.60 |
0.79 |
47.93 |
4.01 |
89 |
7 |
P3HT |
1.76/2.0 |
−3.84/−5.57 |
0.71/230 |
3.83 |
0.67 |
60.0 |
1.54 |
45 |
8 |
P3HT |
1.81/2.0 |
−3.84/−5.48 |
0.34/77 |
5.83 |
0.68 |
49 |
1.85 |
44 |
9 |
BDT-T-DPP |
1.54/1.64 |
−3.83/−5.53 |
0.0023/0.020 |
7.75 |
0.95 |
42 |
3.12 |
93 |
10 |
P3HT |
2.0c/2.0 |
−3.71/−5.71 |
0.071/— |
5.92 |
0.61 |
65 |
2.35 |
43 |
10 |
PffBT4T-2DT |
2.0/1.55 |
−3.83/−5.90 |
0.18/2.3 |
10.7 ± 0.4 |
0.98 ± 0.01 |
57 ± 1 |
6.0 ± 0.3 |
95 |
11 |
PBDTTT-C-T |
2.1/1.58 |
— |
— |
10.58 |
0.73 |
46.8 |
3.63 |
96 |
11 |
PBDTBDD |
2.1/1.77c |
−3.87/−5.95 |
—/1.0 |
8.26 |
0.87 |
61.1 |
4.39 |
97 |
11 |
PTB7-Th |
2.1/1.58 |
−4.04/−6.13 |
0.033/43.6 |
11.98 ± 0.34 |
0.80 ± 0 0.01 |
59 ± 1 |
5.90 |
100 |
12 |
PDBT-T1 |
2.20/1.85 |
−3.85/— |
2.8/1.2 |
11.65 ± 0.21 |
0.90 ± 0.003 |
65.5 ± 0.58 |
6.90 ± 0.15 |
101 |
13 |
PTB7-Th |
2.1/1.58 |
−3.77/−6.04 |
0.34/0.29 |
13.5 ± 0.2 |
0.796 ± 0.005 |
55 ± 1 |
5.94 ± 0.07 |
102 |
Linkage of two PDI units through the bay region is another dimeric way towards twisted, solution-processable PDI dimer acceptors. Zhan, Yao and coworkers reported a series of PDI dimers, PDI 6–8 for example. Using a thienyl unit as the aromatic linker, the LUMO and HOMO energy levels of the resultant PDI dimer were raised from −3.95/−5.83 eV for PDI 6a to −3.84/−5.65 eV for PDI 6b and −3.84/−5.57 eV for PDI 7 after 2 and 4 electron-donating alkoxyl side-chains were introduced on the bay region, respectively, and PDI 7 showed the best compatibility with P3HT, leading to the best PCE of 1.54% of the three dimers.45 The HOMO could be further increased to −5.48 eV when the electron-donating 2T-BDT was selected as the aromatic π-bridge, giving PDI 8, which had a PCE of 1.9% when using P3HT as the donor polymer. It was hypothesized that the highly twisted conformation can provide so-called steric-pairing effects for the self-organization of the twisted PDI dimer molecules.44 The PDI dimer’s LUMO energy can be fixed at −3.84 eV, while its HOMO can be finely tuned from −5.65 up to −5.10 eV by simply extending the oligothienyl π-bridge from 1T to 6T.88 As the blended donor polymer was shifted from P3HT to the low bandgap PBDTTT-C-T, the photoactive layer may absorb more visible and even near infrared solar photons, leading to a dramatic increase of Jsc, and the lower lying LUMO of PBDTTT-C-T over P3HT contributes to a higher Voc. As a result, both PDI 6b41 and 6d89 gave a PCE of 4%. Using 2T-BDT-mediated diketopyrrolopyrrole (DPP) dimeric molecules as the donor materials, PDI 6b gave a PCE between 1.3 and 2.0%.47,53 When the donor molecule was switched to high-efficiency p-DTS(FBTTh2)2, PDI 6a yielded a PCE of 3.7%.90 Although the twisted dimeric conformation can improve the solution-processability and reduce the acceptor domain size close to the effective exciton diffusion length, the ordered packing of the dimeric molecules is compromised, and this leads to a lower electron mobility and increased recombination loss in the resulting solar cells. To improve the dimeric acceptor packing, Zhan, Yao and coworkers recently demonstrated that treatment of the photoactive layer with the vapour of the processing host solvent, i.e. 1,2-dicholobenzene (o-DCB), in a fully sealed Petri dish can significantly increase both the Jsc and FF values. This is because the slow evaporation of the host solvent is helpful for the acceptor molecules to self-organise and phase separate with the blend donor polymer, leading to the increase of electron mobility and reduction of recombination loss. Following such a solvent vapor annealing (SVA) process, the PDI 6b:PBDTTT-C-T system yielded an optimum PCE value of 6.1%.91 Besides the film morphology in the photoactive layer, Zhan, Yao and coworkers developed a system based on a PDI 6c:PBDTTT-C-T combination and showed that the donor-to-acceptor compositions in the air and buried surfaces of the photoactive layer can be finely tuned with the amount of solvent additive, DIO, and it was found that the acceptor-to-donor abundance in the buried surface played a very important role in affecting the injection and extraction of the free electrons or holes, which then contributed to the cell’s Jsc and FF.92 By using indaceno[1,2-b:5,6-b′]dithiophene (IDT) as the aromatic π-bridge, Zhan and coworkers presented another solution-processable twisted PDI dimer (PDI 9), which showed a PCE of 3.1% when using a 2T-BDT-mediated DPP dimer as the donor material.93 When the IDT was changed to the thienyl bridge, the resulting PDI dimer produced a PCE of 3.6% with PBDTTT-C-T as the donor polymer.94 The different PCE values of 3.6% for this PDI dimer and the value of 6.1% achieved for PDI 6b with the same polymer as the donor and with an identical cell structure suggest that the additional oxygen atom from the 2-methoxylethoxyl group in PDI 6b could be important in realizing efficient NF-OSCs.
Pei, Zhao and coworkers reported a series of PDI dimers featuring arylene bay linkers, such as spirobifluorene-2,7′-diyl (PDI 10). This PDI dimer showed an absorption spectrum similar to that in solution, indicating minimal intermolecular aggregation in the thin film. The best inverted cell based on PDI 10:P3HT yielded a PCE of 2.35%.43 As the blend donor material was changed into a high-efficiency polymer PffBT4T-2DT, Yan and coworkers reported that PDI 10 achieved a PCE of 6.3%.95 Wang and coworkers designed another series of bay-linked (singly, doubly and triply) PDI dimers featuring branched alkyl chains at the nitrogen positions. The bay-linked PDI 11 enabled a PCE of 3.63%,96 4.39%,97 4.48%,98 and 3.12%,99 respectively utilizing PBDTTT-CT, PBDTBDD, PTB7-Th, and P(IID-DTC) as the blended polymer donor. A PCE of 5.90% was reported from blend of PDI 11:PTB7-Th using an inverted cell structure with a self-assembling monolayer of fullerene atop the ZnO interlayer.100 During preparation of this review paper, Wang and coworkers reported another PDI dimer, PDI 12, with two S atoms fused on the bay region of the two PDI units. A PCE of 7.16% was achieved when blended with the polymer donor of PDBT-T1.101 Nuckolls and coworkers designed a twisted PDI dimer formed by the fusion of two PDI units with a carbon–carbon double bond bridge (PDI 13), which achieved an efficiency of 6.05% when using PTB7-Th as the donor polymer and using 1% DIO plus 1% CN as the cosolvent additive.102
PDI trimer and tetramer. The spherical shape of the fullerene π-system is assumed to be capable of aligning with the donor π-plane in a three-dimensional (3-D) manner, which may decrease the Coulombic barrier for charge separation due to enhanced entropic effects and enables isotropic charge transport.103 For this reason, PDI trimers and even tetramers with a 3-D structure were synthesized. Zhan, Yao and coworkers reported a star-shaped PDI trimer, PDI 14, in which three PDI units were covalently linked via a triphenylamine bridge (Fig. 11). This PDI trimer gave a PCE of 1.85% when blended with PBDTTT-C-T (Table 3).104 Zhan and coworkers also presented a similar nonplanar, star-shaped PDI trimer, PDI 15, whose bay region was functionalized with butyloxyl side-chains. When using PBDTTT-C-T as the blended donor material, the device gave an optimum PCE of 3.22%.105 Yan and coworkers reported a tetraphenylethylene (TPE) core-based PDI tetramer (PDI 16). Its four phenyl rings were highly twisted due to strong steric hindrance: they all tilted by about 50° relative to the plane of the central double bond and formed a “four-wing propeller-shape” molecular structure. Due to their highly twisted molecular structure, the TPE-based molecule exhibited weak intermolecular interactions and thus excellent solubility in organic solvents. In the blend with PTB7-Th, the best cell gave a PCE of 5.53%.106 When the tetraphenylethylene core was changed into a tetraphenylmethane core, the resulting PDI tetramer (17) had a PCE of 4.3% using PffBT4T-2DT as the donor polymer.107 This PCE value is lower than that from PDI 10 also using PffBT4T-2DT as the donor. At the same time, Zhang and coworkers also designed another tetraphenylmethane-mediated PDI tetramer (18), which exhibited a PCE of 2.73% when using PBDTTT-C-T as the donor polymer.108 From comparisons of the PCE values achieved from the trimer and tetramer of PDI and those from the PDI dimer, it seems that the PDI dimer can give a better cell performance when blended with the same donor molecule.
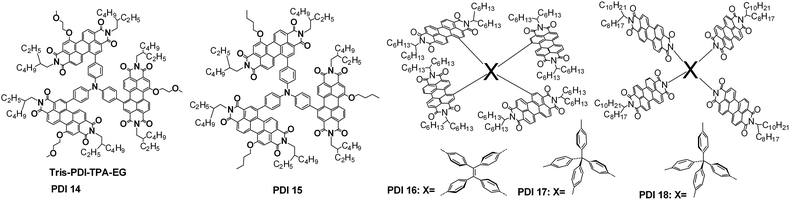 |
| Fig. 11 Chemical structures of the PDI trimer and tetramer acceptors. | |
Table 3 Optoelectronic properties, electron–hole mobilities, and cell parameters from PDI 14–18
PDI |
Donor |
Eoptga (eV) |
LUMO/HOMO (eV) |
μe/μh (×10−3 cm2 V−1 s−1) |
Jsc (mA cm−2) |
Voc (V) |
FF (%) |
PCEb (%) |
Ref. |
Optical bandgap obtained from the neat A and D film and presented in the manner of A/D. Under illumination with AM 1.5G, 100 mW cm−2. |
14 |
PBDTTT-C-T |
1.71/1.58 |
−3.75/−5.60 |
— |
5.89 |
0.91 |
34 |
1.85 |
104 |
15 |
PBDTTT-C-T |
1.76/1.58 |
−3.70/−5.40 |
0.010/0.53 |
11.27 |
0.87 |
33 |
3.22 |
105 |
16 |
PTB7-Th |
2.05/1.58 |
−3.72/−5.77 |
1/— |
11.7 |
0.91 |
52 |
5.53 |
106 |
17 |
PffBT4T-2DT |
2.25/1.55 |
−3.75/−6.00 |
0.28/— |
9.2 |
0.96 |
49 |
4.3 |
107 |
18 |
PBDTTT-C-T |
2.14/1.58 |
−3.82/−5.96 |
0.0018/0.056 |
7.83 |
0.77 |
45 |
2.73 |
108 |
3.2. Non-PDI, large fused-ring π-system based small molecules
Pentacene is a typical p-type organic semiconductor, while octafluoropentacene derivatives such as M1 with two silylethyne substituted groups, as shown in Fig. 12, have low-lying LUMO and HOMO levels, and can be used as non-fullerene acceptors, although they show an inferior performance when blended with a DPP-based small molecule donor (Table 4).109 Wang and coworkers reported that quinacridone derivatives such as M2, which also contains five fused six-membered rings, gave a PCE value of 1.57% when it was blended with the commonly used donor polymer, P3HT.110 Pei and coworkers reported a series of fluoranthene-fused imide derivatives, for example, M3. Their LUMO level could be tuned between −3.40 and −3.48 eV by changing the peripheral aryl substituted groups. Among them, that substituted with o-methylphenyl groups had a LUMO level of −3.43 eV and yielded a PCE value of 2.90% when using P3HT as the donor and following a solvent and subsequent thermal annealing process.111,112 We noted that this PCE value of 2.90% was interesting because it was obtained by using a wide bandgap organic acceptor (2.83 eV) and the wide bandgap donor polymer P3HT.
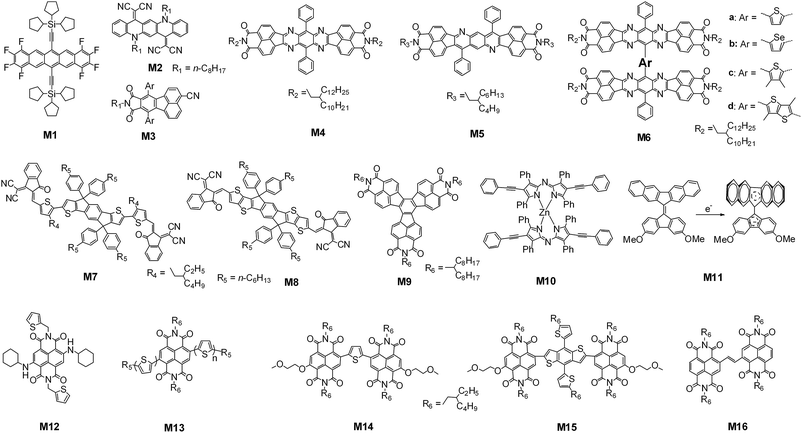 |
| Fig. 12 Chemical structures of the typical reported non-PDI, large fused-ring π-system based small-molecule acceptors. | |
Table 4 Optoelectronic properties, electron–hole mobilities, and cell parameters for M1–16
Acceptor |
Donor |
Eoptga (eV) |
LUMO/HOMO (eV) |
μe/μh (×10−3 cm2 V−1 s−1) |
Jsc (mA cm−2) |
Voc (V) |
FF (%) |
PCEb (%) |
Ref. |
Optical bandgap obtained from the neat A and D film and presented in the manner of A/D. Under illumination with AM 1.5G, 100 mW cm−2. Calculated from the absorption edge (λabsedge) with the equation of Eoptg = 1240/λabsedge (eV). The value of λabsedge was obtained from the absorption spectrum presented in this reference. Estimated using ultraviolet photoelectron spectroscopy (UPS) and optical bandgap data. |
M1 |
C6PT2C6 |
1.7c/1.7c |
−3.71/−5.71 |
0.015/0.0015 |
0.66 ± 0.10 |
0.91 ± 0.13 |
45 ± 9 |
0.28 ± 0.10 |
109 |
M2 |
P3HT |
1.8c/2.0 |
−4.1/−5.9d |
0.11/— |
5.72 |
0.48 |
57 |
1.57 |
110 |
M3 |
P3HT |
2.83/2.0 |
−3.44/−6.27 |
0.009/0.15 |
6.35 |
0.95 |
48 |
2.90 |
112 |
M4 |
PSEHTT |
2.2 c/1.65c |
−3.6/−5.8 |
0.00035/0.18 |
3.16 |
0.94 |
49 |
1.39 ± 0.05 |
114 |
M5 |
PTB7-Th |
2.22/1.58 |
−3.6/−6.0 |
0.06/0.068 |
9.02 |
0.96 |
35 |
2.91 ± 0.08 |
113 |
M6a |
PSEHTT |
1.8c/1.65c |
−3.8/−5.8 |
0.12/0.28 |
10.14 |
0.86 |
58 |
4.91 ± 0.13 |
114 |
M6c |
PSEHTT |
1.91/1.65c |
−3.70/−5.82 |
33.2/0.157 |
12.10 ± 0.36 |
0.91 ± 0.004 |
56 ± 1 |
6.18 ± 0.13 |
115 |
M7 |
PTB7-Th |
1.57/1.58 |
−3.82/−5.42 |
0.10/0.45 |
13.05 ± 0.35 |
0.967 ± 0.002 |
47.3 ± 4 |
6.08 ± 0.20 |
119 |
M8 |
PTB7-Th |
1.59/1.58 |
−3.83/−5.48 |
0.11/0.043 |
14.21 |
0.81 |
59 |
6.8 |
120 |
M9 |
P3HT |
2.20/20 |
−3.61/−5.81 |
— |
4.87 |
0.58 |
57 |
1.6 |
121 |
M10 |
P3HT |
1.55/2.0 |
−3.85/−5.60 |
0.19/0.21 |
8.8 ± 0.5 |
0.76 ± 0.01 |
57 ± 3 |
3.90 ± 0.20 |
122 |
M11 |
P3HT |
2.18/2.0 |
−3.24/−5.18 |
— |
3.9 |
1.10 |
40 |
1.7 |
123 |
M12 |
P3HT |
1.7c/2.0 |
−3.71/−5.69 |
0.23/0.13 |
2.99 ± 0.09 |
0.70 ± 0.02 |
44 ± 1 |
0.94 ± 0.03 |
124 |
M13 |
P3HT |
1.57/2.0 |
−4.05/−5.53 |
— |
3.43 |
0.82 |
0.53 |
1.45 |
125 |
M14 |
PBDTTT-C-T |
1.70/1.58 |
−3.80/−5.88 |
— |
2.70 |
0.96 |
51 |
1.31 |
126 |
M15 |
PBDTTT-C-T |
1.61/1.58 |
−3.78/−5.46 |
— |
3.26 |
0.95 |
40 |
1.24 |
126 |
M16 |
PTB7 |
2.4c/1.63 |
−4.35/−6.74 |
0.017/— |
5.47 |
0.74 |
59.1 |
2.41 |
127 |
Jenekhe and coworkers reported tetraazabenzodifluoranthene diimide (BFI) and bis(naphthalene imide)diphenylanthrazoline (BNIDPA) based organic acceptors. The conjugated benzodifluoranthene diimide can support efficient electron delocalization and polaron formation. The LUMO and HOMO energies of BNIDPA (M5) were both close to the values of the monomeric BFI (M4). However, the M5:PTB7-Th blend had a much higher electron mobility, and hence a much higher Jsc value than that of M4:PSEHTT (poly[(4,4′-bis(2-ethylhexyl)dithieno[3,2-b:2′,3′-d]silole)-2,6-diyl-alt-(2,5-bis(3-(2-ethylhexyl)thiophen-2-yl)thiazolo[5,4-d]thiazole)]). M5 gave a PCE value of 3%,113 whereas M4 only had a PCE of 1.5%.114 When two BFI monomers were covalently linked together via a thienyl bridge, the resulting dimer, M6a, had a PCE of 5%, also due to the higher electron mobility and again the higher hole mobility.114 The dihedral angle between the two BFI planes can be tuned up to 62° by using the 3,4-dimethylthienyl bridge. The resulting dimer can give an even higher Jsc value and a higher PCE of 6.2% was obtained.115
Indaceno[1,2-b:5,6-b0]dithiophene (IDT)116 and indacenodithieno[3,2-b]thiophene (IDTT)117 are two typical ladder-type electron-donating groups, in which the two thiophene/bithiophene subunits are covalently fastened to the central phenyl core via two sp3 carbon atoms, forming coplanar large fused-ring π-systems. The four bulky p-(n-hexyl)aryl groups are introduced onto the sp3 carbon atom as the peripheral substituents for tailoring the solution-processability. By covalently conjugating the electron-donating IDT or IDTT with the electron-accepting 2-(3-oxo-2,3-dihydroinden-1-ylidene)malononitrile, Zhan and coworkers reported two A–D–A based organic acceptors, M7 and M8. When using PTB7-Th as the donor polymer and PDIN (a PDI monomer whose nitrogen positions were functionalized with 3-(N,N-dimethyl)propyl units)118 as the cathode layer, M7 and M8 had a PCE of 6.31% and 6.80%, respectively.119,120 Wudl and coworkers reported a coplanar, star-shaped decacyclene triimide based organic acceptor, M9, with three naphthaleneimide subunits fused with a central phenyl core. It had a PCE of 1.6% with P3HT as the blend polymer donor.121
Bis[2,6-diphenylethynyl-1,3,7,9-tetraphenylazadipyrromethene]zinc(II), M10, reported by Sauvé and coworkers, is an interesting non-fullerene acceptor in which the two azadipyrromethene planes are highly twisted with the conjugated arms pointing in four directions. Its low bandgap, intense absorption and low-lying LUMO and HOMO levels give it the potential to be applied as an electron accepting material. The best M10:P3HT blend based solar cell had a PCE of 4.10% and the control cell of the PC61BM:P3HT blend had an optimum PCE of 3.7%.122 Another interesting case is 9,9′-bifluorenylidene based derivatives, such as M11, reported by Wudl and coworkers. It was speculated that the addition of an electron across the 9,9′-double bond is highly favourable because of steric strain relief and a gain in aromaticity to form a 14-pi-electron system. Therefore, although M11 had high-lying LUMO and HOMO energy levels (−3.24/−5.18 eV), it could act as an electron-accepting material and yielded a PCE of 1.7% when using P3HT as the donor.123
Naphthalene diimides (NDIs) are another kind of traditional n-type organic semiconductor. NDIs normally show an absorption band with an optical bandgap of 3 eV. One of the strategies to narrow the bandgap is to introduce electron-donating groups, favouring the intramolecular charge-transfer (ICT) transition from the peripheral unit to the NDI core. Recently, Sauvé and coworkers reported such an NDI derivative, M12, by introducing two N-cyclohexylamino groups onto the bay region of the NDI core. The resulting molecule had an optical bandgap down to 1.7 eV and its LUMO and HOMO energy levels were −3.71 and −5.69 eV, respectively. The ICT band is even stronger than the π–π* transition of the NDI core. Because the absorption of P3HT just follows between the ICT and the π–π* absorption of M12, the M12:P3HT blend thus had a wide solar spectrum coverage from 300 to 720 nm, and the solar cell based on this blend had an optimum PCE value of 1% after using 0.2% DIO as the additive and annealing below 100 °C for 1 h.124 Jenekhe and coworkers reported another series of NDI monomer-based acceptors by covalently linking two oligothiophene units onto the 2 and 6-positions. The large oligothiophene size led to a red-shift of the onset of the resulting ICT absorption with the optical bandgap changing from 2.1 to 1.4 eV. Unfortunately, the ICT absorption was much weaker than the absorption of the NDI core. Of the molecules, M13 had a PCE of 1.5% when using P3HT as the donor, TPBI as the cathode layer, 0.2% DIO as the additive and thermally annealing at 100 °C for 10 min.125 Recently, Zhan and coworkers presented two NDI dimers, M14 and M15, in which two NDI units were covalently linked with a bridge of thiophene or 2T-BDT. Compared to the thienyl bridged dimer, that mediated by 2T-BDT had a lower bandgap and its ICT absorption extended to 770 nm. However, the ICT absorption was still weak, which corresponded to a lower Jsc. When blended with PBDTTT-C-T, M14 and M15 yielded a Voc of 0.95–0.96 V and PCE values of 1.31% and 1.24%, respectively.126 Russell and coworkers synthesized another NDI dimer (M16) with a carbon–carbon double bond as the bridge. The M16:PTB7 blend based solar cell had an optimum PCE of 2.41% when using 0.5% DIO as the additive.127
3.3. Other conjugated π-system based small molecules
Compared to the PDI and non-PDI large fused-ring π-systems, there is another class of non-fullerene small-molecule acceptors which are normally constructed by covalently linking relatively small electron-donating and electron-accepting π-aromatics such as thiophene, benzene, vinazene, phthalimides, naphthalimides, thienoimide and indandione together. For these kinds of molecules, the formation of a coplanar backbone seems to be important for the achievement of high-efficiency charge transportation and electric performance.
Fréchet and coworkers reported that the 2-ethylhexyl dicyanoimidazol-2-yl (vinazene)-based molecule, M17a (Fig. 13), displayed an average PCE of 1.4% (Table 5) when it was blended with the polymer donor of poly[3-(4-n-octyl)phenylthiophene] (POPT).128 When blended with a DPP based small molecular donor, which was terminated by benzofuran groups, M17b had a higher PCE of 1.10% than M17a (0.80%).129 Sellinger and coworkers modified the molecular structure by replacing flanked vinazene with phthalimides or naphthalimides. The naphthalimide terminated molecule was typically amorphous in a solid film. However, the phthalimide terminated molecule (M18) showed significant crystallization in a spin-coated film and had a higher PCE value (2.54% vs. 0.12%) than the naphthalimide terminated molecule when mixed with P3HT. This was rationalised by M18 possessing a more coplanar conjugated backbone, whereas the steric interactions between neighbouring hydrogen atoms in the naphthyl and vinyl moieties induced a 27.3° twist for the naphthalimide terminated backbone.130 The PCE from the M18:P3HT based solar cell was further improved to 3.7% through optimization of the synthetic and purification procedures.131 Fréchet and coworkers replaced the phthalimides with thienoimides and used a thienyl bridge instead of the CH
CH one, affording molecule M19. M19 exhibited a broader absorption band and higher extinction coefficients than the phthalimide terminated control molecule, and had a higher PCE (2.4% vs. 0.85%) when blended with a DPP small molecule with perylene side-arms.132
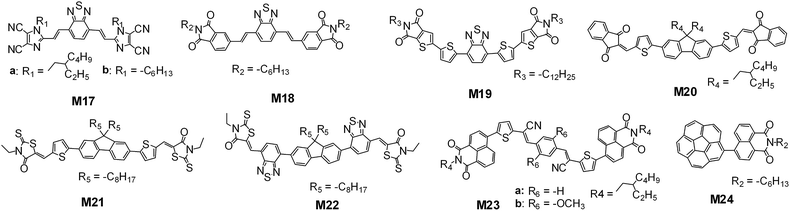 |
| Fig. 13 Chemical structures of the reported typical small π-aromatic conjugation based small-molecule acceptors. | |
Table 5 Optoelectronic properties, electron–hole mobilities, and cell parameters from M17–24
Acceptor |
Donor |
Eoptga (eV) |
LUMO/HOMO (eV) |
μe/μh (×10−3 cm2 V−1 s−1) |
Jsc (mA cm−2) |
Voc (V) |
FF (%) |
PCEb (%) |
Ref. |
Optical bandgap obtained from the neat A and D film and presented in the manner of A/D. Under illumination with AM 1.5G, 100 mW cm−2. Calculated from the absorption edge (λabsedge) with the equation of Eoptg = 1240/λabsedge (eV). The value of λabsedge was obtained from the absorption spectrum presented in this reference. |
M17a |
POPT |
2.43/1.8 |
−3.5/−6.0 |
— |
5.50 |
0.62 |
40 |
1.41 |
128 |
M17b |
DPP |
2.43/1.75 |
−3.5/−6.0 |
0.063/— |
2.76 |
1.08 |
37 |
1.10 |
129 |
M18 |
P3HT |
2.34/2.0 |
−3.30/−5.77 |
— |
4.7 |
0.96 |
56 |
2.54 |
130 |
M18 |
P3HT |
2.34/2.0 |
−3.30/−5.77 |
— |
6.5 |
0.94 |
61 |
3.7 |
131 |
M19 |
DPP-Py |
1.89/1.72 |
−4.10/−5.99 |
— |
3.72 |
1.05 |
60 |
2.33 ± 0.05 |
132 |
M20 |
P3HT |
2.07c/2.0 |
−3.95/−5.95 |
|
3.82 |
0.95 |
67 |
2.12 ± 0.18 |
133 |
M21 |
P3HT |
1.55/2.0 |
−3.53/−5.58 |
|
5.7 |
1.03 |
52 |
3.08 |
134 |
M22 |
P3HT |
2.14/2.0 |
−3.57/−5.70 |
0.026/— |
7.95 |
0.82 |
63 |
4.11 |
135 |
M23a |
P3HT |
2.22/2.0 |
−3.42/−5.90 |
0.02/5.65 × 10−5 |
8.04 |
0.73 |
46 |
2.51 ± 0.11 |
136 |
M23b |
DTS(FBTTh2)2 |
2.22/1.55 |
−3.42/−5.90 |
0.175/0.0845 |
9.62 ± 0.269 |
0.85 ± 0.005 |
64 ± 1.4 |
5.30 ± 0.091 |
137 |
M24 |
P3HT |
3.04/2.0 |
−3.24/— |
0.132/— |
2.75 |
0.82 |
46 |
1.03 |
138 |
Watkins and coworkers reported another different molecule, M20, in which a fluorene was end-capped with electron-accepting 2,3-dihydro-1H-indene-1,3-dione units via the thienyl bridge. The M20:P3HT based solar cell yielded a PCE of 2.4%.133 Lim and coworkers replaced the indene-1,3-dione units with rhodanine, and synthesized a narrow bandgap molecule, M21, which had a PCE of 3.08%, also using P3HT as the donor.134 Holliday and coworkers utilized the electron-accepting benzothiadiazole to replace the electron-donating thienyl bridge in M21. The resulting molecule M22 had a PCE of 4.11%, again using P3HT as the donor.135 Park and coworkers used two naphthalimide units to cap a dicyanodistyrylbenzene core with two thienyl bridges. The resulting molecules M23a and M23b had a PCE of 2.71% with P3HT as the donor136 and a PCE of 5.44% with the low bandgap small molecule of p-DTS(FBTTh2)2 as the blended donor.137 By covalently linking Corannulene with one naphthalimide unit, Cao and coworkers reported another molecule, M24, which had 1% efficiency using P3HT as the donor.138
3.4. Vacuum-deposited small molecules
Subphthalocyanines (SubPcs) are a class of phthalocyanine-related organic semiconductors (Fig. 14). A SubPc molecule consists of three instead of four diiminoisoindoline units, which are arranged around a boron atom.139 In 2011, Torres, Heremans and coworkers reported a 2.5% efficiency vacuum deposited bilayered non-fullerene organic solar cell based on a fluorinated fused subphthalocyanine dimer and SubPc (M26). With the addition of a C60 layer atop the fluorinated fused dimer layer, an efficiency of 4% was obtained.140 Bender and coworkers reported that the bilayer cell based on pentafluorophenoxy substituted boron subphthalocyanine (M28) (40 nm) and SubPc (10 nm) had an efficiency of 1% (Table 6).141 The boron subnaphthalocyanine (M27) had a red-shifting absorption band and edge (730 nm), compared to M26. The absorption of the hexachloro-substituted SubPc M29 was complementary to that of M27. Torres, Heremans and coworkers reported that a 6.4% efficiency was obtained from bilayered M29/M27 using a blend of bathocuproine (BCP) and C60 as the cathode layer.142 Recently, Heremans and coworkers achieved a PCE of 8.4% from a three-layered solar cell by exploiting the cascade energy transfer from a hexathiophene donor layer to the M26 and M27 layers.143
 |
| Fig. 14 Chemical structures of the reported vacuum-deposited small-molecule acceptors. | |
Table 6 Optoelectronic properties, electron–hole mobilities, and cell parameters from PDI 26–32
Acceptor |
Donor |
Eoptga (eV) |
LUMO/HOMO (eV) |
Jsc (mA cm−2) |
Voc (V) |
FF (%) |
PCEb (%) |
Ref. |
Optical bandgap obtained from the neat A and D film and presented in the manner of A/D. Under illumination with AM 1.5G, 100 mW cm−2. Calculated from the absorption edge (λabsedge) with the equation of Eoptg = 1240/λabsedge (eV). The value of λabsedge was obtained from the absorption spectrum presented in the reference. Estimated using ultraviolet photoelectron spectroscopy (UPS) and optical bandgap data. |
M28 |
M26 |
—/1.9 |
−3.6/−5.7 |
2.06 ± 0.71 |
1.06 ± 0.03 |
43.2 ± 0.9 |
0.94 ± 0.33 |
141 |
M29 |
M27 |
—/1.7 |
— |
9.0 |
1.03 |
71 |
6.4 |
142 |
M26/M27 |
α-6T |
1.9c/1.7/2.17c |
— |
14.55 |
0.96 |
61.0 |
8.40 |
143 |
M30 |
M26 |
2c/1.9 |
−4.23/−6.28 |
2.34 |
1.38 |
40 |
1.28 |
144 |
M31 |
ZnPc |
2.10/— |
−3.88/−6.0 |
3.45 |
0.59 |
63 |
1.30 |
145 |
M32 |
DBP |
2.07c/1.9 |
−4.10/−6.4d |
6.2 |
0.88 |
68 |
3.6 |
146 |
Sullivan and coworkers reported that a bilayered cell based on bis(diethyl-barbituric acid)-2,2′-bithiophene (M30) and M26 had an efficiency of 1.3%.144 Recently, Thompson and coworkers reported a zinc chlorodipyrrin based organic acceptor (M31). They demonstrated that the symmetry-broken zinc chlorodipyrrin was helpful to achieve a high Voc value. The M31/tetraphenyldibenzoperyflanthrene cell had a Voc of 1.33 V and the estimated CT state energy was 1.70 eV.145 Nielsen and coworkers presented a star-shaped organic acceptor, M32. Compared to the mother unsubstituted truxenone core, the introduction of one, two and three ethyl cyanoacetate groups led to the truxenone core bending by 1.46°, 2.01°, and 2.68°, respectively. The best bilayered cell had a PCE of 1.30% with ZnPc as the donor layer.146
4. Polymer non-fullerene acceptors
D–A conjugation is a general strategy to polymer acceptors. Similarly to the small molecules, the rylene diimide based systems are widely investigated polymer acceptors. Bao, Zhao and coworkers reported 4.21% efficiency from a PDI-thiophene based polymer acceptor, P1 (Fig. 15), with an isoindigo-bithiophene based polymer (PiI-2T-PS5) as the blended donor material (Table 7).147 When using P3HT as the donor, the PDI-bithiophene based polymer (P2) had a PCE of 2.17%.148 Zhan and coworkers reported the PDI-dithienothiophene based polymer (P3) in 1997, which had a PCE of 1% using bi(thienylenevinylene)-substituted polythiophene as the donor.52 The same polymer acceptor had a PCE of 3.45% when using PBDTTT-C-T as the donor and a PDI derivative as the additive.149 Yu and coworkers used an electron-accepting moiety, 4,10-bis(2-butyloctyl)thieno[2′,3′:5,6]pyrido[3,4-g]thieno[3,2-c]isoquinoline-5,11-dione (TPTI),150 instead of an electron-donating unit to conjugate the PDI chromophore. The resulting polymer acceptor had a PCE of 1% when PTB7 was used as the donor material.151 When TPTI was replaced with its isomer, 5,11-bis(2-butyloctyl)dihydrothieno[2′,3′:4,5]pyrido[2,3-g]thieno[3,2-c]quinoline-4,10-dione (TPTQ), the resulting polymer acceptor, P4, had a PCE of 3.22% when blended with PTB7-Th.152 The LUMO energy level of PDI based polymers was normally in the region of −3.8 to −4.0 eV. When two thienyl units were fused with the 1,12- and 6,7-positions of the PDI core,153 or the diimide functionalities were converted into tetracarboxylic tetraester groups,154 the LUMO energy of the resulting PDI polymer could be up to about −3.5 eV.
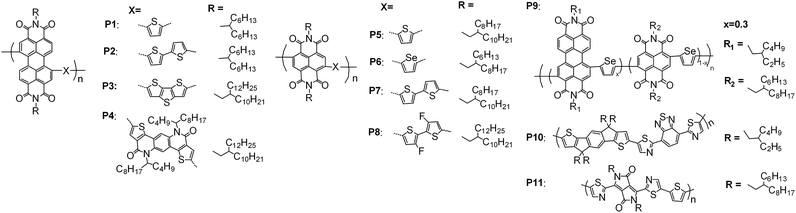 |
| Fig. 15 Chemical structures of the typical reported PDI, NDI and other electron-accepting unit based polymer acceptors. | |
Table 7 Optoelectronic properties, electron–hole mobilities, and cell parameters for polymer acceptors P1–P11
A |
D |
Mna (kDa) |
PDIb |
Eoptgc A/D (eV) |
LUMO/HOMO (eV) |
μe/μh (×10−3 cm2 V−1 s−1) |
Jsc (mA cm−2) |
Voc (V) |
FF (%) |
PCEd (%) |
Ref. |
Number-average molecular weight of the polymer acceptor. Polydispersity index of the polymer acceptor. Optical bandgap obtained from the neat D and A film and presented in the manner of A/D. Under illumination wit AM 1.5G, 100 mW cm−2. Calculated from the absorption edge (λabsedge) with the equation of Eoptg = 1240/λabsedge (eV). The value of λabsedge was obtained from the absorption spectrum presented in this reference. By inverse photoemission spectroscopy (IPES). By UPS. |
P1 |
PiI-2T-PS5 |
20.5 |
2.1 |
1.8/1.6 |
−3.80f/−5.72g |
0.02/0.2 |
8.77 ± 0.29 |
1.04 ± 0.01 |
46 ± 1 |
4.21 ± 0.10 |
147 |
P2 |
P3HT |
15.1 |
1.81 |
1.7e/2.0 |
−3.8/−5.5 |
0.5/— |
7.65 |
0.52 |
55 |
2.17 |
148 |
P3 |
PBDTTT-C-T |
— |
— |
—/1.58 |
−3.9/−5.9 |
0.0135/— |
8.55 |
0.752 |
51.5 |
3.45 |
149 |
P4 |
PTB7-Th |
21.6 |
2.83 |
1.74/1.58 |
−3.97/−5.97 |
0.11/0.84 |
7.72 ± 0.12 |
0.70 ± 0.01 |
57 ± 0.8 |
3.08 ± 0.14 |
152 |
P5 |
PTB7-Th |
48.2 |
2.1 |
1.85/1.58 |
3.79/−5.64 |
0.084/0.28 |
13.46 |
0.79 |
56 |
5.96 |
155 |
P6 |
PTB7-Th |
28.4 |
1.5 |
1.76/1.59 |
−3.84/−6.0 |
7.25/0.274 |
18.61 ± 0.21 |
0.80 ± 0.00 |
48 ± 1 |
7.21 ± 0.24 |
46 |
P7 |
DTP-DPP |
— |
— |
1.45/1.52 |
−4.1/−5.7 |
—/— |
10.14 |
0.82 |
58 |
4.82 |
161 |
P7 |
PTB7-Th |
— |
— |
1.38e/1.58 |
−4.3/−5.9 |
4.1/0.96 |
13.0 ± 0.22 |
0.795 ± 0.00 |
53.4 ± 0.1 |
5.50 ± 0.08 |
166 |
P8 |
PTB7-Th |
41.4 |
1.6 |
1.55e/1.58 |
−3.9/−5.8 |
0.49/0.55 |
13.53 |
0.81 |
62 |
6.71 |
167 |
P9 |
PBDTTT-C-T |
37.5 |
1.4 |
1.77/1.58 |
−3.9/−5.95 |
1.0/2.6 |
18.22 ± 0.28 |
0.78 ± 0.002 |
43 ± 1 |
6.17 ± 0.10 |
172 |
P10 |
P3HT |
14.3 |
1.82 |
1.98/2.0 |
−3.45/−5.43 |
—/— |
2.6 |
1 |
0.45 |
1.18 |
174 |
P11 |
PDDP5T |
93.3 |
3.05 |
1.44/1.30 |
−4.00/−5.63 |
—/— |
6.9 |
0.81 |
0.51 |
2.9 |
175 |
NDI based polymers are another interesting class of polymer acceptors. Recently, Kim and coworkers used thiophene as the conjugated electron-donating moiety and reported a NDI-thiophene based polymer, P5, which had a PCE of 6% when blended with PTB7-Th.155 Based on P5, Kim, Yoo and coworkers demonstrated that the polymer donor weight of PPDT2FBT had a significant effect on the polymer aggregation and phase segregation, and, hence the electric performance of the all-polymer organic solar cell. From this D–A system, they found that a high molecular weight can afford the most efficient cell device.156 Jenekhe and coworkers reported a NDI-selenophene based polymer, P6, which had a PCE value of 3.3%
157 and 7.7%
46 when using PSEHTT and PTB7-Th as the polymer donor, respectively. The NDI-bithiophene based polymer, P7, is commercially available. A PCE value of 6.4% was published by Polyera for this polymer acceptor in 2013.12 The non-fullerene small molecular organic solar cells (NF-SMSCs) based on the blends of P7 (polymer acceptor) and small molecule donors such as p-DTS(FBTTh2)2158,159 or electron-donating moiety-mediated DPP dimers160,161 showed a PCE value of 2–5%. The all-polymer organic solar cells (all PSCs) with P7 as the polymer acceptor exhibited a PCE of 2–5.5%, with polymers such as PTB7,162 PTQ1 (poly[2,3-bis(3-octyloxyphenyl)quinoxaline-5,8-diyl-alt-thiophene-2,5-diyl]),163 BFS4 (a dithienyl-benzo[1,2-b:4,5-b]dithiophene/5-fluoro-2,1,3-benzothiadiazole co-polymer),164 NT (a naphthobisthiadiazole-benzodithiophene co-polymer),165 and PTB7-Th166 used as the donor material, respectively. By fluorinating the bithiophene unit in P7 and further converting the side-chains on the NDI units into 2-decyltetradecyl, Jen and coworkers reported a new NDI-bithiophene based copolymer, P8, from which a PCE of 6.7% was obtained with PTB7-Th as the polymer donor.167 Other NDI based polymer acceptors, with thienylene–vinylene–thienylene168 and N-(1-octylnonyl)carbazole,169 for example, as the conjugated electron-donating unit were reported to have a PCE of 4.3% and 3.7%, respectively, with PTB7-Th and another low-bandgap co-polymer of VVT7 as the donor material. Besides this, naphthobisthiazole diimide based copolymers were synthesized and investigated as polymer acceptors with PSEHTT as the donor, giving an optimum PCE of 1.4%.170 With respect to the NDI-selenophene/NDI-selenophene random copolymer (with 2-butyloctyl and 2-decyltetradecyl on the NDI nitrogen positions, respectively),171 the PDI-selenophene/NDI-selenophene random copolymer (P9) had a higher electron and hole mobility when blended with PBDTTT-C-T, and a PCE of 6.3% was obtained.172 When carbazole was selected as the electron-donating unit, the resulting PDI–NDI random copolymer had a very inferior electric performance with a PCE of <0.5%.173
Otherwise, there have been reported several non-PDI or non-NDI based copolymer acceptors such as the dithiazolyl-benzothiadiazole based P10 and the DPP-thiazole based P11, which had a PCE of 1.2%
174 and 2.9%,175 respectively, with P3HT and the DPP-terthiophene copolymer as the donor material.
5. Challenges in NF-OSCs
Nowadays, among the four parameters from solution-processed NF-OSCs, the Voc value can be up to 0.8–1.1 V, comparable to those of perovskite solar cells, which are typically about 1 V, but much higher than that of the corresponding fullerene solar cells. The Jsc value can be up to 13 mA cm−2, and even up to 18–19 mA cm−2.46,172 The high Jsc values of 18–19 mA cm−2 have become comparable to those of high-efficiency fullerene organic solar cells, typically 18–20 mA cm−2.5,6,24–37 While the FF value is normally smaller than 60%, some of cells can have a higher FF value, approaching about 65%. It can be seen that the FF value from NF-OSCs is much smaller than the value of the high-efficiency fullerene organic solar cells, typically up to 70–80%. Therefore, improving the FF value is a key issue in designing high-efficiency NF-OSCs that can compete with their fullerene counterparts.
The FF value is relative to the recombination losses, carrier mobility and lifetime, etc. Meanwhile, the Jsc is scaled by the recombination losses and carrier mobility and lifetime, and it is also related to the light absorption ability of the donor–acceptor materials. As we can see from the above parts, the donor-to-acceptor combination and film morphology optimization are both important for high Jsc, FF and even Voc values. From Tables 1–5 and 7, we can see that the electron mobility from most reported donor-to-acceptor systems is normally smaller than the corresponding hole mobility measured from the same blend film. In this context, designing acceptor materials with an improved electron mobility is required. However, the current design concept, for example, for the most efficient PDI and non-PDI large fused ring π-system based small molecule acceptors, is to compromise the crystallinity and the solution-processability, for example, to achieve nanoscale phase domains. The electron-mobility is therefore traded off. Designing new acceptor molecules with an ideal electron mobility is a big challenge to date.
Non-geminate loss of the CT state should be another focus. The donor-to-acceptor arrangement at the interface is an important factor in influencing charge separation. Cvetko, Morgante and coworkers indicated that a donor molecule with shape-complementarity to the spherical PCBM may have an extended interface between the donor and acceptor molecules, leading to faster electron transfer from the donor to the PCBM.65 McGehee, Beaujuge, and coworkers reported that the intermolecular arrangement of the donor and PCBM can be adjusted by the positions of the branched and linear alkyl side chains either on the D or A moiety of the conjugated backbone of the polymer donor: the steric effects from the 2-ethylhexyl side chain on the D unit (with the linear one on the A moiety) hinder interactions of the D unit with the fullerene while the fullerene docks with the A moiety, presenting a higher cell performance.66 Fréchet, Brédas and coworkers showed that introducing the twisted octylphenyl side group instead of a linear alkyl one on the polythiophene backbone decreases the barrier to the charge separation (for example, with the enlargement of the donor–acceptor distance), leading to a higher efficiency.67 Zhan and coworkers demonstrated that the capped diphenylamine can improve the donor-to-acceptor compatibility through the π–π interactions between the diphenylamine group and PC71BM, with respect to the butyl capped alkyl chain, and thus, enhance the charge separation and Ge–hmax value.68 However, effects of the donor-to-acceptor interfacial structures on the charge separation is less known to date. Deeper understanding of these effects is urgently needed and is another big challenge in the development of high-efficiency non-fullerene organic solar cells or even fullerene organic solar cells, in particular, on the basis of ultrafast-absorption-spectrum-data.
6. Conclusions
Since 2013, fast advances have been achieved in NF-OSCs, a sub-branch of organic solar cells. These advances include the judicious design and synthesis of new acceptor molecules, realization of new efficient donor–acceptor combinations, studying the role of the acceptor domain morphology and structural order in the cell performance. Along with such great efforts, the efficiency of NF-OSCs has been raised from 4% up to 8% in a short 3 year period of time. Among these advances, several kinds of small molecule and polymer acceptors have been investigated. The reported small-molecules include those based on perylene diimide, non-perylene diimide large π-fused rings and other conjugated systems from relatively small electron donating and accepting π-aromatics. Of the polymer acceptors, current interest is focused on the perylene diimide based polymer, while other polymers utilizing DPP or benzothiadiazole as the electron-accepting units have also been studied.
Although fast advances have been achieved, the reported efficiency of NF-OSCs as yet lags behinds their fullerene counterparts. One of the major obstacles is the relatively low electron mobility and strong recombination losses. Finding ways towards improving the electron mobility and suppressing recombination losses through the acceptor molecule design, film morphology optimization, and organic–electrode interface engineering, for example, are necessary to realize efficient NF-OSCs. Both low electron mobility and strong recombination loss significantly limit the cell’s FF value. Recently, Neher, Koster and coworkers introduced a dimensionless parameter to quantify the ratio of recombination and extraction rates, showing improvements of charge transportation, while reduction of the charge recombination loss is needed to achieve a high FF value.176
Organic acceptors are much richer in source than fullerene ones. Chemical modifications on organic molecules are more accessible, and hence, the tuning of the absorption, either blue or red shifting, and energy levels, either going to high- or low-lying levels, are more available on organic π-systems than on fullerene systems. This accompanies the achievements in the synthesis of high-efficiency donor molecules. Even high efficiency NF-OSCs can be expected in the near future and NF-OSCs will become competitive with their fullerene counterparts.
Acknowledgements
This work was financially supported by NSFC (No. 21327805, 91227112, 91433202 and 21221002), and Chinese Academy of Sciences (XDB12010200).
Notes and references
- 2013 Renewable Energy Data Book, http://www.nrel.gov/ Search PubMed.
- M. A. Green, K. Emery, Y. Hishikawa, W. Warta and E. D. Dunlop, Solar cell efficiency tables (Version 45), Prog. Photovoltaics, 2015, 23, 1–9 Search PubMed.
- G. Yu, J. Gao, J. C. Hummelen, F. Wudl and A. J. Heeger, Polymer photovoltaic cells: Enhanced efficiencies via a network of internal donor–acceptor heterojunctions, Science, 1995, 270, 1789–1791 CAS.
- C. W. Tang, Two-layer organic photovoltaic cell, Appl. Phys. Lett., 1986, 48, 183–185 CrossRef CAS.
- Y. Liu, J. Zhao, Z. Li, C. Mu, W. Ma, H. Hu, K. Jiang, H. Lin, H. Ade and H. Yan, Aggregation and morphology control enables multiple cases of high-efficiency polymer solar cells, Nat. Commun., 2014, 5, 5293 CrossRef CAS PubMed.
- A. R. B. M. Yusoff, D. Kim, H. P. Kim, F. K. Shneider, W. J. da Silva and J. Jang, High efficiency solution processed polymer inverted triple-junction solar cell exhibiting conversion efficiency of 11.83%, Energy Environ. Sci., 2015, 8, 303–316 CAS.
- J. E. Anthony, Small-Molecule, Nonfullerene Acceptors for Polymer Bulk Heterojunction Organic Photovoltaics, Chem. Mater., 2011, 23, 583–590 CrossRef CAS.
- Y. Shu, Y.-F. Lim, Z. Li, B. Purushothaman, R. Hallani, J. E. Kim, S. R. Parkin, G. G. Malliaras and J. E. Anthony, A survey of electron-deficient pentacenes as acceptors in polymer bulk heterojunction solar cells, Chem. Sci., 2011, 2, 363–368 RSC.
- P. Sonar, J. P. F. Lim and K. L. Chan, Organic non-fullerene acceptors for organic photovoltaics, Energy Environ. Sci., 2011, 4, 1558–1574 CAS.
- E. Kozma and M. Catellani, Perylene diimides based materials for organic solar cells, Dyes Pigm., 2013, 98, 160–179 CrossRef CAS.
- C. L. Chochos, N. Tagmatarchisc and V. S. G. Gregoriou, Rational design on n-type organic materials for high performance organic photovoltaics, RSC Adv., 2013, 3, 7160–7181 RSC.
- A. Facchetti, Polymer donor–polymer acceptor (all-polymer) solar cells, Mater. Today, 2013, 16, 123–132 CrossRef CAS.
- P. Hudhomme, An overview of molecular acceptors for organic solar cells, EPJ Photovoltaics, 2013, 4, 40401 CrossRef CAS.
- A. F. Eftaiha, J.-P. Sun, I. G. Hill and G. C. Welch, Recent advances of non-fullerene, small molecular acceptors for solution processed bulk heterojunction solar cells, J. Mater. Chem. A, 2014, 2, 1201–1213 CAS.
- Y. Kim and E. Lim, Development of Polymer Acceptors for Organic Photovoltaic Cells, Polymers, 2014, 6, 382–407 CrossRef.
- Y. Lin and X. Zhan, Non-fullerene acceptors for organic photovoltaics: an emerging horizon, Mater. Horiz., 2014, 1, 470–488 RSC.
- C. Li and H. Wonneberger, Perylene Imides for Organic Photovoltaics: Yesterday, Today, and Tomorrow, Adv. Mater., 2012, 24, 613–636 CrossRef CAS PubMed.
- M.-E. Ragoussia and T. Torres, New generation solar cells: concepts, trends and perspectives, Chem. Commun., 2015, 51, 3957–3972 RSC.
- C. L. Zhan and A. D. Q. Li, Perylene Diimide: Versatile Building Blocks for Molecular Self-Assemblies, Folding Motifs, and Reaction-Directing Codes, Curr. Org. Chem., 2011, 15, 1314–1339 CrossRef CAS.
- F. Wang, Z. Tan and Y. Li, Solution-processable metal oxides/chelates as electrode buffer layers for efficient and stable polymer solar cells, Energy Environ. Sci., 2015, 8, 1059–1091 CAS.
- S. Braun, W. R. Salaneck and M. Fahlman, Energy-Level Alignment at Organic/Metal and Organic/Organic Interfaces, Adv. Mater., 2009, 21, 1450–1472 CrossRef CAS.
- L. Lu and L. Yu, Understanding Low Bandgap Polymer PTB7 and Optimizing Polymer Solar Cells Based on It, Adv. Mater., 2014, 26, 4413–4430 CrossRef CAS PubMed.
- S.-H. Liao, H.-J. Jhuo, Y.-S. Cheng and S.-A. Chen, Fullerene Derivative-Doped Zinc Oxide Nanofilm as the Cathode of Inverted Polymer Solar Cells with Low-Bandgap Polymer (PTB7-Th) for High Performance, Adv. Mater., 2013, 25, 4766–4771 CrossRef CAS PubMed.
- V. Gupta, A. K. K. Kyaw, D. H. Wang, S. Chand, G. C. Bazan and A. J. Heeger, Barium: An Efficient Cathode Layer for Bulk-heterojunction Solar Cells, Sci. Rep., 2013, 3, 1965 Search PubMed.
- B. Kan, M. Li, Q. Zhang, F. Liu, X. Wan, Y. Wang, W. Ni, G. Long, X. Yang, H. Feng, Y. Zuo, M. Zhang, F. Huang, Y. Cao, T. P. Russell and Y. Chen, A Series of Simple Oligomer-like Small Molecules Based on Oligothiophenes for Solution-Processed Solar Cells with High Efficiency, J. Am. Chem. Soc., 2015, 137, 3886–3893 CrossRef CAS PubMed.
- S. Zhang, L. Ye, W. Zhan, B. Yang, Q. Wang and J. Hou, Realizing Over 10% Efficiency in Polymer Solar Cell by Device Optimization, Sci. China: Chem., 2015, 58, 248–256 CrossRef CAS.
- J.-D. Chen, C. Cui, Y.-Q. Li, L. Zhou, Q.-D. Ou, C. Li, Y. Li and J.-X. Tang, Single-Junction Polymer Solar Cells Exceeding 10% Power Conversion Efficiency, Adv. Mater., 2015, 27, 1035–1041 CrossRef CAS PubMed.
- Z. He, B. Xiao, F. Liu, H. Wu, Y. Yang, S. Xiao, C. Wang, T. P. Russell and Y. Cao, Single-junction polymer solar cells with high efficiency and photovoltage, Nat. Photonics, 2015, 9, 174–179 CrossRef CAS.
- J. Zhang, Y. Zhang, J. Fang, K. Lu, Z. Wang, W. Ma and Z. Wei, Conjugated polymer-small molecule alloy leads to high efficient ternary organic solar cells, J. Am. Chem. Soc., 2015, 137, 8176–8183 CrossRef CAS PubMed.
- W. Yu, L. Huang, D. Yang, P. Fu, L. Zhou, J. Zhang and C. Li, Efficiency exceeding 10% for inverted polymer solar cells with ZnO/ionic liquid combined cathode interfacial layer, J. Mater. Chem. A, 2015, 2015(3), 10660–10665 Search PubMed.
- S. Liu, P. You, J. Li, J. Li, C.-S. Lee, B. S. Ong, C. Surya and F. Yan, Enhanced efficiency of polymer solar cells by adding a high-mobility conjugated polymer, Energy Environ. Sci., 2015, 8, 1463–1470 CAS.
- J. Huang, C.-Z. Li, C.-C. Chueh, S.-Q. Liu, J.-S. Yu and A. K.-Y. Jen, 10.4% Power Conversion Efficiency of ITO-Free Organic Photovoltaics Through Enhanced Light Trapping Configuration, Adv. Energy Mater., 2015, 1500406 Search PubMed.
- L. K. Jagadamma, M. Al-Senani, A. El-Labban, I. Gereige, G. O. N. Ndjawa, J. C. D. Faria, T. Kim, K. Zhao, F. Cruciani, D. H. Anjum, M. A. McLachlan, P. M. Beaujuge and A. Amassian, Polymer Solar Cells with Efficiency >10% Enabled via a Facile Solution-Processed Al-Doped ZnO Electron Transporting Layer, Adv. Energy Mater., 2015, 1500204 Search PubMed.
- V. Vohra, K. Kawashima, T. Kakara, T. Koganezawa, I. Osaka, K. Takimiya and H. Murata, Efficient inverted polymer solar cells employing favourable molecular orientation, Nat. Photonics, 2015, 9, 403–408 CrossRef CAS.
- X. Ouyang, R. Peng, L. Ai, X. Zhang and Z. Ge, Efficient polymer solar cells employing
a non-conjugated small-molecule electrolyte, Nat. Photonics, 2015, 9, 520–524 CrossRef CAS.
- L. Zuo, C.-Y. Chang, C.-C. Chueh, S. Zhang, H. Li, A. K.-Y. Jen and H. Chen, Design of a versatile interconnecting layer for highly efficient series-connected polymer tandem solar cells, Energy Environ. Sci., 2015, 8, 1712–1718 CAS.
- H. Zhou, Y. Zhang, C.-K. Mai, S. D. Collins, G. C. Bazan, T.-Q. Nguyen and A. J. Heeger, Polymer Homo-Tandem Solar Cells with Best Efficiency of 11.3%, Adv. Mater., 2015, 27, 1767–1773 CrossRef CAS PubMed.
- Y. Li, Molecular Design of Photovoltaic Materials for Polymer Solar Cells: Toward Suitable Electronic Energy Levels and Broad Absorption, Acc. Chem. Res., 2012, 45, 723–733 CrossRef CAS PubMed.
- E. Zhou, J. Cong, Q. Wei, K. Tajima, C. Yang and K. Hashimoto, All-Polymer Solar Cells from Perylene Diimide Based Copolymers: Material Design and Phase Separation Control, Angew. Chem., Int. Ed., 2011, 50, 2799–2803 CrossRef CAS PubMed.
- J. T. Bloking, X. Han, A. T. Higgs, J. P. Kastrop, L. Pandey, J. E. Norton, C. Risko, C. E. Chen, J.-L. Brédas, M. D. McGehee and A. Sellinger, Solution-Processed Organic Solar Cells with Power Conversion Efficiencies of 2.5% using Benzothiadiazole/Imide-Based Acceptors, Chem. Mater., 2011, 23, 5484–5490 CrossRef CAS.
- X. Zhang, Z. H. Lu, L. Ye, C. L. Zhan, J. Hou, S. Zhang, B. Jiang, Y. Zhao, J. H. Huang, S. L. Zhang, Y. Liu, Q. Shi, Y. Liu and J. N. Yao, A Potential Perylene Diimide Dimer-Based Acceptor Material for Highly Efficient Solution-Processed Non-Fullerene Organic Solar Cells with 4.03% Efficiency, Adv. Mater., 2013, 25, 5791–5797 CrossRef CAS PubMed.
- R. Shivanna, S. Shoaee, S. Dimitrov, S. K. Kandappa, S. Rajaram, J. R. Durrant and K. S. Narayan, Charge Generation and Transport in Efficient Organic Bulk Heterojunction Solar Cells with a Perylene Acceptor, Energy Environ. Sci., 2014, 7, 435–441 CAS.
- Q. F. Yan, Y. Zhou, Y. Q. Zheng, J. Pei and D. H. Zhao, Towards Rational Design of Organic Electron Acceptors for Photovoltaics: a Study Based on Perylenediimide Derivatives, Chem. Sci., 2013, 4, 4389–4394 RSC.
- B. Jiang, X. Zhang, C. L. Zhan, Z. H. Lu, J. H. Huang, X. L. Ding, S. G. He and J. N. Yao, Benzodithiophene Bridged Dimeric Perylene Diimide Amphiphile as Efficient Solution-Processed Non-Fullerene Small Molecule, Polym. Chem., 2013, 4, 4631–4638 RSC.
- Z. H. Lu, X. Zhang, C. L. Zhan, B. Jiang, X. L. Zhang, L. L. Chen and J. N. Yao, Impact of Molecular Solvophobicity vs. Solvophilicity on Device Performances of Dimeric Perylene Diimide Based Solution-Processed Non-Fullerene Organic Solar Cells, Phys. Chem. Chem. Phys., 2013, 15, 11375–11385 RSC.
- Y.-J. Hwang, B. A. E. Courtright, A. S. Ferreira, S. H. Tolbert and S. A. Jenekhe, 7.7% Efficient All-Polymer Solar Cells, Adv. Mater., 2015, 27, 4578–4584 CrossRef CAS PubMed.
- J. H. Huang, X. Wang, X. Zhang, Z. X. Niu, Z. H. Lu, B. Jiang, Y. X. Sun, C. L. Zhan and J. N. Yao, Additive-Assisted Control over Phase-Separated Nanostructures by Manipulating Alkylthienyl Position at Donor Backbone for Solution-Processed Non-Fullerene All-Small-Molecule Solar Cells, ACS Appl. Mater. Interfaces, 2014, 6, 3853–3862 CAS.
- Y. He, H. Chen, J. Hou and Y. Li, Indene–C60 Bisadduct: A New Acceptor for High-Performance Polymer Solar Cells, J. Am. Chem. Soc., 2010, 132, 1377–1382 CrossRef CAS PubMed.
- A. Anctil, C. W. Babbitt, R. P. Raffaelle and B. J. Landi, Material and Energy Intensity of Fullerene Production, Environ. Sci. Technol., 2011, 45, 2353–2359 CrossRef CAS PubMed.
- X. Zhang, C. L. Zhan and J. N. Yao, Non-Fullerene Organic Solar Cells with 6.1% Efficiency through Fine-Tuning Parameters of Film-Forming Process, Chem. Mater., 2015, 27(1), 166–173 CrossRef CAS.
- H. K. H. Lee, Z. Li, I. Constantinou, F. So, S. W. Tsang and S. K. So, Batch-to-Batch Variation of Polymeric Photovoltaic Materials: its Origin and Impacts on Charge Carrier Transport and Device Performances, Adv. Energy Mater., 2014, 1400768 Search PubMed.
- X. Zhan, Z. Tan, B. Domercq, Z. An, X. Zhang, S. Barlow, Y. Li, D. Zhu, B. Kippelen and S. R. Marder, A High-Mobility Electron-Transport Polymer with Broad Absorption and Its Use in Field-Effect Transistors and All-Polymer Solar Cells, J. Am. Chem. Soc., 2007, 129, 7246–7247 CrossRef CAS PubMed.
- Y. X. Chen, A. L. Tang, X. Zhang, Z. L. Lu, J. H. Huang, C. L. Zhan and J. N. Yao, A New Solution-Processed Diketopyrrolopyrrole Donor for Non-Fullerene Small-Molecule Solar Cells, J. Mater. Chem. A, 2014, 2, 1869–1876 CAS.
- K. Vandewal, A. Gadisa, W. D. Oosterbaan, S. Bertho, F. Banishoeib, I. V. Severen, L. Lutsen, T. J. Cleij, D. Vanderzande and J. V. Manca, The Relation Between Open-Circuit Voltage and the Onset of Photocurrent Generation by Charge-Transfer Absorption in Polymer:Fullerene Bulk Heterojunction Solar Cells, Adv. Funct. Mater., 2008, 18, 2064–2070 CrossRef CAS.
- S. Gelinas, A. Rao, A. Kumar, S. L. Smith, A. W. Chin, J. Clark, T. S. van der Poll, G. C. Bazan and R. H. Friend, Ultrafast Long-Range Charge Separation in Organic Semiconductor Photovoltaic Diodes, Science, 2014, 343, 512–516 CrossRef CAS PubMed.
- V. D. Mihailetchi, L. J. A. Koster, P. W. M. Blom, C. Melzer, B. de Boer, J. K. J. van Duren and R. A. J. Janssen, Compositional Dependence of the Performance of Poly(p-phenylene vinylene):Methanofullerene Bulk-Heterojunction Solar Cells, Adv. Funct. Mater., 2005, 15, 795–801 CrossRef CAS.
- S. R. Cowan, R. A. Street, S. Cho and A. J. Heeger, Transient Photoconductivity in Polymer Bulk Heterojunction Solar Cells: Competition between Sweep-Out and Recombination, Phys. Rev. B: Condens. Matter Mater. Phys., 2011, 83, 035205 CrossRef.
- A. Sharenko, D. Gehrig, F. Laquai and T.-Q. Nguyen, The Effect of Solvent Additive on the Charge Generation and Photovoltaic Performance of a Solution-Processed Small Molecule:Perylene Diimide Bulk Heterojunction Solar Cell, Chem. Mater., 2014, 26, 4109–4118 CrossRef CAS.
- I. Riedel, J. Parisi, V. Dyakonov, L. Lutsen, D. Vanderzande and J. C. Hummelen, Effect of Temperature and Illumination on the Electrical Characteristics of Polymer–Fullerene Bulk-Heterojunction Solar Cells, Adv. Funct. Mater., 2004, 14, 38–44 CrossRef CAS.
- C. Shuttle, B. O’Regan, A. Ballantyne, J. Nelson, D. Bradley and J. Durrant, Phys. Rev. B: Condens. Matter Mater. Phys., 2008, 78, 113201 CrossRef.
- K. Vandewal, K. Tvingstedt, A. Gadisa, O. Inganäs and J. V. Manca, On the origin of the open-circuit voltage of polymer–fullerene solar cells, Nat. Mater., 2009, 8, 904–909 CrossRef CAS PubMed.
- M. D. Perez, C. Borek, S. R. Forrest and M. E. Thompson, Molecular and Morphological Influences on the Open Circuit Voltages of Organic Photovoltaic Devices, J. Am. Chem. Soc., 2009, 131, 9281–9286 CrossRef CAS PubMed.
- Z. He, B. Xiao, F. Liu, H. Wu, Y. Yang, S. Xiao, C. Wang, T. P. Russell and Y. Cao, Single-junction polymer solar cells with high efficiency and photovoltage, Nat. Photonics, 2015, 9, 174–179 CrossRef CAS.
- T. Heumueller, T. M. Burke, W. R. Mateker, I. T. Sachs-Quintana, K. Vandewal, C. J. Brabec and M. D. McGehee, Disorder-Induced Open-Circuit Voltage Losses in Organic Solar Cells During Photoinduced Burn-In, Adv. Energy Mater., 2015, 1500111 Search PubMed.
- T. Schiros, G. Kladnik, D. Prezzi, A. Ferretti, G. Olivieri, A. Cossaro, L. Floreano, A. Verdini, C. Schenck, M. Cox, A. A. Gorodetsky, K. Plunkett, D. Delongchamp, C. Nuckolls, A. Morgante, D. Cvetko and I. Kymissis, Donor–Acceptor Shape Matching Drives Performance in Photovoltaics, Adv. Energy Mater., 2013, 3, 894 CrossRef CAS.
- K. R. Graham, C. Cabanetos, J. P. Jahnke, M. N. Idso, A. El Labban, G. O. Ngongang Ndjawa, T. Heumueller, K. Vandewal, A. Salleo, B. F. Chmelka, A. Amassian, P. M. Beaujuge and M. D. McGehee, Importance of the Donor: Fullerene Intermolecular Arrangement for High-Efficiency Organic Photovoltaics, J. Am. Chem. Soc., 2014, 136, 9608–9618 CrossRef CAS PubMed.
- T. W. Holcombe, J. E. Norton, J. Rivnay, C. H. Woo, L. Goris, C. Piliego, G. Griffini, A. Sellinger, J. L. Bredas, A. Salleo and J. M. Frechet, Steric Control of the Donor/Acceptor Interface: Implications in Organic Photovoltaic Charge Generation, J. Am. Chem. Soc., 2011, 133, 12106–12114 CrossRef CAS PubMed.
- A. L. Tang, C. L. Zhan and J. N. Yao, Comparative Study of Effects of Terminal Non-Alkyl Aromatic and Alkyl Groups on Small-Molecule Solar Cell Performance, Adv. Energy Mater., 2015, 5, 1500059 Search PubMed.
- Y. Zhao, Y. Guo and Y. Liu, 25th Anniversary Article: Recent Advances in n-Type and Ambipolar Organic Field-Effect Transistors, Adv. Mater., 2013, 25, 5372–5391 CrossRef CAS PubMed.
- F. Würthner, Perylene bisimide dyes as versatile building blocks for functional supramolecular architectures, Chem. Commun., 2004, 1564–1579 RSC.
- D. M. Ke, C. L. Zhan, S. P. Xu, X. L. Ding, A. D. Peng, J. Sun, S. G. He, A. D. Q. Li and J. N. Yao, Self-Assembled Hollow Nanospheres Strongly Enhance Photoluminescence, J. Am. Chem. Soc., 2011, 133, 11022–11025 CrossRef CAS PubMed.
- Z. G. Zhang, C. L. Zhang, X. Zhang, S. L. Zhang, J. H. Huang, A. D. Q. Li and J. N. Yao, A Self-Assembling Phase Diagram from Amphiphilic Perylene Diimides, Chem.–Eur. J., 2012, 18, 12305–12313 CrossRef CAS PubMed.
- J. J. Dittmer, E. A. Marseglia and R. H. Friend, Electron Trapping in Dye/Polymer Blend Photovoltaic Cells, Adv. Mater., 2000, 12, 1270–1274 CrossRef CAS.
- A. Sharenko, C. M. Proctor, T. S. van der Poll, Z. B. Henson, T.-Q. Nguyen and G. C. Bazan, A High-Performing Solution-Processed Small Molecule:Perylene Diimide Bulk Heterojunction Solar Cell, Adv. Mater., 2013, 25, 4403–4407 CrossRef CAS PubMed.
- Y. X. Chen, X. Zhang, C. L. Zhan and J. N. Yao, In-Depth Understanding of Photocurrent Enhancement in Solution-Processed Small-Molecule:Perylene-Diimide Non-fullerene Organic Solar Cells, Phys. Status Solidi A, 2015, 212, 1961–1968 CrossRef CAS.
- L. J. Huo, S. Q. Zhang, X. Guo, F. Xu, Y. F. Li and J. H. Hou, Replacing Alkoxy Groups with Alkylthienyl Groups: A Feasible Approach To Improve the Properties of Photovoltaic Polymers, Angew. Chem., Int. Ed., 2011, 50, 9697–9702 CrossRef CAS PubMed.
- R. Singh, E. Aluicio-Sarduy, Z. Kan, T. Ye, R. C. I. MacKenzie and P. E. Keivanidis, Fullerene-free organic solar cells with an efficiency of 3.7% based on a low-cost geometrically planar perylene diimide monomer, J. Mater. Chem. A, 2014, 2, 14348–14353 CAS.
- T. Ye, R. Singh, H.-J. Butt, G. Floudas and P. E. Keivanidis, Effect of Local and Global Structural Order on the Performance of Perylene Diimide Excimeric Solar Cells, ACS Appl. Mater. Interfaces, 2013, 5, 11844–11857 CAS.
- E. Aluicio-Sarduy, R. Singh, Z. Kan, T. Ye, A. Baidak, A. Calloni, G. Berti, L. Duò, A. Iosifidis, S. Beaupré, M. Leclerc, H.-J. Butt, G. Floudas and P. E. Keivanidis, Elucidating the Impact of Molecular Packing and Device Architecture on the Performance of Nanostructured Perylene Diimide Solar Cells, ACS Appl. Mater. Interfaces, 2015, 7, 8687–8698 CAS.
- D. W. Gehrig, S. Roland, I. A. Howard, V. Kamm, H. Mangold, D. Neher and F. Laquai, Efficiency-Limiting Processes in Low-Bandgap Polymer: Perylene Diimide Photovoltaic Blends, J. Phys. Chem. C, 2014, 118, 20077–20085 CAS.
- S. Shoaee, F. Deledalle, P. S. Tuladhar, R. Shivanna, S. Rajaram, K. S. Narayan and J. R. Durrant, A Comparison of Charge Separation Dynamics in Organic Blend Films Employing Fullerene and Perylene Diimide Electron Acceptors, J. Phys. Chem. Lett., 2015, 6, 201–205 CrossRef CAS PubMed.
- X. L. Zhang, B. Jiang, X. Zhang, J. H. Huang, C. L. Zhan and J. N. Yao, Cooperatively Tuning Phase Size and Absorption of Near IR Photons in P3HT: Perylene Diimide Solar Cells by Bay-Modifications on the Acceptor, J. Phys. Chem. C, 2014, 118, 24212–24220 CAS.
- Y. Cai, L. Huo, X. Sun, D. Wei, M. Tang and Y. Sun, High Performance Organic Solar Cells Based on a Twisted Bay-Substituted Tetraphenyl Functionalized Perylenediimide Electron Acceptor, Adv. Energy Mater., 2015, 1500032 Search PubMed.
- P. E. Hartnett, A. Timalsina, H. S. S. R. Matte, N. Zhou, X. Guo, W. Zhao, A. Facchetti, R. P. H. Chang, M. C. Hersam, M. R. Wasielewski and T. J. Marks, Slip-Stacked Perylenediimides as an Alternative Strategy for High Efficiency Nonfullerene Acceptors in Organic Photovoltaics, J. Am. Chem. Soc., 2014, 136, 16345–16356 CrossRef CAS PubMed.
- S. Rajaram, R. Shivanna, S. K. Kandappa and K. S. Narayan, Nonplanar Perylene Diimides as Potential Alternatives to Fullerenes in Organic Solar Cells, J. Phys. Chem. Lett., 2012, 3, 2405–2408 CrossRef CAS PubMed.
- L. Ye, K. Sun, W. Jiang, S. Zhang, W. Zhao, H. Yao, Z. Wang and J. Hou, Enhanced Efficiency in Fullerene-Free Polymer Solar Cell by Incorporating Fine-designed Donor and Acceptor Materials, ACS Appl. Mater. Interfaces, 2015, 7(17), 9274–9280 CAS.
- C.-H. Wu, C.-C. Chueh, Y.-Y. Xi, H.-L. Zhong, G.-P. Gao, Z.-H. Wang, L. D. Pozzo, T.-C. Wen and A. K.-Y. Jen, Influence of Molecular Geometry of Perylene Diimide Dimers and Polymers on Bulk Heterojunction Morphology Toward High-Performance Nonfullerene Polymer Solar Cells, Adv. Funct. Mater., 2015, 25, 5326–5332 CrossRef CAS.
- X. Zhang, J. N. Yao and C. L. Zhan, Synthesis and Photovoltaic Properties of Low Bandgap Dimeric Perylene Diimide Based Non-Fullerene Acceptors, Sci. China: Chem., 2015 DOI:SCC-2015-0241.
- X. Zhang, J. N. Yao and C. L. Zhan, Selenophenyl Bridged Perylene Diimide Dimer as Efficient Solution-Processable Small Molecule Acceptor, Chem. Commun., 2015, 51, 1058–1061 RSC.
- Y. X. Chen, X. Zhang, C. L. Zhang and J. N. Yao, Origin of Effects of Additive Solvent on Film-Morphology in Solution-ProcessedNon-Fullerene Solar Cells, ACS Appl. Mater. Interfaces, 2015, 7, 6462–6471 CAS.
- X. Zhang, C. L. Zhan and J. N. Yao, Non-Fullerene Organic Solar Cells with 6.1% Efficiency through Fine-Tuning Parameters of Film-Forming Process, Chem. Mater., 2015, 27, 166–173 CrossRef CAS.
- Z. H. Lu, B. Jiang, X. Zhang, A. L. Tang, L. L. Chen, C. L. Zhan and J. N. Yao, Perylene-Diimide Based Non-Fullerene Solar Cells with 4.34% Efficiency through Engineering Surface D/A Compositions, Chem. Mater., 2014, 26, 2907–2914 CrossRef CAS.
- Y. Lin, J. Wang, S. Dai, Y. Li, D. Zhu and X. Zhan, A Twisted Dimeric Perylene Diimide Electron Acceptor for Efficient Organic Solar Cells, Adv. Energy Mater., 2014, 4, 1400420 Search PubMed.
- J. Wang, Y. Yao, S. Dai, X. Zhang, W. Wang, Q. He, L. Han, Y. Lin and X. Zhan, Oligothiophene-bridged perylene diimide dimers for fullerene-free polymer solar cells: effect of bridge length, J. Mater. Chem. A, 2015, 3, 13000–13010 Search PubMed.
- J. Zhao, Y. Li, H. Lin, Y. Liu, K. Jiang, C. Mu, T. Ma, J. Y. L. Lai, H. Hu, D. Yu and H. Yan, High-efficiency non-fullerene organic solar cells enabled by a difluorobenzothiadiazole-based donor polymer combined with a properly matched small molecule acceptor, Energy Environ. Sci., 2015, 8, 520–525 CAS.
- W. Jiang, L. Ye, X. Li, X. Ciao, F. Tan, W. Zhao, J. Hou and Z. Wang, Bay-linked Perylene Bisimides as Promising Non-Fullerene Acceptors for Organic Solar Cells, Chem. Commun., 2014, 50, 1024–1026 RSC.
- L. Ye, W. Jiang, W. Zhao, S. Zhang, D. Qian, Z. Wang and J. Hou, Selecting a Donor Polymer for Realizing Favorable Morphology in Efficient Non-fullerene Acceptor-based Solar Cells, Small, 2014, 10, 4658–4663 CrossRef CAS PubMed.
- L. Ye, W. Jiang, W. Zhao, S. Zhang, Y. Cui, Z. Wang and J. Hou, Toward efficient non-fullerene polymer solar cells: Selection of donor polymers, Org. Electron., 2015, 17, 295–303 CrossRef CAS.
- Y. Fu, Q. Yang, Y. Deng, W. Jiang, Z. Wang, Y. Geng and Z. Xie, Suppressed charge recombination in polymer solar cells based on perylene diimide derivative acceptors via solvent vapor annealing, Org. Electron., 2015, 18, 24–31 CrossRef CAS.
- Y. Zang, C.-Z. Li, C.-C. Chueh, S. T. Williams, W. Jiang, Z.-H. Wang, J.-S. Yu and A. K.-Y. Jen, Integrated Molecular, Interfacial, and Device Engineering towards High-Performance Non-Fullerene Based Organic Solar Cells, Adv. Mater., 2014, 26, 5708–5714 CrossRef CAS PubMed.
- D. Sun, D. Meng, Y. Cai, B. Fan, Y. Li, W. Jiang, L. Huo, Y. Sun and Z. Wang, Non-Fullerene-Acceptor-Based Bulk-Heterojunction Organic Solar Cells with Efficiency over 7%, J. Am. Chem. Soc., 2015, 137, 11156–11162 CrossRef CAS PubMed.
- Y. Zhong, M. T. Trinh, R. Chen, W. Wang, P. P. Khlyabich, B. Kumar, Q. Xu, C.-Y. Nam, M. Y. Sfeir, C. Black, M. L. Steigerwald, Y.-L. Loo, S. Xiao, F. Ng, X.-Y. Zhu and C. Nuckolls, Efficient Organic Solar Cells with Helical Perylene Diimide Electron Acceptors, J. Am. Chem. Soc., 2014, 136, 15215–15221 CrossRef CAS PubMed.
- B. A. Gregg, Entropy of Charge Separation in Organic Photovoltaic Cells: The Benefit of Higher Dimensionality, J. Phys. Chem. Lett., 2011, 2, 3013–3015 CrossRef CAS.
- X. Zhang, B. Jiang, S. Zhang, J. Hou, J. N. Yao and C. L. Zhan, Solution-processible perylene diimide based star-shaped small molecule acceptor: Synthesis and photovoltaic properties, Proc. SPIE, Organic Photovoltaics XV, 2014, vol. 9184, p. 91840C Search PubMed.
- Y. Lin, Y. Wang, J. Wang, J. Hou, Y. Li, D. Zhu and X. Zhan, A Star-Shaped Perylene Diimide Electron Acceptor for High-Performance Organic Solar Cells, Adv. Mater., 2014, 26, 5137–5142 CrossRef CAS PubMed.
- Y. Liu, C. Mu, K. Jiang, J. Zhao, Y. Li, L. Zhang, Z. Li, J. Y. L. Lai, H. Hu, T. Ma, R. Hu, D. Yu, X. Huang, B. Z. Tang and H. Yan, A Tetraphenylethylene Core-Based 3D Structure Small Molecular Acceptor Enabling Efficient Non-Fullerene Organic Solar Cells, Adv. Mater., 2015, 27, 1015–1020 CrossRef CAS PubMed.
- Y. Liu, J. Y. L. Lai, S. Chen, Y. Li, K. Jiang, J. Zhao, Z. Li, H. Hu, T. Ma, H. Lin, J. Liu, J. Zhang, F. Huang, D. Yu and H. Yan, Efficient non-fullerene polymer solar cells enabled by tetrahedron-shaped core based 3D-structure small-molecular electron acceptors, J. Mater. Chem. A, 2015, 3, 13632–13636 CAS.
- W. Chen, X. Yang, G. Long, X. Wan, Y. Chen and Q. Zhang, A perylene diimide (PDI)-based small molecule with tetrahedral configuration as a non-fullerene acceptor for organic solar cells, J. Mater. Chem. C, 2015, 3, 4698–4705 RSC.
- C. B. Nielsen, E. Voroshazi, S. Holliday, K. Cnops, D. Cheyns and I. McCulloch, Electron-deficient truxenone derivatives and their use in organic photovoltaics, J. Mater. Chem. A, 2014, 2, 12348–12354 CAS.
- T. Zhou, T. Jia, B. Kang, F. Li, M. Fahlman and Y. Wang, Nitrile-Substituted QA Derivatives: New Acceptor Materials for Solution-Processable Organic Bulk Heterojunction Solar Cells, Adv. Energy Mater., 2011, 1, 431–439 CrossRef CAS.
- Y. Zhou, L. Ding, K. Shi, Y.-Z. Dai, N. Ai, J. Wang and J. Pei, A Non-Fullerene Small Molecule as Efficient Electron Acceptor in Organic Bulk Heterojunction Solar Cells, Adv. Mater., 2012, 24, 957–961 CrossRef CAS PubMed.
- Y. Zhou, Y.-Z. Dai, Y.-Q. Zheng, X.-Y. Wang, J.-Y. Wang and J. Pei, Non-fullerene acceptors containing fluoranthene-fused imides for solution-processed inverted organic solar cells, Chem. Commun., 2013, 49, 5802–5804 RSC.
- H. Li, T. Earmme, S. Subramaniyan and S. A. Jenekhe, Bis(Naphthalene Imide)diphenylanthrazolines: A New Class of Electron Acceptors for Efficient Nonfullerene Organic Solar Cells and Applicable to Multiple Donor Polymers, Adv. Energy Mater., 2015, 1402041 Search PubMed.
- H. Li, T. Earmme, G. Ren, A. Saeki, S. Yoshikawa, N. M. Murari, S. Subramaniyan, M. J. Crane, S. Seki and S. A. Jenekhe, Beyond Fullerenes: Design of Nonfullerene Acceptors for Efficient Organic Photovoltaics, J. Am. Chem. Soc., 2014, 136, 14589–14597 CrossRef CAS PubMed.
- H. Li, Y.-J. Hwang, B. A. E. Courtright, F. N. Eberle, S. Subramaniyan and S. A. Jenekhe, Fine-Tuning the 3D Structure of Nonfullerene Electron Acceptors Toward High-Performance Polymer Solar Cells, Adv. Mater., 2015, 27, 3266–3272 CrossRef CAS PubMed.
- C.-P. Chen, S.-H. Chan, T.-C. Chao, C. Ting and B.-T. Ko, Low-Bandgap Poly(Thiophene-Phenylene-Thiophene) Derivatives with Broaden Absorption Spectra for Use in High-Performance Bulk-Heterojunction Polymer Solar Cells, J. Am. Chem. Soc., 2008, 130, 12828–12833 CrossRef CAS PubMed.
- Y.-X. Xu, C.-C. Chueh, H.-L. Yip, F.-Z. Ding, Y.-X. Li, C.-Z. Li, X. Li, W.-C. Chen and A. K. Y. Jen, Improved Charge Transport and Absorption Coefficient in Indacenodithieno[3,2-b]thiophene-based Ladder-Type Polymer Leading to Highly Efficient Polymer Solar Cells, Adv. Mater., 2012, 24, 6356–6361 CrossRef CAS PubMed.
- Z.-G. Zhang, B. Qi, Z. Jin, D. Chi, Z. Qi, Y. Li and J. Wang, Perylene diimides: a thickness-insensitive cathode interlayer for high performance polymer solar cells, Energy Environ. Sci., 2014, 7, 1966–1973 CAS.
- Y. Lin, Z.-G. Zhang, H. Bai, J. Wang, Y. Yao, Y. Li, D. Zhu and X. Zhan, High-performance fullerene-free polymer solar cells with 6.31% efficiency, Energy Environ. Sci., 2015, 8, 610–616 CAS.
- Y. Lin, J. Wang, Z.-G. Zhang, H. Bai, Y. Li, D. Zhu and X. Zhan, An Electron Acceptor Challenging Fullerenes for Efficient Polymer Solar Cells, Adv. Mater., 2015, 27, 1170–1174 CrossRef CAS PubMed.
- T. V. Pho, F. M. Toma, M. L. Chabinyc and F. Wudl, Self-Assembling Decacyclene Triimides Prepared through a Regioselective Hextuple Friedel–Crafts Carbamylation, Angew. Chem., Int. Ed., 2013, 52, 1446–1451 CrossRef CAS PubMed.
- Z. Mao, W. Senevirathna, J.-Y. Liao, J. Gu, S. V. Kesava, C. Guo, E. D. Gomez and G. Sauvé, Azadipyrromethene-Based Zn(II) Complexes as Nonplanar Conjugated Electron Acceptors for Organic Photovoltaics, Adv. Mater., 2014, 26, 6290–6294 CrossRef CAS PubMed.
- F. G. Brunetti, X. Gong, M. Tong, A. J. Heeger and F. Wudl, Strain and Hückel Aromaticity: Driving Forces for a Promising New Generation of Electron Acceptors in Organic Electronics, Angew. Chem., Int. Ed., 2010, 49, 532–536 CrossRef CAS PubMed.
- Z. Mao, T. P. Le, K. Vakhshouri, R. Fernando, F. Ruan, E. Muller, E. D. Gomez and G. Sauvé, Processing additive suppresses phase separation in the active layer of organic photovoltaics based on naphthalene diimide, Org. Electron., 2014, 15, 3384–3391 CrossRef CAS.
- E. Ahmed, G. Ren, F. S. Kim, E. C. Hollenbeck and S. A. Jenekhe, Design of New Electron Acceptor Materials for Organic Photovoltaics: Synthesis, Electron Transport, Photophysics, and Photovoltaic Properties of Oligothiophene-Functionalized Naphthalene Diimides, Chem. Mater., 2011, 23, 4563–4577 CrossRef CAS.
- X. Wang, J. H. Huang, Z. X. Niu, Y. X. Sun and C. L. Zhan, Dimeric Naphthalene Diimide Based Small Molecule Acceptors: Synthesis, Characterization, and Photovoltaic Properties, Tetrahedron, 2014, 70, 4726–4731 CrossRef CAS.
- Y. Liu, L. Zhang, H. Lee, H.-W. Wang, A. Santala, F. Liu, Y. Diao, A. L. Briseno and T. P. Russell, NDI-Based Small Molecule as Promising Nonfullerene Acceptor for Solution-Processed Organic Photovoltaics, Adv. Energy Mater., 2015, 1500195 Search PubMed.
- C. H. Woo, T. W. Holcombe, D. A. Unruh, A. Sellinger and J. M. J. Fréchet, Phenyl vs. Alkyl Polythiophene: A Solar Cell Comparison Using a Vinazene Derivative as Acceptor, Chem. Mater., 2010, 22, 1673–1679 CrossRef CAS.
- B. Walker, X. Han, C. Kim, A. Sellinger and T.-Q. Nguyen, Solution-Processed Organic Solar Cells from Dye Molecules: An Investigation of Diketopyrrolopyrrole:Vinazene Heterojunctions, ACS Appl. Mater. Interfaces, 2012, 4, 244–250 CAS.
- J. T. Bloking, X. Han, A. T. Higgs, J. P. Kastrop, L. Pandey, J. E. Norton, C. Risko, C. E. Chen, J.-L. Brédas, M. D. McGehee and A. Sellinger, Solution-Processed Organic Solar Cells with Power Conversion Efficiencies of 2.5% using Benzothiadiazole/Imide-Based Acceptors, Chem. Mater., 2011, 23, 5484–5490 CrossRef CAS.
- J. T. Bloking, T. Giovenzana, A. T. Higgs, A. J. Ponec, E. T. Hoke, K. Vandewal, S. Ko, Z. Bao, A. Sellinger and M. D. McGehee, Comparing the Device Physics and Morphology of Polymer Solar Cells Employing Fullerenes and Non-Fullerene Acceptors, Adv. Energy Mater., 2014, 4, 1301426 Search PubMed.
- J. D. Douglas, M. S. Chen, J. R. Niskala, O. P. Lee, A. T. Yiu, E. P. Young and J. M. J. Fréchet, Solution-Processed, Molecular Photovoltaics that Exploit Hole Transfer from Non-Fullerene, n-Type Materials, Adv. Mater., 2014, 26, 4313–4319 CrossRef CAS PubMed.
- K. N. Winzenberg, P. Kemppinen, F. H. Scholes, G. E. Collis, Y. Shu, T. B. Singh, A. Bilic, C. M. Forsyth and S. E. Watkins, Indan-1,3-dione electron-acceptor small molecules for solution-processable solar cells: a structure–property correlation, Chem. Commun., 2013, 49, 6307–6309 RSC.
- Y. Kim, C. E. Song, S.-J. Moonc and E. Lim, Rhodanine dye-based small molecule acceptors for organic photovoltaic cells, Chem. Commun., 2014, 50, 8235–8238 RSC.
- S. Holliday, R. S. Ashraf, C. B. Nielsen, M. Kirkus, J. A. Röhr, C.-H. Tan, E. Collado-Fregoso, A.-C. Knall, J. R. Durrant, J. Nelson and I. McCulloch, A Rhodanine Flanked Nonfullerene Acceptor for Solution-Processed Organic Photovoltaics, J. Am. Chem. Soc., 2015, 137, 898–904 CrossRef CAS PubMed.
- O. K. Kwon, J.-H. Park, S. K. Park and S. Y. Park, Soluble Dicyanodistyrylbenzene-Based Non-Fullerene Electron Acceptors with Optimized Aggregation Behavior for High-Efficiency Organic Solar Cells, Adv. Energy Mater., 2014, 1400929 Search PubMed.
- O. K. Kwon, J.-H. Park, D. W. Kim, S. K. Park and S. Y. Park, An All-Small-Molecule Organic Solar Cell with High Efficiency Nonfullerene Acceptor, Adv. Mater., 2015, 27, 1951–1956 CrossRef CAS PubMed.
- R.-Q. Lu, Y.-Q. G. Zheng, Y.-N. Zhou, X.-Y. Yan, T. Lei, K. Shi, Y. Zhou, J. Pei, L. Zoppi, K. K. Baldridge, J. S. Siegeld and X.-Y. Cao, Corannulene derivatives as non-fullerene acceptors in solution-processed bulk heterojunction solar cells, J. Mater. Chem. A, 2014, 2, 20515–20519 CAS.
- C. G. Claessens, D. González-Rodríguez and T. Torres, Subphthalocyanines: Singular Nonplanar Aromatic CompoundssSynthesis, Reactivity, and Physical Properties, Chem. Rev., 2002, 102, 835–853 CrossRef CAS PubMed.
- B. Verreet, B. P. Rand, D. Cheyns, A. Hadipour, T. Aernouts, P. Heremans, A. Medina, C. G. Claessens and T. Torres, A 4% Efficient Organic Solar Cell Using a Fluorinated Fused Subphthalocyanine Dimer as an Electron Acceptor, Adv. Energy Mater., 2011, 1, 565–568 CrossRef CAS.
- G. E. Morse, J. L. Gantz, K. X. Steirer, N. R. Armstrong and T. P. Bender, Pentafluorophenoxy Boron Subphthalocyanine (F5BsubPc) as a Multifunctional Material for Organic Photovoltaics, ACS Appl. Mater. Interfaces, 2014, 6, 1515–1524 CAS.
- B. Verreet, K. Cnops, D. Cheyns, P. Heremans, A. Stesmans, G. Zango, C. G. Claessens, T. Torres and B. P. Rand, Decreased Recombination Through the Use of a Non-Fullerene Acceptor in a 6.4% Efficient Organic Planar Heterojunction Solar Cell, Adv. Energy Mater., 2014, 4, 1301413 Search PubMed.
- K. Cnops, B. P. Rand, D. Cheyns, B. Verreet, M. A. Empl and P. Heremans, 8.4%
efficient fullerene-free organic solar cells exploiting long-range exciton energy transfer, Nat. Commun., 2014, 5, 3406 Search PubMed.
- P. Sullivan, G. E. Collis, L. A. Rochford, J. F. Arantes, P. Kemppinen, T. S. Jones and K. N. Winzenberg, An N-ethylated barbituric acid end-capped bithiophene as an electron-acceptor material in fullerene-free organic photovoltaics, Chem. Commun., 2015, 51, 6222–6225 RSC.
- A. N. Bartynski, M. Gruber, S. Das, S. Rangan, S. Mollinger, C. Trinh, S. E. Bradforth, K. Vandewal, A. Salleo, R. A. Bartynski, W. Bruetting and M. E. Thompson, Symmetry-Breaking Charge Transfer in a Zinc Chlorodipyrrin Acceptor for High Open Circuit Voltage Organic Photovoltaics, J. Am. Chem. Soc., 2015, 137, 5397–5405 CrossRef CAS PubMed.
- C. B. Nielsen, E. Voroshazi, S. Holliday, K. Cnops, D. Cheyns and I. McCulloch, Electron-deficient truxenone derivatives and their use in organic photovoltaics, J. Mater. Chem. A, 2014, 2, 12348–12354 CAS.
- Y. Zhou, T. Kurosawa, W. Ma, Y. Guo, L. Fang, K. Vandewal, Y. Diao, C. Wang, Q. Yan, J. Reinspach, J. Mei, A. L. Appleton, G. I. Koleilat, Y. Gao, S. C. B. Mannsfeld, A. Salleo, H. Ade, D. Zhao and Z. Bao, High Performance All-Polymer Solar Cell via Polymer Side-Chain Engineering, Adv. Mater., 2014, 26, 3767–3772 CrossRef CAS PubMed.
- Y. Zhou, Q. Yan, Y.-Q. Zheng, J.-Y. Wang, D. Zhao and J. Pei, New polymer acceptors for organic solar cells: the effect of regio-regularity and device configuration, J. Mater. Chem. A, 2013, 1, 6609–6613 CAS.
- P. Cheng, L. Ye, X. Zhao, J. Hou, Y. Li and X. Zhan, Binary additives synergistically boost the efficiency of all-polymer solar cells up to 3.45%, Energy Environ. Sci., 2014, 7, 1351–1356 CAS.
- J. Cao, Q. Liao, X. Du, J. Chen, Z. Xiao, Q. Zuo and L. Ding, A pentacyclic aromatic lactam building block for efficient polymer solar cells, Energy Environ. Sci., 2013, 6, 3224–3228 CAS.
- I. H. Jung, W.-Y. Lo, J. Jang, W. Chen, D. Zhao, E. S. Landry, L. Lu, D. V. Talapin and L. Yu, Synthesis and Search for Design Principles of New Electron Accepting Polymers for All-Polymer Solar Cells, Chem. Mater., 2014, 26, 3450–3459 CrossRef CAS.
- I. H. Jung, D. Zhao, J. Jang, W. Chen, E. S. Landry, L. Lu, D. V. Talapin and L. Yu, Development and Structure/Property Relationship of New Electron Accepting Polymers Based on Thieno[2′,3′:4,5]pyrido[2,3-g]thieno[3,2-c]quinoline-4,10-dione for All-Polymer Solar Cells, Chem. Mater., 2015, 27, 5941–5948 CrossRef CAS.
- W. Zhou, Z.-G. Zhang, L. Ma, Y. Li and X. Zhan, Dithienocoronenediimidebasedconjugatedpolymersaselectronacceptors for all-polymersolarcells, Sol. Energy Mater. Sol. Cells, 2013, 112, 13–19 CrossRef CAS.
- Y. Jiang, L. Lu, M. Yang, C. Zhan, Z. Xie, F. Verpoorta and S. Xiao, Taking the place of perylene diimide: perylene tetracarboxylic tetraester as a building block for polymeric acceptors to achieve higher open circuit voltage in all-polymer bulk heterojunction solar cells, Polym. Chem., 2013, 4, 5612–5620 RSC.
- C. Lee, H. Kang, W. Lee, T. Kim, K.-H. Kim, H. Y. Woo, C. Wang and B. J. Kim, High-Performance All-Polymer Solar Cells Via Side-Chain Engineering of the Polymer Acceptor: The Importance of the Polymer
Packing Structure and the Nanoscale Blend Morphology, Adv. Mater., 2015, 27, 2466–2471 CrossRef CAS PubMed.
- H. Kang, M. A. Uddin, C. Lee, K.-H. Kim, T. L. Nguyen, W. Lee, Y. Li, C. Wang, H. Y. Woo and B. J. Kim, Determining the Role of Polymer Molecular Weight for High-Performance All-Polymer Solar Cells: Its Effect on Polymer Aggregation and Phase Separation, J. Am. Chem. Soc., 2015, 137, 2359–2365 CrossRef CAS PubMed.
- T. Earmme, Y.-J. Hwang, N. M. Murari, S. Subramaniyan and S. A. Jenekhe, All-Polymer Solar Cells with 3.3% Efficiency Based on Naphthalene Diimide-Selenophene Copolymer Acceptor, J. Am. Chem. Soc., 2013, 135, 14960–14963 CrossRef CAS PubMed.
- Y. Kim, D. S. Chung and C. E. Park, Highly thermallystablenon-fullereneorganic solar cells:p-DTS(FBTTh2)2:P(NDI2OD-T2) bulk heterojunction, Nano Energy, 2015, 15, 343–352 CrossRef CAS.
- Z. Li, J. D. A. Lin, H. Phan, A. Sharenko, C. M. Proctor, P. Zalar, Z. Chen, A. Facchetti and T.-Q. Nguyen, Competitive Absorption and In efficient Exciton Harvesting: Lessons Learned from Bulk Heterojunction Organic Photovoltaics Utilizing the Polymer Acceptor P(NDI2OD-T2), Adv. Funct. Mater., 2014, 24, 6989–6998 CrossRef CAS.
- Z. Tang, B. Liu, A. Melianas, J. Bergqvist, W. Tress, Q. Bao, D. Qian, O. Inganäs and F. Zhang, A New Fullerene-Free Bulk-Heterojunction System for Efficient High-Voltage and High-Fill Factor Solution-Processed Organic Photovoltaics, Adv. Mater., 2015, 27, 1900–1907 CrossRef CAS PubMed.
- J. W. Jung, T. P. Russell and W. H. Jo, A Small Molecule Composed of Dithienopyran and Diketopyrrolopyrrole as Versatile Electron Donor Compatible with Both Fullerene and Nonfullerene Electron Acceptors for High Performance Organic Solar Cells, Chem. Mater., 2015, 27, 4865–4870 CrossRef CAS.
- N. Zhou, H. Lin, S. J. Lou, X. Yu, P. Guo, E. F. Manley, S. Loser, P. Hartnett, H. Huang, M. R. Wasielewski, L. X. Chen, R. P. H. Chang, A. Facchetti and T. J. Marks, Morphology-Performance Relationships in High-Efficiency All-Polymer Solar Cells, Adv. Energy Mater., 2014, 4, 1300785 Search PubMed.
- D. Mori, H. Benten, I. Okada, H. Ohkita and S. Ito, Low-Bandgap Donor/Acceptor Polymer Blend Solar Cells with Efficiency Exceeding 4%, Adv. Energy Mater., 2014, 4, 1301006 Search PubMed.
- K. D. Deshmukh, T. Qin, J. K. Gallaher, A. C. Y. Liu, E. Gann, K. O’Donnell, L. Thomsen, J. M. Hodgkiss, S. E. Watkins and C. R. McNeill, Performance, morphology and photophysics of high open-circuit voltage, low band gap allpolymer solar cells, Energy Environ. Sci., 2015, 8, 332–342 CAS.
- C. Mu, P. Liu, W. Ma, K. Jiang, J. Zhao, K. Zhang, Z. Chen, Z. Wei, Y. Yi, J. Wang, S. Yang, F. Huang, A. Facchetti, H. Ade and H. Yan, High-Efficiency All-Polymer Solar Cells Based on a Pair of Crystalline Low-Bandgap Polymers, Adv. Mater., 2014, 26, 7224–7230 CrossRef CAS PubMed.
- D. Mori, H. Benten, I. Okada, H. Ohkita and S. Ito, Highly Efficient Charge-Carrier Generation and Collection in Polymer/Polymer Blend Solar Cells with a Power Conversion Efficiency of 5.7%, Energy Environ. Sci., 2014, 7, 2939–2943 CAS.
- J. W. Jung, J. W. Jo, C.-C. Chueh, F. Liu, W. H. Jo, T. P. Russell and A. K.-Y. Jen, Fluoro-Substituted n-Type Conjugated Polymers for Additive-Free All-Polymer Bulk Heterojunction Solar Cells with High Power Conversion Efficiency of 6.71%, Adv. Mater., 2015, 27, 3310–3317 CrossRef CAS PubMed.
- J. Choi, K.-H. Kim, H. Yu, C. Lee, H. Kang, I. Song, Y. Kim, J. H. Oh and B. J. Kim, Importance of Electron Transport Ability in Naphthalene Diimide-Based Polymer Acceptors for High-Performance, Additive-Free, All-Polymer Solar Cells, Chem. Mater., 2015, 27, 5230–5237 CrossRef CAS.
- E. Zhou, J. Cong, K. Hashimoto and K. Tajima, Control of Miscibility and Aggregation Via the Material Design and Coating Process for High-Performance Polymer Blend Solar Cells, Adv. Mater., 2013, 25, 6991–6996 CrossRef CAS PubMed.
- S. Subramaniyan, T. Earmme, N. M. Murari and S. A. Jenekhe, Naphthobisthiazole diimide-based n-type polymer semiconductors: synthesis, p-stacking, field-effect charge transport, and all-polymer solar cells, Polym. Chem., 2014, 5, 5707–5715 RSC.
- Y.-J. Hwang, T. Earmme, S. Subramaniyan and S. A. Jenekhe, Side chain engineering of n-type conjugated polymer enhances photocurrent and efficiency of all-polymer solar cells, Chem. Commun., 2014, 50, 10801–10804 RSC.
- Y.-J. Hwang, T. Earmme, B. A. E. Courtright, F. N. Eberle and S. A. Jenekhe, nType Semiconducting Naphthalene Diimide-Perylene Diimide Copolymers: Controlling Crystallinity, Blend Morphology, and Compatibility Toward High-Performance All-Polymer Solar Cells, J. Am. Chem. Soc., 2015, 137, 4424–4434 CrossRef CAS PubMed.
- L. M. Kozycz, D. Gao, A. J. Tilley and D. S. Seferos, One Donor–Two Acceptor (D–A1)-(D–A2) Random Terpolymers Containing Perylene Diimide, Naphthalene Diimide, and Carbazole Units, J. Polym. Sci., Part A: Polym. Chem., 2014, 52, 3337–3345 CrossRef CAS.
- Y. Cao, T. Lei, J. Yuan, J.-Y. Wang and J. Pei, Dithiazolyl-benzothiadiazole-containing polymer acceptors: synthesis, characterization, and all-polymer solar cells, Polym. Chem., 2013, 4, 5228–5236 RSC.
- W. Li, W. S. C. Roelofs, M. Turbiez, M. M. Wienk and R. A. J. Janssen, Polymer Solar Cells with Diketopyrrolopyrrole Conjugated Polymers as the Electron Donor and Electron Acceptor, Adv. Mater., 2014, 26, 3304–3309 CrossRef CAS PubMed.
- D. Bartesaghi, I. del Carmen Pérez, J. Kniepert, S. Roland, M. Turbiez, D. Neher and L. J. A. Koster, Competition between recombination and extraction of free charges determines the fill factor of organic solar cells, Nat. Commun., 2015, 6, 7083 CrossRef CAS PubMed.
|
This journal is © The Royal Society of Chemistry 2015 |
Click here to see how this site uses Cookies. View our privacy policy here.