Mitochondrial biofuel cells: expanding fuel diversity to amino acids
Received
29th July 2010
, Accepted 13th October 2010
First published on 10th November 2010
Abstract
Although mitochondria have long been considered the powerhouse of the living cell, it is only recently that we have been able to employ these organelles for electrocatalysis in electrochemical energy conversion devices. The concept of using biological entities for energy conversion, commonly referred to as a biofuel cell, has been researched for nearly a century, but until recently the biological entities were limited to microbes or isolated enzymes. However, from the perspectives of efficient energy conversion and high volumetric catalytic activity, mitochondria may be a possible compromise between the efficiency of microbial biofuel cells and the high volumetric catalytic activity of enzymatic biofuel cells. This perspective focuses on comparing mitochondrial biofuel cells to other types of biofuel cells, as well as studying the fuel diversity that can be employed with mitochondrial biofuel cells. Pyruvate and fatty acids have previously been studied as fuels, but this perspective shows evidence that amino acids can be employed as fuels as well.
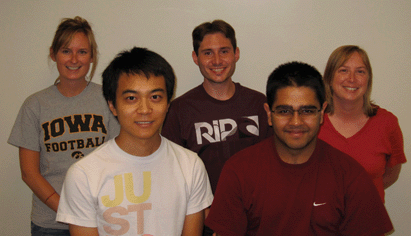
Dushyant Bhatnagar, Shuai Xu, Caitlin Fischer, Robert L. Arechederra, Shelley D. Minteer
| Dushyant Bhatnagar is a summer researcher working in the Department of Chemistry at Saint Louis University as a STARS (Students and Teachers as Research Scientists) Scholar. |
| Shuai Xu received his Bachelor's degree from Tongji University in 2007. He is currently a PhD candidate in the Department of Chemistry at Saint Louis University. His PhD research is focused on deep oxidation via bioelectrocatalysis. |
| Caitlin Fischer received her Bachelor's degree in Chemistry at University of Missouri—Columbia in 2008. She is currently working as a research assistant in the Department of Chemistry at Saint Louis University. Her research is focused on mitochondrial bioelectrocatalysis for sensor applications. |
| Robert Arechederra received his PhD in Analytical Chemistry at Saint Louis University in 2009 and is currently a postdoctoral research associate in the Minteer Research Group at Saint Louis University. His research interests are focused on non-platinum electrocatalysis for fuel cell applications. |
| Shelley D. Minteer received her PhD in Analytical Chemistry at University of Iowa in 2000. She, then, joined the faculty in the Department of Chemistry at Saint Louis University and currently holds the title of College of Arts and Sciences Endowed Professor of Chemistry. She also has a joint appointment in Biomedical Engineering. Her research interests are focused on enzymatic and mitochondrial biofuel cells and biosensors. |
Introduction
Biofuel cells are similar to traditional fuel cells in that they are energy conversion devices that convert the chemical energy of a fuel into electricity. However, biofuel cells perform energy conversion through the use of biological catalysts at the anode and/or the cathode. Biofuel cells have been a researched energy conversion device for almost a century and there is a wealth of literature and reviews on the subject.1–12 The earliest biofuel cells employed microbes as electrocatalysts, but in the 1960s, another type of biofuel cell was developed that employed isolated oxidoreductase enzymes to catalyze the redox reactions in the electrochemical energy conversion device.2 Much research and development has occurred in the field. Generally, these two types of biofuel cells are considered for different applications. Microbial fuel cells have high coulombic efficiency, can deeply or completely oxidize biofuels, and can have long lifetimes (up to 5 years).7,13,14 However, microbial fuel cells typically have low geometric current and power density (i.e. 0.001 to 0.1 mW cm−2 of geometric anode area, although there are a few recent reports of up to 0.69 mW cm−2 of geometric anode area)7,15 due to lower volumetric catalytic activity and performance limitations due to slow mass transport of fuel across cellular membranes.2 They also require operation in environmental conditions (temperature, pH, ionic strength, etc.) that are conducive to microbe survival. These environmental conditions are dependent on the microbe, since there are extremophiles and thermophiles that survive and grow under harsh conditions.16 On the other hand, enzymatic fuel cells traditionally have higher current and power density due to higher volumetric catalytic activity and improved mass transport, but they typically employ a single enzyme at the anode, which results in partial oxidation of simple biofuels and low coulombic efficiency.17 For instance, the most common enzyme system employed in enzymatic biofuel cells is glucose oxidase. Glucose oxidase oxidizes glucose to gluconolactone; thereby, generating 2 electrons for each molecule of glucose. Complete oxidation of glucose to carbon dioxide, which is performed in vivo via the metabolic pathways of glycolysis and the Kreb's cycle, generates 24 electrons per molecule of glucose. Therefore, only 1/12th of the theoretical electrons are generated by employing glucose oxidase at the anode of a biofuel cell, so the efficiency and the energy density are low. Although enzymatic fuel cells have been shown to operate at a wider temperature range (i.e. 10 °C to 65 °C for glycerol)18 than most microbial fuel cells, there are extremophilic microorganisms that allow for microbial fuel cell operation from 4 °C to 45 °C19,20 and possible even wider ranges depending on the extremophilic microbial system. Another consideration with both microbial and enzymatic biofuel cells is that they also have to operate in a pH, ionic strength, and solvent that does not kill the microbe or inhibit/denature the protein. Although a great deal of research has focused on improving lifetimes, enzymatic biofuel cells still have shorter lifetimes than microbial fuel cells. Because of the differences between the two types of biofuel cells, they have been considered for different applications. For instance, enzymatic biofuel cells are frequently considered for implantable power sources due to the almost unlimited supply of fuel (typically glucose) and therefore no need for high efficiency or deep oxidation of fuel. The implantable application is primarily focused at high current density, high power density, and biocompatibility in the human body or other living systems (i.e. plant, cactus, or tree).21 On the other hand, microbial fuel cells have been considered for such applications as wastewater treatment plants15 and sea floor batteries,22 where the size of the biofuel cell is not so important, but lifetime is important. For instance, microbial fuel cells planted on the sea floor can be as large or as small as needed, so there is no concern with current, power, or energy density. However, they need to be extremely stable, because you cannot easily replace components once they have been implanted into the sand at the bottom of the sea.
A few years ago, a new type of biofuel cell was invented employing subcellular organelles, specifically mitochondria,23 as the biocatalyst at the anode of a biofuel cell. A schematic of a mitochondrial bioanode for a biofuel cell is shown in Fig. 1. Mitochondria are the organelle of the living cell that contain several (but not all) of the protein pathways of metabolism, including the citric acid cycle and the electron transport chain. They are responsible for a variety of metabolic processes including fatty acid metabolism and pyruvate oxidation, where pyruvate is produced outside of the mitochondria from glycolytic pathway oxidation of sugars. Mitochondrial biofuel cells have been shown to oxidize pyruvate completely to carbon dioxide and to oxidize simple fatty acids.24 The ability of mitochondria to completely oxidize pyruvate shows that mitochondrial fuel cells can have similar coulombic efficiency and degree of oxidation as microbial fuel cells23 for simple substrates/fuels. However, they have been shown to have current and power densities closer to common enzymatic biofuel cells due to higher volumetric catalytic activity than most microbes. Therefore, they are attractive alternatives for applications that need both deep oxidation of simple fuels, which results in high energy density, and high current and power densities. Note: more complex fuels, like sugars, cannot be utilized by mitochondria, because mitochondria do not contain the enzymes of the glycolytic pathway. Essentially, they lend themselves to biofuel cells or biobatteries with both high energy density and high power density. In the simplest terms, they are interesting for electrical applications that require a high battery drain rate and long battery lifetime between “recharge”.
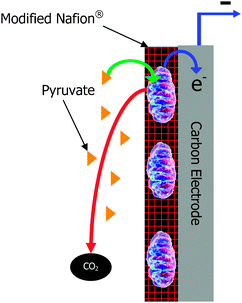 |
| Fig. 1 Schematic of a mitochondrial bioanode. | |
Mitochondrial biofuel cells are similar to enzymatic and microbial biofuel cells in that they can employ either mediated electron transfer (MET) or direct electron transfer (DET). Direct electron transfer is the process by which mitochondria can transfer electrons from the surface of the mitochondria directly to the electrode surface. Mediated electron transfer is the process by which a small molecule and/or polymeric redox mediator is needed to transfer electrons from the mitochondria to the electrode. Mitochondria are intriguing, because they are capable of direct electron transfer through cytochrome c and FAD/FADH2 to carbon electrodes,25 whereas it is more difficult to do mediated electron transfer unless the mitochondria are lysed and exist as submitochondrial particles.24,25 It is important to note that although lysed mitochondria contain all of the enzymes of the Kreb's cycle metabolic pathway, they do not provide the same pyruvate energy conversion potential as the intact mitochondria. Fig. 2 shows representative power curves of the performance of a pyruvate/air mitochondrial biofuel cell with intact mitochondria versus lysed mitochondria. These data illustrate that mediated electron transfer with mitochondria give much lower (<10%) power densities than mitochondria-based biofuel cells employing direct electron transfer. It is clear that both (a) the three dimensional structures or complexes (called metabolons) that exist in the mitochondria must be preserved for efficient energy conversion via the Kreb's cycle and (b) the surface interaction between the electron transport chain complexes and the electrode surface improves electrochemical performance compared to the use of external mediators. It is also important to note that the biofuel cell community would prefer to work in the absence of required mediators, because mediators add complexity to biofuel cell systems and they frequently have limited lifetime, because redox reactions are not completely reversible and therefore the mediators have a maximum number of turnovers before the mediator needs to be replaced.
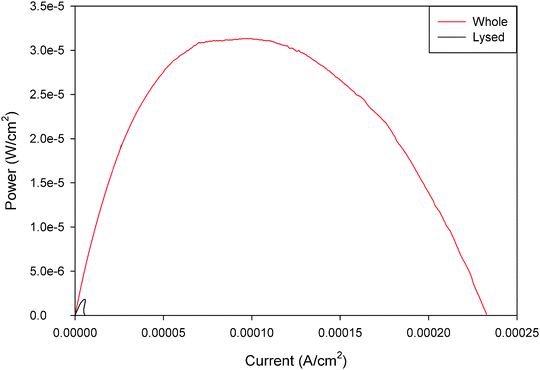 |
| Fig. 2 Representative power curves for unmediated whole and poly(methylene green) mediated lysed mitochondrial bioanodes in pyruvate/air biofuel cell. Both fuel solutions contained 10 mM pH 7.45 phosphate buffer with 100 mM pyruvate fuel and 6 M NaNO3 supporting electrolyte with 1 mg ml−1 ADP. The lysed bioanodes had an additional 1 mg ml−1 of NAD+ in the fuel solution.24 Reprinted with permission from Elsevier. | |
The majority of mediators employed in mitochondrial biofuel cells have been the same type of redox mediators employed in FAD and NAD-dependent oxidoreductase-based enzymatic biofuel cells. Poly(methylene green) being the most common polymeric redox mediator and ferricyanide being the most common small molecule mediator. Clearly, both of these mediator systems have limited stability and reversibility, so if they are employed, they greatly limit the lifetime of the biofuel cell. However, other mediators could be employed, so long as they have appropriate redox potential, good reversibility, and low overpotential on traditional electrode materials (carbon paper, glassy carbon, gold, etc.). For instance, recent work with osmium redox hydrogels,5 metallacarboranes,26 and ferrocene-based redox polymers,27 which have all been discussed for enzymatic biofuel cells could also be employed for mitochondrial biofuel cells.
Mitochondrial immobilization at electrode surfaces
When considering employing mitochondria as catalysts at the anode of a biofuel cell, it is important to consider that the catalyst needs to be immobilized at the surface of the electrode. Mitochondria can be effectively immobilized at carbon electrodes,25 as well as gold electrodes.28 Researchers have most commonly employed glassy carbon electrodes, Toray carbon fiber paper electrodes, and pyrolytic carbon for mitochondria modified electrodes. In theory, mitochondria are like enzymes and living cells and can be immobilized at these electrode surfaces by a variety of methods. The earliest method for immobilizing mitochondrion at an electrode surface was to “sandwich” a suspension of mitochondria in buffer between an electrode and a dialysis membrane.29 This technique is good in that it is a mild technique that does not disrupt the mitochondrial membrane structure. However, this technique results in a long diffusional distance between the mitochondria and the electrode, which prohibits direct electron transfer. This technique also doesn't provide any buffering or structural protection to the mitochondria. Interestingly, this technique does show the relative stability of mitochondria. Mitochondria sandwiched between an electrode and a dialysis membrane have a lifetime of greater than 10 days,29 so they are actually more stable than most enzymes in buffer solutions. This is not intuitive in that most people would consider an organelle to be more fragile than an isolated enzyme, but the mitochondrial membranes are quite robust.
Other techniques for immobilizing mitochondria include crosslinking of mitochondria to a carbon electrode via glutaraldehyde in the presence of bovine serum albumin for stabilization.25 This harsh crosslinking technique will likely decrease catalytic activity, but increase stability. This would result in longer operational lifetimes, but lower current densities. A final immobilization material is the use of hydrophobically modified Nafion.30 Hydrophobically modified Nafion has been used for a wide variety of enzyme immobilization applications.31–34 The theory behind the use of hydrophobically modified Nafion for enzyme immobilization has been the ability to encapsulate the proteins in the amphiphilic micellar pocket structures of Nafion. In the case of mitochondria, these organelles are too large for encapsulation in the micellar structures, but the amphiphilic membrane-like properties of the modified Nafion provide a stabilizing effect. Greater than two months of stability has been observed with mitochondria in tetrabutylammonium bromide modified Nafion in a biofuel cell environment. However, it is important to note that mitochondria do not contain all of the cellular machinery for regeneration of all of their metabolic enzymes, so we believe that the active lifetime of the mitochondria-based biofuel cells will be lower than microbial fuel cells.
Fuel diversity
Mitochondria are considered the “powerhouse” of the living cell, because they have very high volumetric catalytic activity for oxidation. This is due to the high concentration of metabolic enzymes in the mitochondria. The mitochondria contain the enzymes of the Krebs cycle. These 9 enzymes of the cycle can oxidize pyruvate to carbon dioxide in an indirect manner. There are 5 oxidoreductase enzymes: pyruvate dehydrogenase, isocitrate dehydrogenase, ketoglutarate dehydrogenase, succinate dehydrogenase, and malate dehydrogenase that catalyze oxidation reactions, but there are also 4 other enzymes that catalyze other chemical reactions to rearrange the reactant molecules for the next step of oxidation. This complex pathway contains enzymes with attributes to maintain metabolic control. It contains enzymes with substrate inhibition, product inhibition, and coenzyme dependencies that allow for the maintenance of appropriate metabolic control in a method similar to what is experienced with microbes in microbial fuel cells, but completely different than what is typically thought with metallic electrocatalysis in traditional fuel cells. Another aspect of the Krebs cycle in the mitochondria that is noteworthy is the fact that the enzymes in the mitochondria are in a three-dimensional structure called a metabolon. This nanostructure helps to ensure substrate channeling from one enzyme of the cycle to the next enzyme of the cycle, which minimizes transport effects and improves efficiency. Mitochondria also contain the electron transport chain, which allow for the transport of electrons generated from oxidation of the fuel in the Kreb's cycle to the outer surface of the mitochondria for communication with the electrode surface. This component of the mitochondria is critical to the ability to use mitochondria in a direct electron transfer mode in biofuel cells.
Mitochondria are interesting organelles, because they have the biological machinery to metabolize pyruvate and its metabolites and fatty acids and their metabolites, but they also have biological machinery for breaking down amino acids. Researchers have shown mitochondrial modified electrodes capable of oxidation of pyruvate,24 succinate,25 and fatty acids.24 Pyruvate is the main substrate for the Kreb's cycle and the mitochondria have mechanisms for efficiently transporting pyruvate into the mitochondria, but since the Kreb's cycle is a cycle, then the input fuel/substrate is flexible. Metabolites such as succinate, malate, fumarate, acetyl-CoA, citrate, isocitrate, and ketoglutarate can be considered as fuels, because these are components of the cycle.
Since mitochondria contain the mechanisms for fatty acid metabolism, then a wide variety of fatty acids have or can be considered as fuels. Currently, the fuel cell design employed is not a membrane electrode assembly (MEA) design and instead has a gap between the anode and the polymer electrode membrane/separator. This gap contains fuel solution as shown in Fig. 3. Therefore, the fuel solution must be a high conductivity aqueous fuel solution with large electrolyte concentrations to minimize internal resistance. This limits the choice of fatty acids, because only fatty acids with some reasonable solubility in water can be utilized. In theory, if the fuel cell design is transferred from a liquid system to the MEA style system typically employed in traditional fuel cells, then all liquid fatty acid solutions could be employed.
As we continue to explore mitochondrial biofuel cells, it becomes obvious that there are other fuels to consider. Mitochondria are well known for their ability to oxidize amino acids, which are the building block of proteins. There is not one central pathway for amino acid breakdown/metabolism, but there are several pathways, most of which break down the amino acids into substrates that can be further oxidized by the Kreb's cycle. For instance, glycine, alanine, tryptophan, threonine, serine, and cysteine are broken down to form pyruvate, which is the natural substrate of the Kreb's cycle. Proline, glutamate, histidine, arginine, and glutamine are broken down to form ketoglutarate, which is a key metabolite in the Kreb's cycle. Methionine, isoleucine, and valine are broken down to form succinyl-CoA, which is another key metabolite down the Kreb's cycle pathway from ketoglutarate. On the other hand, asparagines and aspartate/aspartic acid are broken down to oxaloacetate and feed into the back side of the Kreb's cycle. Fig. 4 shows a schematic of several amino acid metabolic pathways into the Kreb's cycle. The enzymes shown are mitochondrial enzymes and these amino acids have been shown to either enter the mitochondria passively or by active transport.35
For this reason, we have recently started studying the ability of amino acids to be oxidized by a mitochondrial bioanode in a biofuel cell. Glycine, glutamate, aspartic acid, serine, proline, valine, methionine, and cysteine were studied in a mitochondrial biofuel cell and all amino acids were metabolized and resulted in current generation with 100 mM fuel concentrations in the biofuel cell containing the mitochondrial bioanode and an air breathing commercial platinum cathode. Table 1 shows the performance data for fuel cell utilizing different amino acids. It is interesting to note that the fuel cell performance is dependent on the amino acid fuel choice, but all amino acids show higher current density and maximum power density than the same concentration of pyruvate. The highest performing amino acid was cysteine with a current density of 185 ± 39 μA cm−2 and a power density of 10.3 ± 1.4 μW cm−2, but it is also different in that it is the only amino acid tested that is electroactive, so the results in Fig. 5 compare the response of cysteine in mitochondrial bioanode versus a bioanode that had been coated with immobilization polymer, but no mitochondria (control experiment). There is clear bioelectrocatalysis shown for cysteine. Fig. 5 shows a 11.8 fold increase in current density associated with bioelectrocatalysis of cysteine versus non-catalytically oxidized cysteine and a 11.6 fold increase in power density. The second highest performance was for aspartic acid/aspartate, although statistically the performance is not different from the fuel cell performance for valine, proline, methionine, serine, and glutamine. Overall, these biofuel cell experiments show that amino acids can indeed be employed as fuels in mitochondrial biofuel cells. The performance of the fuel cells with amino acids outperform pyruvate electrochemically (i.e. current density, potential, power density), which is similar to behavior observed in biofuel cells for fatty acids. Lifetime has not been tested for amino acid fueled biofuel cells, but it is expected that lifetime is shorter due to the fact that not all of the enzymes of the urea cycle are present in mitochondria and so the build up of high concentrations of NH4+ will limit lifetime.
Table 1 Data from polarization and power curves of pyruvate and amino acid fueled mitochondrial biofuel cells. Mitochondrial bioanodes were fabricated as described in ref. 23, with the exception of the anode material which is Fuel Cell Earth Toray paper that is lower in surface area than the E-Tek Toray paper described in ref. 23. Cathode and fuel cell test setup were as described in ref. 24. The fuel solutions contained 100 mM fuel in pH 8.0 phosphate buffer with 6 M sodium nitrate electrolyte. Electrodes were allowed to equilibrate in the fuel solution for a minimum of 30 minutes and polarization curves were run on a CH Instruments Model 650 potentiostat. Since cysteine is electrochemically active, a control experiment was done with no mitochondrial bioelectrocatalyst
Amino acid |
Open circuit potential/V |
Current density at 0 V/μA cm−2 |
Maximum power density/μW cm−2 |
Pyruvate |
0.515 ± 0.032 |
2.4 ± 0.3 |
0.15 ± 0.01 |
Aspartic acid |
0.841 ± 0.011 |
25.4 ± 13.5 |
2.57 ± 1.70 |
Serine |
0.734 ± 0.028 |
15.1 ± 4.1 |
1.42 ± 0.30 |
Glycine |
0.566 ± 0.019 |
3.0 ± 0.5 |
0.20 ± 0.01 |
Valine |
0.714 ± 0.013 |
13.9 ± 3.1 |
1.18 ± 0.24 |
Glutamine |
0.611 ± 0.094 |
17.9 ± 10.9 |
0.96 ± 0.17 |
Methionine |
0.716 ± 0.007 |
13.2 ± 3.6 |
1.35 ± 0.44 |
Proline |
0.761 ± 0.071 |
17.2 ± 8.0 |
1.07 ± 0.40 |
Cysteine |
0.678 ± 0.062 |
185 ± 39 |
10.3 ± 1.4 |
Cysteine control (no mitochondria) |
0.631 |
15.7 |
0.89 |
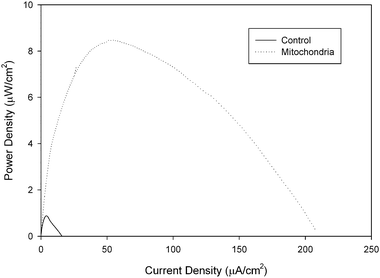 |
| Fig. 5 Representative power curves of a 100 mM cysteine/air biofuel cell employing a mitochondrial modified bioanode versus a control bioanode that has only been coated with tetrabutylammonium bromide modified Nafion immobilization polymer. Experiments were performed at room temperature. | |
Concluding views
Mitochondrial biofuel cells are an interesting new class of biofuel cells, because they have some of the attractive properties of microbial biofuel cells (high efficiency and deep or complete oxidation of fuel), but they also have some of the attractive properties of enzymatic biofuel cells (high current and power density as well as high volumetric catalytic activity). Researchers have shown they can employ fatty acids, pyruvate, and succinate as fuels, but this perspective shows experimental evidence that mitochondrial biofuel cells can also employ amino acids, the building blocks of proteins, as fuel. Although amino acids have been considered as biofuels for other types of biofuel cells, they can also be utilized for mitochondria-based biofuel cells and they may lead to interesting possibilities. Amino acids are abundant in biological solutions. However, amino acids, especially in the dilute concentrations normally observed in biological solutions, are not really any more energy dense than carbohydrates and carbohydrate metabolites and obviously less dense than oils and fatty acids. However, this work leads us to consider the importance of fuel diversity in fuel cell applications. Very few solutions that would be considered fuels for different applications of biofuel cells (i.e. human serum, tree sap, or wastewater) are pure fuels or a pure fuel dissolved in water or electrolyte. Most of these solutions are complex mixtures that contain a variety of possible biofuels. In this case, in order to get maximum energy density from the biofuel cell, the biofuel cell needs to be able to oxidize different components in the complex mixtures. Therefore, mitochondrial biofuel cells are interesting from the perspective of fuel diversity. They oxidize a wide variety of different fuels and therefore may be advantageous in applications where complex fuel mixtures are present.
Acknowledgements
The authors would like to thank the National Science Foundation for generous funding. DB would also like to thank the Students and Teachers as Research Scientists (STARS) program for support.
References
- S. C. Barton, J. Gallaway and P. Atanassov, Chem. Rev., 2004, 104, 4867–4886 CrossRef CAS
.
- G. Palmore and G. M. Whitesides, ACS Symp. Ser., 1994, 566, 271–290 CAS
.
- M. J. Cooney, V. Svoboda, C. Lau, G. P. Martin and S. D. Minteer, Energy Environ. Sci., 2008, 1, 320–337 RSC
.
- A. Heller, Phys. Chem. Chem. Phys., 2004, 6, 209–216 RSC
.
- A. Heller, Curr. Opin. Chem. Biol., 2006, 10, 664–672 CrossRef CAS
.
- R. A. Bullen, T. C. Arnot, J. B. Lakeman and F. C. Walsh, Biosens. Bioelectron., 2006, 21, 2015–2045 CAS
.
- B. E. Logan, Appl. Microbiol. Biotechnol., 2010, 85, 1665–1671 CrossRef CAS
.
- F. Davis and S. P. J. Higson, Biosens. Bioelectron., 2007, 22, 1224–1235 CrossRef CAS
.
- J. Kim, H. Jia and P. Wang, Biotechnol. Adv., 2006, 24, 296–308 CrossRef CAS
.
- M. Pita and E. Katz, Electroanalysis, 2009, 21, 252–260 CrossRef CAS
.
- A. Ramanavicius and A. Ramanaviciene, Fuel Cells, 2009, 9, 25–36 CrossRef CAS
.
- I. Willner, Y. M. Yan, B. Willner and R. Tel-Vered, Fuel Cells, 2009, 9, 7–24 CrossRef CAS
.
- M. J. Moehlenbrock and S. D. Minteer, Chem. Soc. Rev., 2008, 37, 1188–1196 RSC
.
- M. Balat, Energy Sources, Part A: Recovery, Util. Environ. Eff., 2010, 32, 26–35 Search PubMed
.
- B. H. Kim, I. S. Chang and G. M. Gadd, Appl. Microbiol. Biotechnol., 2007, 76, 485–494 CrossRef CAS
.
- B. J. Mathis, C. W. Marshall, C. E. Milliken, R. S. Makkar, S. E. Creager and H. D. May, Appl. Microbiol. Biotechnol., 2008, 78, 147–155 CrossRef CAS
.
- D. Sokic-Lazic, R. L. Arechederra, B. L. Treu and S. D. Minteer, Electroanalysis, 2010, 22, 757–764 CAS
.
- R. L. Arechederra and S. D. Minteer, Fuel Cells, 2009, 9, 63–69 CrossRef CAS
.
- S. Cheng, D. Xing and B. E. Logan, Biosens. Bioelectron., 2010 Search PubMed
, in press.
- S. A. Patil, F. Harnisch, B. Kapadnis and U. Schroder, Biosens. Bioelectron., 2010, 26, 803–808 CrossRef CAS
.
- S. Calabrese-Barton, J. Gallaway and P. Atanassov, Chem. Rev., 2004, 104, 4867–4886 CrossRef CAS
.
- L. M. Tender, C. E. Reimers, H. A. Stecher, D. E. Holmes, D. R. Bond, D. A. Lowy, K. Pilobello, S. J. Fertig and D. R. Lovley, Nat. Biotechnol., 2002, 20, 821–825 CAS
.
- R. L. Arechederra and S. D. Minteer, Electrochim. Acta, 2008, 53, 6698–6703 CrossRef CAS
.
- R. L. Arechederra, K. Boehm and S. D. Minteer, Electrochim. Acta, 2009, 54, 7268–7273 CrossRef CAS
.
- J. Zhao, F. Meng, X. Zhu, K. Han, S. Liu and G. Li, Electroanalysis, 2008, 20, 1593–1598 CrossRef CAS
.
- S. W. Buckner, P. A. Jelliss, A. Nukic, E. R. Zalocusky and J. Schumacher, Bioelectrochemistry, 2010, 78, 130–134 CrossRef CAS
.
- S. A. Merchant, T. O. Iran, M. T. Meredith, T. C. Cline, D. T. Glatzhofer and D. W. Schmidtke, Langmuir, 2009, 25, 7736–7742 CrossRef CAS
.
-
United States Patent Application 12/059,212, 2008 Search PubMed
.
- M. A. Arnold and G. A. Rechnitz, Anal. Chem., 1980, 52, 1170–1174 CrossRef CAS
.
-
United States Patent Application 12/373,777, 2007 Search PubMed
.
- C. M. Moore, N. L. Akers, A. D. Hill, Z. C. Johnson and S. D. Minteer, Biomacromolecules, 2004, 5, 1241–1247 CrossRef CAS
.
- C. M. Moore, S. Hackman, T. Brennan and S. D. Minteer, J. Membr. Sci., 2005, 255, 233–238 CrossRef CAS
.
- T. L. Klotzbach, M. M. Watt, Y. Ansari and S. D. Minteer, J. Membr. Sci., 2006, 282, 276–283 CrossRef CAS
.
- T. L. Klotzbach, M. M. Watt, Y. Ansari and S. D. Minteer, J. Membr. Sci., 2008, 311, 81–88 CrossRef CAS
.
- S. Passerella, A. Atlante, D. Valenti and L. de Bari, Mitochondrion, 2003, 2, 319–343 CrossRef
.
|
This journal is © the Owner Societies 2011 |
Click here to see how this site uses Cookies. View our privacy policy here.