DOI:
10.1039/C8NH00101D
(Communication)
Nanoscale Horiz., 2018,
3, 551-555
High Curie-temperature intrinsic ferromagnetism and hole doping-induced half-metallicity in two-dimensional scandium chlorine monolayers†
Received
7th May 2018
, Accepted 18th July 2018
First published on 19th July 2018
Abstract
Two-dimensional (2D) ferromagnetic materials provide new platforms for spintronic applications, but most of the reported 2D magnetic orderings can only be maintained at low temperature. The search for high Curie temperature intrinsic ferromagnetism is still a current hotspot. Herein through comprehensive first-principles calculations and Monte Carlo simulation, we predict a promising 2D scandium chlorine (ScCl) monolayer with intrinsic ferromagnetism and a Curie temperature of 185 K, which is much higher than that of the reported CrI3 monolayer (45 K) and the boiling point of liquid nitrogen (77 K). Moreover, a small amount of hole doping can induce a transition from a ferromagnetic metal to a half-metal. Furthermore, the ScCl monolayer possesses excellent thermal and dynamical stabilities as well as feasibility of experimental exfoliation from its layered bulk. These intriguing electronic and magnetic properties make the ScCl monolayer a promising candidate for spintronic applications.
Conceptual insights
High Curie-temperature ferromagnetic two-dimensional (2D) materials with large spin polarization have been a long-sought goal due to their potential in spintronics applications. Here we report a promising 2D scandium chlorine (ScCl) monolayer with intrinsic ferromagnetism and high Curie temperature up to 185 K. Moreover, a small amount of hole doping can turn the ScCl monolayer into a half-metal and further improves the magnetic moment and Curie temperature. The novel ferromagnetism can also be extended to ScCl bilayers. In addition, the ScCl monolayer possesses excellent thermal and dynamical stabilities and great feasibility of experimental exfoliation from its layered bulk.
|
Introduction
Spintronics, which utilizes the spin degree of freedom of electrons for information processing, has attracted enormous interest in science and technology.1 To fabricate spintronic devices at the nanoscale, one of the key issues is to develop low-dimensional intrinsic ferromagnetic (FM) materials with large spin polarization and high Curie temperature. In recent years, plenty of two-dimensional (2D) materials have widely emerged with exceptional properties and potential applications.2 Nevertheless, most of them lack intrinsic ferromagnetic properties, which has limited their practical applications in spintronics. The absence of ferromagnetic ordering has stimulated great efforts to acquire 2D ferromagnetic materials by defect engineering,3–5 strain tuning,6–8 doping,9–11 surface adsorption,12 and heterostructures.13,14 For instance, the antiferromagnetic (AFM) MnPSe3 monolayer9 and nonmagnetic InP3 monolayer10 become ferromagnetic under carrier doping. Most recently, the ferromagnetic ordering of monolayer VSe2/van der Waals heterostructures persists above room temperature.13 However, achieving robust long-range ferromagnetism in 2D systems is still very challenging.15
Ferromagnetic Cr2Ge2Te6 few layers16,17 and CrI3 monolayers18 have been successfully exfoliated from their layered bulk forms, opening the door for the application of 2D spintronics at the nanoscale. Besides CrI3 monolayers, various binary transition metal halides have also attracted much attention because of their fascinating physical and chemical properties.19–26 Among them, transition metal trihalides (CrCl3 and CrBr3) were theoretically predicted to have ferromagnetic orderings with Curie temperatures of 66 and 86 K,20 respectively, while NiCl3 monolayers showed spin-gapless semiconducting properties and ferromagnetism.25 Transition metal dihalide TMX2 (TM = V, Cr, Fe, Co, Ni, Mn) nanosheets with different magnetic states were also revealed computationally,26 and monolayer FeCl2 presented a half-metallic ground state with a Curie temperature of 17 K.23 Clearly, the Curie temperatures of these intrinsic 2D magnetic orderings can only be maintained at rather low temperature, such as 30 K for the Cr2Ge2Te6 bilayer and 45 K for the CrI3 monolayer, and thus their direct practical applications are hindered. Therefore, it is highly desirable to design new intrinsically ferromagnetic 2D crystals with robust spin ordering and high Curie temperature simultaneously.
In this work, by using ab initio density functional calculations, we investigate a novel class of 2D materials-transition metal chloride monolayers (TMCl; TM = Sc, Y, Zr), which have layered structures in their bulk form.27–29 Very interestingly, the ScCl monolayer possesses intrinsic ferromagnetism and large spin polarization. Monte Carlo simulations on the basis of the 2D Heisenberg Hamiltonian model show that the Curie temperature can be as high as 185 K for the ScCl monolayer. Moreover, a low concentration of hole doping in the ScCl monolayer can turn the ferromagnetic metal into a half-metal. Its dynamical and thermal stabilities are also confirmed by our phonon dispersion calculations and ab initio molecular dynamics (AIMD) simulations, respectively. Meanwhile, both YCl and ZrCl monolayers are nonmagnetic metals (Table S1 and Fig. S1, ESI†), which will only be discussed in the ESI.†
Results and discussion
Bulk ScCl, which possesses closely packed quadruple layers (Fig. 1a), has been known as a family of layered materials since the 1970s.27 Top and side views of a ScCl monolayer exfoliated from the bulk phase are shown in Fig. 1b. It consists of double hexagonal Sc atomic layers sandwiched by two hexagonal halogen atomic layers in the layering sequence of Cl–Sc–Sc–Cl. Mechanical cleavage is one of the most popular techniques to produce single and few layers from the layered bulk counterparts.30 To examine the feasibility of fabricating ScCl monolayers through exfoliation, we calculated the cleavage energy from a 3-layer slab, which mimics the bulk form. It can clearly be seen from Fig. 1c that the cleavage energy gradually increases with the separation d − d0 and converges to 0.27 J m−2, which is notably less than the experimental value of graphite (0.36 J m−2).31 Note that our calculated cleavage energy (0.34 J m−2) of graphite matches well with the experimental value (0.36 J m−2), indicating the reliability of the present calculation.
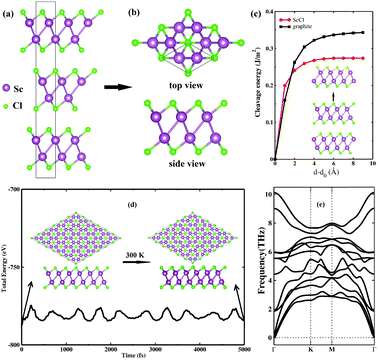 |
| Fig. 1 (a) Crystal structure of bulk ScCl. (b) Top and side views of the ScCl monolayer. (c) Calculated exfoliation energy vs. separation distance (d − d0) in comparison with graphite, where d0 indicates the van der Waals gap between adjacent layers in the bulk crystal. (d) Evolution of total energy and snapshots of the ScCl monolayer from AIMD simulations at 0 and 5 ps. (e) Phonon dispersion of the ScCl monolayer. | |
The stability of a 2D crystal is crucial for its experimental fabrication and practical applications. AIMD simulations are performed to test the room-temperature thermal stability of the ScCl monolayer. As presented in Fig. 1d, snapshots of the geometries after annealing at 300 K for 5 ps show that the ScCl monolayer can keep its original configuration unbroken. Small fluctuations of energy with time during the simulations further confirm its thermal stability. All the phonon branches of the ScCl monolayer have real frequencies along the high-symmetry paths in the Brillouin zone (Fig. 1e), proving its dynamical stability. The small cleavage energy and excellent stabilities strongly suggest that the freestanding 2D ScCl monolayer could possibly be prepared in the experiment and survive at room temperature.
The magnetic and electronic properties of the ScCl monolayer are further investigated. The ScCl monolayer energetically prefers FM coupling among all the considered magnetic configurations (Fig. S2, ESI†). The FM configuration is 64 meV and 128 meV lower in energy than the AFM1 and AFM2 ones per unit cell, respectively. The spin density of the FM state is shown in Fig. 2a and the magnetic moments are mainly localized on the Sc atoms. The total magnetic moment of the ScCl monolayer is as high as 1.56 μB per cell, indicating a large spin polarization in this 2D crystal. The electronic band structure calculation indicates that the ScCl monolayer is a magnetic metal (Fig. 2b). The metallic band structure is also confirmed by the high-accuracy hybrid HSE06 functional32 as shown in Fig. 2c. A close examination of the projected density of states (DOS) reveals that the magnetism in the ScCl monolayer is mainly contributed by the 3d orbital of the Sc atom (Fig. 2d).
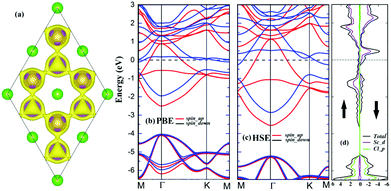 |
| Fig. 2 (a) Spin density for ferromagnetic states with an iso-value of 0.065 e Å−3. Band structures of the ScCl monolayer calculated at the PBE level (b) and HSE level (c) together with the corresponding projected density of states (d). | |
The Curie temperature (Tc) is a key parameter for the practical application of spintronic devices. Therefore, it is necessary to understand the behavior of the magnetism with temperature. Based on the Heisenberg model, the Tc of the ScCl monolayer can be estimated by using Monte Carlo (MC) simulations:
where
J1 and
J2 are the first and second nearest neighboring exchange parameters (Fig. S3, ESI
†), respectively.
Si is the spin vector of each atom,
Szi is the spin component along the
z direction, and
A is the anisotropy energy parameter (more details in the ESI
†). Based on the calculated total energies of different magnetic configurations, the exchange parameters
J1 and
J2 are calculated to be 37.3 meV and 35.3 meV, respectively. Within the MC scheme, the spins on all magnetic sites flip randomly. We employed a 2D 100 × 100 supercell with 10
5 MC steps for each temperature. Providing the exact solution to the spin Hamiltonians,
Tc can be estimated by locating the peak position of magnetic susceptibility.
Fig. 3a presents the calculated magnetic moment and magnetic susceptibility as functions of temperature. It shows that the magnetic moment decreases to zero at about 220 K and the magnetic susceptibility reaches the peak at 185 K. Thus, the estimated Curie temperature is about 185 K, which is much higher than the recently reported Cr
2Ge
2Te
6 bilayer (30 K)
16 and CrI
3 monolayer (45 K).
18 Using the same approach, our calculated
Tc for the CrI
3 monolayer is around 43 K as shown in Fig. S4 (ESI
†), which is in excellent agreement with the experimental measurement of 45 K.
18 The high
Tc indicates that the ScCl monolayer may be a promising spintronic material.
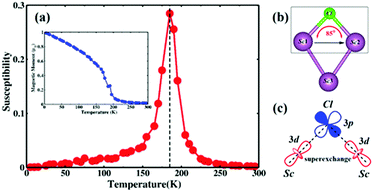 |
| Fig. 3 (a) Variations of the average magnetic moment (blue) and susceptibility (red) for the ScCl monolayer with respect to temperature. (b) Schematic mechanism of superexchange (Sc1–Cl–Sc2) interaction. (c) Mechanism of superexchange interaction for a 90° bond angle. | |
The microscopic origin of the exchange interactions of the ScCl monolayer can be well understood by the coexistence of direct exchange (Sc1–Sc3) and superexchange (Sc1–Cl–Sc2) mediated through the Cl atom as shown in Fig. 3b. From the values above, the nearest neighboring (NN) exchange interaction J1 and the next nearest-neighboring (NNN) exchange interaction J2 are positive, indicating that the ScCl monolayer prefers FM coupling. The NN interaction J1 comes from the direct Sc1–Sc3 exchange interactions and the NNN interaction J2 can be well understood from the superexchange interaction between the Sc1 and Sc2 atoms mediated through the neighboring Cl atom. According to the Goodenough–Kanamori–Anderson (GKA) rule,33–35 in systems with the bond angle of 90°, the superexchange interaction between TM atoms is always ferromagnetic. The bond-angle (85°) of Sc1–Cl–Sc2 as shown in the black box of Fig. 3b is close to 90°, which means that the Sc d orbitals are nearly orthogonal to the Cl p orbitals. This leads to a negligible overlap integral S (Fig. 3c). According to Launay et al.,36 the exchange integral J2 can be written as J2 ≈ 2k + 4βS. k is the potential exchange, which is positive favoring ferromagnetism due to the Hund's rule. β and S are the hopping and overlap integral, respectively. Due to the near zero overlap integral S, J2 is reduced to 2k, which is positive. As a result, the ScCl monolayer adopts a ferromagnetic ordered ground state.
A half-metal (HM), which acts as a conductor in one spin channel and as a semiconductor in another channel, can provide 100% spin-polarized carriers and has great advantages for spintronic applications.37,38 Previous studies have shown that it is likely to induce magnetic transition by carrier doping.9,10,39–41 Due to the unpaired d electrons, carrier doping is expected to manipulate the electronic and magnetic properties in the ScCl monolayer. As shown in Fig. 4a, with a low concentration of hole or electron doping, the ScCl monolayer retains its metallic nature. Further increasing the hole hoping concentration induces a transition from a ferromagnetic metal to a half-metal. The critical point occurs at about 2.26 × 1014 cm−2, above which the ScCl monolayer is a half-metal over a wide range. The corresponding band structure is calculated at different hole doping concentrations for the ScCl monolayer, as shown in Fig. 4b and Fig. S5 (ESI†), for which the half-metallicity with a 100% spin polarization ratio at the Fermi level is realized. The half-metallic property is also confirmed by the more accurate hybrid HSE06 functional32 (Fig. 4c) and the corresponding DOS is presented in Fig. S6 (ESI†). We further calculate the relative energy between FM and AFM states as a function of doping carrier concentration and the FM state is always lower than the AFM state (see Fig. S9, ESI†), indicating that the FM state is quite robust. Note that a charge density modulation of ∼1015 cm−2 has already been experimentally achieved in 2D systems by using an ionic liquid as a gate dielectric.42–44 Therefore, the tunable hole doping can be readily achieved for the ScCl monolayer using currently available gating techniques.
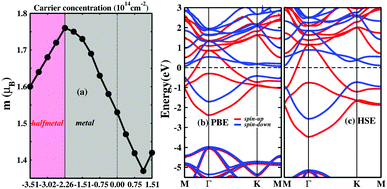 |
| Fig. 4 (a) Total magnetic moments of the ScCl monolayer as a function of carrier concentration, where positive and negative values are for electron and hole doping, respectively. Band structures of the ScCl monolayer at the PBE level (b) and HSE level (c) at the hole doping concentration of 2.26 × 1014 cm−2. The Fermi level is set as zero. | |
Conclusions
In summary, we have predicted a new promising 2D ferromagnetic ScCl monolayer with large spin polarization. The estimated Curie temperature of the ScCl monolayer can be as high as 185 K, which is much higher than the recently reported Tc of 2D Cr2Ge2Te6 (30 K) and CrI3 (45 K) crystals, and even apparently higher than the boiling point of liquid nitrogen (77 K). The ferromagnetic property of the ScCl monolayer is derived from the coexistence of direct nearest-neighboring d–d exchange and superexchange via Cl p states. Moreover, a low concentration of hole doping can turn it into a perfect half-metal, which possibly achieves 100% spin polarization. Meanwhile, this magnetism is not limited to the ScCl monolayer. The ScCl bilayer is also ferromagnetic, and the energy difference EAFM–FM is larger than that of the monolayer, indicating that the ferromagnetism is enhanced with the increase of layers (more details in the ESI†). However, we have to point out that bulk ScCl is not the most stable binary phase of Sc and Cl. However, it is only 11 meV per atom above the solid line of the convex hull (see Fig. S10, ESI†). Considering the excellent dynamical and thermal stability of the ScCl monolayer, and the fact that its cleavage energy is even smaller than that of graphene, the experimental fabrication of ScCl monolayers might be feasible although it is still very challenging.
Conflicts of interest
There are no conflicts to declare.
Acknowledgements
This work is supported by the National Key Research and Development Program of China (2017YFA0204800), the Natural Science Foundation of China (21525311, 21773027), Jiangsu 333 project (BRA2016353), the Scientific Research Foundation of Graduate School of Southeast University (YBJJ1773), and the Fundamental Research Funds for the Central Universities of China. The authors gratefully acknowledge the computational resources at the SEU and National Supercomputing Center in Tianjin.
Notes and references
- A. Fert, Rev. Mod. Phys., 2008, 80, 1517–1530 CrossRef.
- G. R. Bhimanapati, Z. Lin, V. Meunier, Y. Jung, J. Cha, S. Das, D. Xiao, Y. Son, M. S. Strano, V. R. Cooper, L. Liang, S. G. Louie, E. Ringe, W. Zhou, S. S. Kim, R. R. Naik, B. G. Sumpter, H. Terrones, F. Xia, Y. Wang, J. Zhu, D. Akinwande, N. Alem, J. A. Schuller, R. E. Schaak, M. Terrones and J. A. Robinson, ACS Nano, 2015, 9, 11509–11539 CrossRef PubMed.
- Z. H. Zhang, X. L. Zou, V. H. Crespi and B. I. Yakobson, ACS Nano, 2013, 7, 10475–10481 CrossRef PubMed.
- J. Guan, G. T. Yu, X. L. Ding, W. Chen, Z. M. Shi, X. R. Huang and C. C. Sun, ChemPhysChem, 2013, 14, 2841–2852 CrossRef PubMed.
- Y. Tong, Y. Guo, K. Mu, H. Shan, J. Dai, Y. Liu, Z. Sun, A. Zhao, X. C. Zeng, C. Wu and Y. Xie, Adv. Mater., 2017, 29, 1703123 CrossRef PubMed.
- Y. Zhou, Z. Wang, P. Yang, X. Zu, Y. Li, X. Sun and F. Gao, ACS Nano, 2012, 6, 9727–9736 CrossRef PubMed.
- G. Y. Gao, G. Q. Ding, J. Li, K. L. Yao, M. H. Wu and M. C. Qian, Nanoscale, 2016, 8, 8986–8994 RSC.
- S. Z. Zhu and T. Li, Phys. Rev. B: Condens. Matter Mater. Phys., 2016, 93, 115401 CrossRef.
- X. Li, X. Wu and J. Yang, J. Am. Chem. Soc., 2014, 136, 11065 CrossRef PubMed.
- N. Miao, B. Xu, N. C. Bristowe, J. Zhou and Z. Sun, J. Am. Chem. Soc., 2017, 139, 11125–11131 CrossRef PubMed.
- B. Li, T. Xing, M. Zhong, L. Huang, N. Lei, J. Zhang, J. Li and Z. Wei, Nat. Commun., 2017, 8, 1958 CrossRef PubMed.
- K. W. Lee and C. E. Lee, Adv. Mater., 2012, 24, 2019–2023 CrossRef PubMed.
- M. Bonilla, S. Kolekar, Y. Ma, H. C. Diaz, V. Kalappattil, R. Das, T. Eggers, H. R. Gutierrez, M. H. Phan and M. Batzill, Nat. Nanotechnol., 2018, 13, 289–293 CrossRef PubMed.
- D. J. O'Hara, T. Zhu, A. H. Trout, A. S. Ahmed, Y. K. Luo, C. H. Lee, M. R. Brenner, S. Rajan, J. A. Gupta, D. W. McComb and R. K. Kawakami, Nano Lett., 2018, 18, 3125–3131 CrossRef PubMed.
- Y. P. Feng, L. Shen, M. Yang, A. Wang, M. Zeng, Q. Wu, S. Chintalapati and C. R. Chang, Wiley Interdiscip. Rev.: Comput. Mol. Sci., 2017, 7, e1313 Search PubMed.
- C. Gong, L. Li, Z. Li, H. Ji, A. Stern, Y. Xia, T. Cao, W. Bao, C. Wang, Y. Wang, Z. Q. Qiu, R. J. Cava, S. G. Louie, J. Xia and X. Zhang, Nature, 2017, 546, 265–269 CrossRef PubMed.
- W. Xing, Y. Chen, P. M. Odenthal, X. Zhang, W. Yuan, T. Su, Q. Song, T. Wang, J. Zhong, S. Jia, X. C. Xie, Y. Li and W. Han, 2D Mater., 2017, 4, 024009 CrossRef.
- B. Huang, G. Clark, E. Navarro-Moratalla, D. R. Klein, R. Cheng, K. L. Seyler, D. Zhong, E. Schmidgall, M. A. McGuire, D. H. Cobden, W. Yao, D. Xiao, P. Jarillo-Herrero and X. Xu, Nature, 2017, 546, 270–273 CrossRef PubMed.
- M. Ashton, D. Gluhovic, S. B. Sinnott, J. Guo, D. A. Stewart and R. G. Hennig, Nano Lett., 2017, 17, 5251–5257 CrossRef PubMed.
- J. Liu, Q. Sun, Y. Kawazoe and P. Jena, Phys. Chem. Chem. Phys., 2016, 18, 8774–8884 Search PubMed.
- L. Zhou, L. Kou, Y. Sun, C. Felser, F. Hu, G. Shan, S. C. Smith, B. Yan and T. Frauenheim, Nano Lett., 2015, 15, 7867–7872 CrossRef PubMed.
- W. B. Zhang, Q. Qu, P. Zhu and C. H. Lam, J. Mater. Chem. C, 2015, 3, 12457–12468 RSC.
- E. Torun, H. Sahin, S. K. Singh and F. M. Peeters, Appl. Phys. Lett., 2015, 106, 192404 CrossRef.
- M. A. McGuire, H. Dixit, V. R. Cooper and B. C. Sales, Chem. Mater., 2015, 27, 612–620 CrossRef.
- J. He, X. Li, P. Lyu and P. Nachtigall, Nanoscale, 2017, 9, 2246–2252 RSC.
- V. V. Kulish and W. Huang, J. Mater. Chem. C, 2017, 5, 8734–8741 RSC.
- K. R. Poeppelmeier and J. D. Corbett, Inorg. Chem., 1977, 16, 295 Search PubMed.
- J. F. Marchiando, B. N. Harmon. and S. H. Liu, Phys. B+C, 1980, 99, 259–263 CrossRef.
- J. D. Corbett, Acc. Chem. Res., 1981, 14, 239–246 CrossRef.
- K. S. Novoselov, D. Jiang, F. Schedin, T. J. Booth, V. V. Khotkevich, S. V. Morozov and A. K. Geim, Proc. Natl. Acad. Sci. U. S. A., 2005, 102, 10451–10453 CrossRef PubMed.
- R. Zacharia, H. Ulbricht and T. Hertel, Phys. Rev. B: Condens. Matter Mater. Phys., 2004, 69, 155406 CrossRef.
- J. Heyd, G. E. Scuseria and M. Ernzerhof, J. Chem. Phys., 2003, 118, 8207–8215 CrossRef.
- J. B. Goodenough, Phys. Rev., 1955, 100, 564–573 CrossRef.
- P. W. Anderson, Phys. Rev., 1959, 115, 2–13 CrossRef.
- J. Kanamori, J. Appl. Phys., 1960, 31, S14–S23 CrossRef.
-
J. P. Launay and M. Verdaguer, Electrons in Molecules: From Basic Principles to Molecular Electronics, Oxford University Press, New York, 1st edn, 2013 Search PubMed.
- W. X. Ji, B. M. Zhang, S. F. Zhang, C. W. Zhang, M. Ding, P. Li and P. J. Wang, J. Mater. Chem. C, 2017, 5, 8504–8508 RSC.
- W. Ji, B. Zhang, S. Zhang, C. Zhang, M. Ding, P. Wang and R. Zhang, Nanoscale, 2018, 10, 13645–13651 RSC.
- Y. Wan, Y. Sun, X. Wu and J. Yang, J. Phys. Chem. C, 2018, 122, 989–994 CrossRef.
- J. Zhou and Q. Sun, Nanoscale, 2014, 6, 328–333 RSC.
- T. Zhang, L. Zhu and G. Chen, J. Mater. Chem. C, 2016, 4, 10209–10214 RSC.
- A. S. Dhoot, C. Israel, X. Moya, N. D. Mathur and R. H. Friend, Phys. Rev. Lett., 2009, 102, 136402 CrossRef PubMed.
- H. Yuan, H. Shimotani, A. Tsukazaki, A. Ohtomo, M. Kawasaki and Y. Iwasa, Adv. Funct. Mater., 2009, 19, 1046–1053 CrossRef.
- J. T. Ye, S. Inoue, K. Kobayashi, Y. Kasahara, H. T. Yuan, H. Shimotani and Y. Iwasa, Nat. Mater., 2010, 9, 125–128 CrossRef PubMed.
Footnote |
† Electronic supplementary information (ESI) available: Computational methods; the structural and magnetic properties; the band structures of YCl and ZrCl monolayers; possible magnetic configuration; exchange-interaction constants; reliability test; electronic structure at hole doping concentration; band structures of ScCl bilayers; band structures with different Ueff values; relative energy of AFM and FM states under the variation of carrier concentration; formation energy of Sc-Cl bulk. See DOI: 10.1039/c8nh00101d |
|
This journal is © The Royal Society of Chemistry 2018 |