DOI:
10.1039/D4RA00775A
(Review Article)
RSC Adv., 2024,
14, 12676-12702
The untold story of starch as a catalyst for organic reactions†
Received
30th January 2024
, Accepted 4th April 2024
First published on 19th April 2024
Abstract
Starch is one of the members of the polysaccharide family. This biopolymer has shown many potential applications in different fields such as catalytic reactions, water treatment, packaging, and food industries. In recent years, using starch as a catalyst has attracted much attention. From a catalytic point of view, starch can be used in organic chemistry reactions as a catalyst or catalyst support. Reports show that as a catalyst, simple starch can promote many heterocyclic compound reactions. On the other hand, functionalized starch is not only capable of advancing the synthesis of heterocycles but also is a good candidate catalyst for other reactions including oxidation and coupling reactions. This review tries to provide a fair survey of published organic reactions which include using starch as a catalyst or a part of the main catalyst. Therefore, the other types of starch applications are not the subject of this review.
1. A little bit about starch
Starch or amylum is the second most abundant natural polysaccharide on earth in terms of quantity after cellulose.1 Traditionally, this biopolymer has been used in the food industry, however, thanks to the advancement of technology and increasing research, starch is not limited only to the food industry anymore, and there are many other industries like textiles and paper, chemicals, agriculture, medicine, and engineering industries that benefit starch and its applications.2
As the most common carbohydrate in the human diet3 and the main storing carbohydrate in many plants like vascular plants,4 this polymeric polyol can be found in many natural sources including grains (wheat, corn, rice, rye, barley, beans, peas, and lentil), fruit (banana peel, apple pulp, kiwi seeds, avocado, pineapple, and mango), plants (sorghum, cassava, potato, sweet potato, taro, amaranth, sago palm), and many other natural sources.1 Despite the numerous natural sources of starch, about 80% of the global starch production comes from Zea mays (corn).2
From a chemical point of view, starch (C6H10O5) is a white and tasteless powder that is insoluble in cold water, but if the water is heated beyond the boiling point in a closed container, it can be dissolved.5 This hydrocarbon polymer consists of α-D-glucopyranose units as monomers joined together through α-1,4-glycosidic bonds. Although the α-1,4-glycosidic bonds are the main way of connecting monomeric units and making the chain, there are other common bonds like α-1,6-glycosidic bonds which are responsible for making the connection between chains and creating branches.6
Starch is mainly made of two different types of molecules, i.e., amylose (linear and helical structure) and amylopectin (branched structure) (Fig. 1)7 that are responsible for about 20–30% and 70–80% of the natural starch composition, respectively.8 The chemical structure of starch makes this low-cost natural polymer a wonderful compound with many significant features including biodegradability, safe and convenient usage, annual renewability, and ease of availability.8 As a polyol, starch has many hydroxyl groups in its structure which can create a lot of intra and intermolecular hydrogen bonds. The presence of many hydroxyl groups makes starch suitable for many purposes. For example, starch can be converted into many valuable derivatives. Literature review9–11 shows that starch can undergo many reactions similar to alcohols including esterification,12,13 etherification,14,15 oxidation,16,17 cross-linking,18,19 sulfonation,20,21 and so on (Fig. 2). Also, the presence of glycosidic bonds in the structure of starch makes it susceptible to become hydrolyzed under alkaline, acidic, or enzymatic conditions.22–24
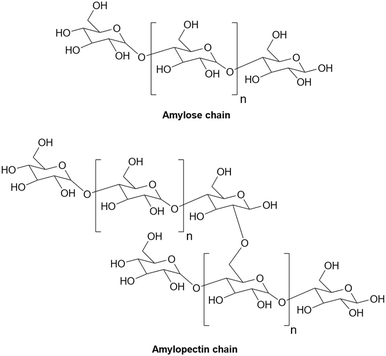 |
| Fig. 1 The chemical structure of two main components of starch. | |
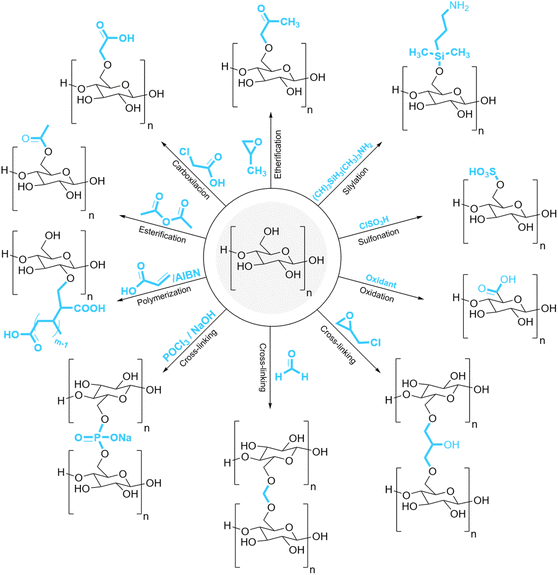 |
| Fig. 2 Some of the common modification reactions for starch.1,50 | |
In addition to transformations such as depolymerization and modification of the hydroxyl groups on the surface of starch, this polysaccharide can undergo more drastic changes to produce many diverse products (Fig. 3). For instance, the thermal dehydration of starch opens the doors to many carbon-based materials such as porous carbon materials, composite carbon materials, and carbon nanomaterials. Also, starch can be a substrate for many small organic molecules especially industrially manufactured products including 5-hydroxymethylfurfural, levulinic acid, lactic acid, acetone, butanol, ethanol, etc.7
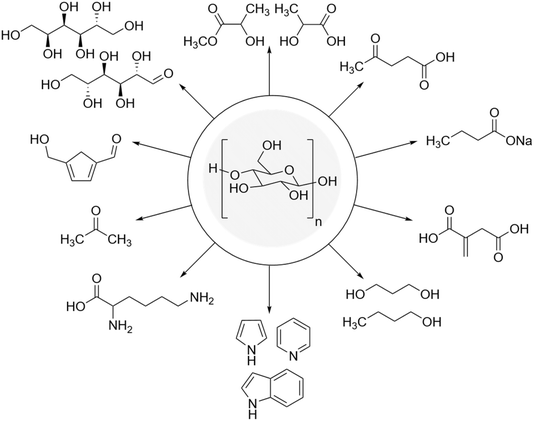 |
| Fig. 3 Some of the important compounds made from starch.7 | |
Apart from the importance of starch as a substrate for the preparation of valuable products, this natural polymer or its slightly modified derivatives can be used as great tools for other purposes.25–31 For example, starch with many hydroxyl groups can act like a molecular octopus with many tentacles to remove environmental pollutants such as heavy metals,32 dyes,33 pesticides,34 anti-biotics,35 and oils.36 Also, there are many reports about starch-based material application as biodegradable and renewable alternatives for current widely used non-renewable resins.37
Besides the application of starch and starch-based materials in the mentioned fields, using starch or modified starch as catalysts has attracted growing attention.38–40 Regarding the increasing number of published reports on the use of starch as a catalyst, it is necessary to write a review about the application of starch in organic reactions. To the best of my knowledge, there is no related review about the role of starch as a catalyst in organic reactions and this one would be the first of its kind. This review aims to comprehensively examine organic reactions that have been carried out using starch or its derivatives as catalysts and categorize them based on the type and structure of the employed starch.
2. Starch as catalyst for organic reactions
Depending on the type and application of the catalyst, there are different mentioned properties for a suitable catalyst in scientific literature. However, for a heterogenous catalyst, we can point out properties like cost, toxicity, stability and durability, volumetric efficiency (good surface area and available active sites), activity (promoting the desired reaction with good yield at a sensible temperature and pressure), selectivity, recoverability and reusability.41 As a heterogeneous candidate catalyst, starch can fulfill many properties expected of a suitable catalyst. For example, it is cheap, non-toxic, environmentally friendly, reusable, and easy to access and apply, making this biopolymer worth exploring for different organic reactions. Also, with many hydroxyl groups on the surface of this member of the carbohydrate family, it is possible to convert starch to desired functionalized derivatives that can even widen the application of starch as a catalyst.
Regarding the fact that there is no such thing as the perfect catalyst, it is not a big surprise that in addition to the many advantages of starch as a heterogeneous catalyst, there are also some disadvantages. Starch is a hygroscopic substance by nature, so it can absorb up to 17% of moisture.42 This means that at least naive starch can not be a good catalyst candidate for water-sensitive reactions. Moreover, naive or simple starch is not capable of coping with some conditions like high temperature, a wide range of pH, or freeze–thaw cycles.42 Starch swells when heated in water. As the temperature of heated starch in water reaches the “gelatinization temperature” which depends on the characteristics of its source, swollen starch loses its semi-crystalline structure. When the increasing temperature goes beyond that temperature, the structural integrity decreases. Eventually, this leads the starch structure to break down into its constituent molecules, including amylose, amylopectin, and some other minor components.43 These limitations combined with a natural tendency of naive starch for decomposition and brittleness make it not to be desirable for every reaction condition. However, chemical modification can overcome some of the limitations of simple starch and make it possible to use this inexpensive and environmentally friendly catalyst in reactions with different conditions.
As mentioned before, starch in simple or chemically modified forms can be used for the desired reaction as a catalyst or catalyst support. To describe the mechanism of the action of starch as a catalyst in organic transformations we should resort to non-covalent interactions (NCIs).44 For the simple starch, the structural hydrogen bonding interactions are the major NCIs that can promote the conversion of starting materials to the desired products. As a polyhydroxy compound, each ring in the structure of starch has three hydroxyl groups (at C2, C4, and C6) that can make hydrogen bondings with active sites on substrate molecules and facilitate their transformations to the related products (Fig. 4).45 For example, Arabpourian and Behbahani proposed an overall reaction mechanism for the synthesis of pyrrole using starch as a heterogeneous catalyst (Fig. 5).46 The authors have shown how starch hydrogen bonding guides the starting materials to the desired product. In the first step, the diketone's carbonyl group is activated by starch's OH-groups to obtain intermediate 1. This hydrogen bonding makes the carbonyl group ready to be attacked by an amine as a nucleophile to produce intermediate 2. The second carbonyl activated by starch hydrogen bonding experiences an intramolecular amine nucleophilic attack which results in intermediate 3. Finally, the desired product 4 is obtained after the dehydration of intermediate 3.
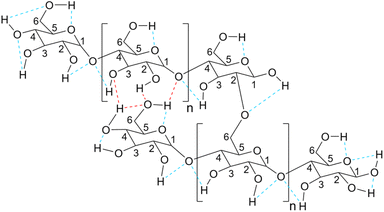 |
| Fig. 4 Intra- (blue) and intermolecular (red) hydrogen bonds in starch (amylopectin chain). | |
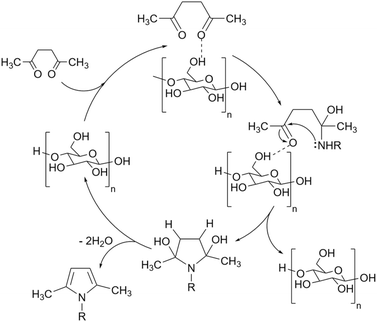 |
| Fig. 5 The proposed mechanism for the synthesis of N-substituted pyrroles using starch as a catalyst.46 | |
Similar to other polysaccharides,47 the hydroxyl groups on the surface of simple starch can be modified and attached to other functional groups to increase the ability of starch and accelerate organic reactions.48 For example, simple starch can be sulfonated using sulfonating agents (sulfuric acid, chlorosulfonic acid, and sulfur trioxide) to convert starch to a suitable brøsnted acid.21 As another instance, attaching amine groups to these hydroxyl groups by appropriate linkers to prepare related Schiff bases makes starch capable of being like molecular pincers holding metal ions as Lewis acid catalysts.49 Depending on the type of chemical functionalization on the surface of starch, the way that these modified starch can promote the reaction will be different but the important point is that the hydrogen bonds of starch with substrates are still considerable. This means when we have an acidic/basic or metal-loaded starch, even if the main driving force from the catalyst is coming from added parts (acid, base, or metal) to the starch, the effect of starch itself to promote the reaction still exists.
Based on published papers about using starch or modified starch as a catalyst for organic transformations, they can be placed in two categories: (1) unfunctionalized and (2) functionalized starch. The first type of starch is related to the ones with no surface functionalization which includes either simple starch or magnetic/composite starch. The second one indicates catalysts with chemical surface functionalization including acidic/basic, and metal-loaded starch. From the structural point of view, these two types of starch can have a simple or complex structure like a core–shell structure where starch is wrapped around a specific core usually a magnetic one, functionalized with desired groups (Fig. 6). In the following sections, this review deals with the reactions that employed simple and functionalized starch as a catalyst or catalyst support.
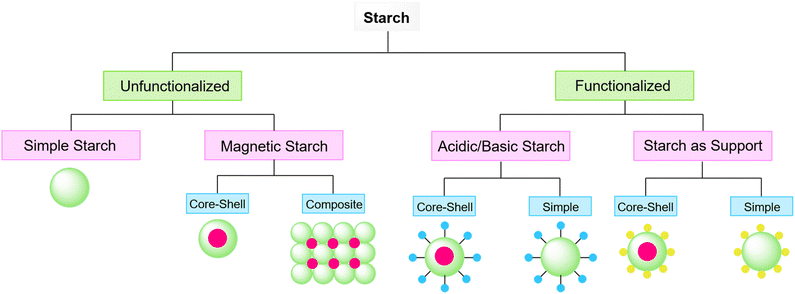 |
| Fig. 6 Different types of starch used as catalyst. | |
2.1 Simple starch
Simple or raw starch without any chemical modification could be used directly as a catalyst for the synthesis of many heterocyclic compounds. Published reports show that this cheap polysaccharide has been successfully used as a catalyst for the preparation of pyrrole, 2-aminothiazole, dihydropyranopyrazole, tetrahydrobenzopyran, and dihydropyranochromene derivatives. In the following, these reactions will be discussed.
2.1.1 Synthesis of pyrroles. Pyrroles are five-membered rings with many known applications in synthetic chemistry as an interesting scaffold for the construction of many valuable compounds.51,52 The most general approach for the synthesis of pyrroles is the Paal–Knorr reaction. Very recently, Arabpourian and Behbahani disclosed a novel method for the Paal–Knorr synthesis of different pyrroles.46 They successfully managed to prepare different 2,5-dimethyl-1-phenyl-1H-pyrrole derivatives from the reaction of 2,5-hexadione with a variety of amines at room temperature using simple starch as a catalyst (Scheme 1).
 |
| Scheme 1 Synthesis of N-aryl pyrroles using simple starch. | |
2.1.2 Synthesis of 2-aminothiazoles. Structures containing 2-aminothiazole moiety are an important class in the family of heterocyclic compounds and have many potential applications especially in medicinal chemistry because of their anti-cancer and anti-tumor, anti-viral, anti-inflammatory, and anti-microbial activities.53–55 Moreover, these compounds can be used as convenient substrates to prepare more complex products like corresponding azo derivatives56 and many polycyclic heterocyclic compounds.57,58 In 2017, our team at the University of Kashan found that simple starch can promote the synthesis of 2-aminothiazole derivatives in the presence of molecular iodine as a halogenating agent (Scheme 2).59 However, we soon found that using starch nanoparticles (starch NPs) instead of bulk starch increases the reaction yield by 1.5 times and also decreases the required starch for the reaction.
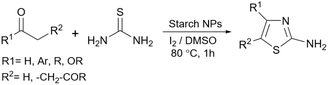 |
| Scheme 2 Synthesis of 2-aminothiazoles using starch nanoparticles. | |
In search for new capabilities of starch as catalyst in the preparation of 2-aminothiazole derivatives, two years later our group succeeded in finding a similar protocol to produce some diheteroaryl thioether derivatives containing 2-aminothiazole units (Scheme 3).60 Compared to our previous protocol for the synthesis of 2-aminothiazoles, this one proceeded at a much slower speed and a lower temperature.
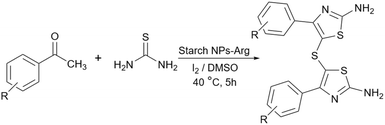 |
| Scheme 3 Synthesis of diheteroaryl thioethers using starch nanoparticles. | |
2.1.3 Synthesis of dihydropyranopyrazoles. As one of the significant fused heterocyclic compounds, dihydropyranopyrazoles have diverse bioactivities including anti-inflammatory, anti-cancer, anti-oxidant, analgesic, and anti-microbial properties.61 In 2015, Kangani and colleagues used starch solution to prepare a wide range of dihydropyrano[2,3-c]pyrazole derivatives from aryl aldehydes, hydrazine monohydrate, ethyl acetoacetate, and malononitrile as precursors (Scheme 4).62 To prepare the starch solution, they added starch to the magnetically stirred water, and after 30 minutes the achieved solution was filtered out and used as a solution containing starch.
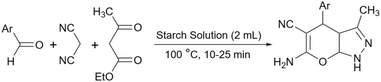 |
| Scheme 4 Synthesis of dihydropyrano[2,3-c]pyrazoles using starch solution. | |
2.1.4 Synthesis of tetrahydrobenzopyrans and dihydropyranochromenes. Tetrahydrobenzopyrans and dihydropyranochromenes not only have similar structures but also have many pharmaceutical and biological activities. For instance, prepared tetrahydrobenzo compounds can be used as an effective anti-cancer, anti-coagulant, diuretic, spasmolytic, and anti-anaphylactic agents63 and dihydropyrano[c]chromenes are similarly known for their anti-coagulant, anti-cancer, anti-bacterial, anti-microbial, anti-alzheimer, and anti-malaria properties.64 Hazeri et al. succeeded in achieving a protocol for the synthesis of both tetrahydrobenzo[b]pyrans and 3,4-dihydropyrano[c]chromenes using starch solution as a catalyst (Scheme 5).65 They prepared the starch solution as mentioned before62 and carried out these two three-component reactions at 50 °C with a very good yield for the products.
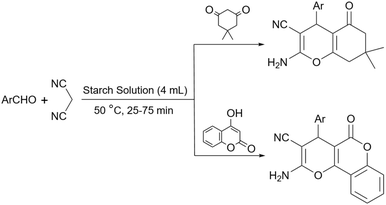 |
| Scheme 5 Synthesis of tetrahydrobenzo[b]pyrans and 3,4-dihydropyrano[c]chromenes using starch solution. | |
2.2 Magnetic starch
The recovery of the catalyst after the end of the reaction is one of the important problems with using simple starch as a catalyst in organic reactions. However, there are some solutions to fix this weakness. One of them is magnetizing the simple starch using iron oxide particles. The papers that used this technique will be presented, in the next sections.
2.2.1 Synthesis of pyran. As an oxygen-containing heterocyclic compound, pyrans are a known family of organic compounds with great importance in synthetic chemistry.66,67 Among the members of this family, fused benzo 4H-pyrans like 4H-chromene derivatives have been well-known because they are extensively present in nature including plants, vegetables, and fruits.68 In 2020, Kamalzare and colleagues introduced a novel starch composite for the synthesis of the 4H-pyran derivatives (Scheme 6).69 They prepared CuFe2O4-starch as a magnetically recyclable nanocatalyst and used it to facilitate a three-component reaction between aldehydes, malononitrile, and dimedone or ethyl acetoacetate (as C–H activated acidic compounds). The authors employed a simple dispersion technique to impregnate the starch with CuFe2O4. The resulting mixture was stirred for 8 hours and then dried on a glass plate for the next 48 hours to form the desired CuFe2O4-starch nanocomposite.
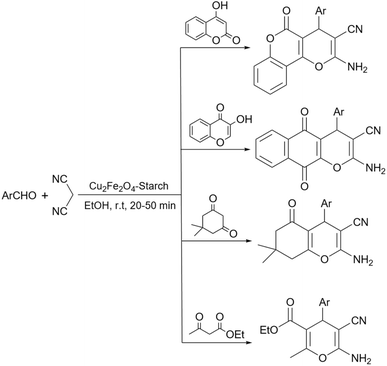 |
| Scheme 6 4H-Pyran synthesis catalyzed by CuFe2O4@starch. | |
2.2.2 Synthesis of chromenoquinolines. Pyranopyazoles, pyranopyrimidines, and pyrazolopyranopyramidines scaffolds can be found in the structure of many biologically active compounds.70,71 Recently, the Ameta research group successfully managed to prepare different pyrazolopyranopyrimidines from ethyl acetoacetate, hydrazine hydrate, barbituric acid, and various benzaldehydes as substrates (Scheme 7).72 To proceed with the reaction they used Fe3O4@starch as catalyst. Based on the proposed mechanism proposed by the authors, the reaction is facilitated by the hydrogen bond between substrates and the hydroxyl groups on the surface of the catalyst.
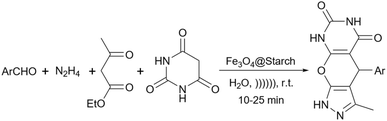 |
| Scheme 7 Chromenoquinoline synthesis catalyzed by Fe3O4@starch. | |
2.2.3 Synthesis of bis-coumarin and xanthenes. In 2018, Razikazemi et al. reported a procedure for the synthesis of bis-coumarin and xanthenes derivatives from 4-hydroxycoumarin and dimedone, respectively (Scheme 8).73 They prepared a magnetic starch from Fe3O4 and hot-water-soluble starch and used this nanocomposite as the catalyst. To prepare hot-water-soluble starch, they used a previously reported method74 including heating wheat starch with ethanol at 98 °C, centrifuging the achieved solution, and drying the prepared solid. The authors proposed that both reactions can proceed through a consecutive proton exchange on the surface of starch.
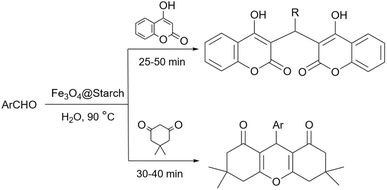 |
| Scheme 8 Synthesis of bis-coumarins and xanthenes using Fe3O4@starch. | |
2.2.4 Synthesis of 4H-pyran and tetrahydro-4H-chromene. Similar work to Hazeri's research65 has been done by Marjani and colleagues in 2020 (Scheme 9). They prepared Ag/Fe3O4@starch and used that as a catalyst for the synthesis of 4H-pyran and tetrahydro-4H-chromene derivatives.75 Authors reported that their magnetic nanocatalyst can be still reused even after up to five runs.
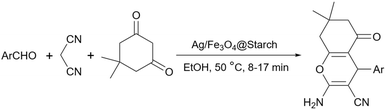 |
| Scheme 9 Synthesis of tetrahydro-4H-chromene catalyzed by Ag/Fe3O4@starch. | |
2.2.5 Synthesis of tetraketones and pyranopyrazoles. Tetraketones are important precursors for the preparation of many organic molecules including heterocyclic compounds. Also, these compounds have shown interesting biological activities including lipoxygenase and tyrosinase-inhibiting properties.76 Very recently, Matloubi Moghaddam and colleagues introduced a method for the preparation of magnetic starch and using it as a catalyst for the synthesis of tetraketone and pyranopyrazole derivatives (Scheme 10).77 They used bread waste as the source of starch and then modified the prepared starch with CoFe2O4 nanoparticles.
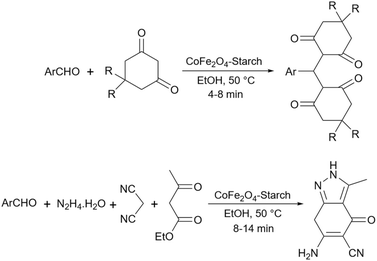 |
| Scheme 10 Synthesis of tetraketones and pyranopyrazoles using CoFe2O4-starch nanocomposite. | |
2.3 Acidic starch
Simple starch has many hydroxyl groups and is capable of helping the reaction process through hydrogen bondings with substrates and intermediates, but sometimes these groups are not effective enough to help the reaction. Therefore, the functionalization of simple starch by acidic groups is used to increase the ability of starch to create more hydrogen bonds and the activation of substrates. The related papers will be discussed in the coming parts.
2.3.1 Synthesis of coumarin. Coumarin derivatives similar to many other heterocyclic compounds have shown interesting pharmacological properties. Based on published research papers, this family that belongs to lactones shows significant properties like anti-fungal, anti-coagulant, insecticidal, and anti-helminthic activities, HIV protease inhibition, and even some potential applications in the treatment of Alzheimer's disease.78To prepare bis-coumarins commonly known as coumarin derivatives (Scheme 11), Rezaei and Sheikhi used starch-sulfuric acid as a catalyst. Instead of modifying starch with linkers and attaching the sulfonate group to the linker,79 they just synthesized starch-sulfuric acid by adding chlorosulfonic acid to the starch in n-hexane as solvent at 0 °C (Scheme 12).80 In their provided protocol, the transformation of aldehydes and coumarins as substrates to the corresponding bis-coumarin derivatives involves a Knoevenagel reaction followed by a Michael addition which both reactions can be facilitated by Brønsted acids like sulfuric acid.
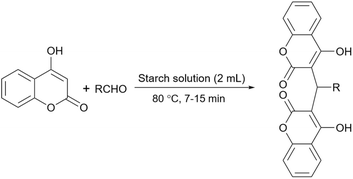 |
| Scheme 11 Synthesis of bis-coumarin using starch-sulfuric acid. | |
 |
| Scheme 12 Preparation of starch-sulfuric acid. | |
2.3.2 Synthesis of pyrazoles. Studies show that pyrazoles could stop many cancer cell types from developing including lung, brain, blood, prostate, colorectal, renal, and pancreatic cancer.81 In 2013, Hatamjafari published a paper on Helvetica Chimica Acta reporting a new method for the synthesis of 1,5-diaryl-1H-pyrazoles catalyzed by starch-sulfuric acid (Scheme 13).82 The provided method was based on the condensation of Baylis–Hillman adducts of an α,β-unsaturated, and phenyl hydrazine as substrates. Two years later, Vekariya and his co-workers used the same catalyst to speed up a 4-component reaction to prepare related 1,4-dihydropyranopyrazoles (Scheme 14).83 They came up with an efficient way to convert ethyl acetoacetate, hydrazine, benzaldehyde, and malononitrile nitrile to the corresponding 1,4-dihydropyrano[2,3-c]-pyrazole-5-carbonitriles in less than 30 minutes with pretty good yields.
 |
| Scheme 13 Synthesis of the 1,5-diaryl-1H-pyrazoles using starch-sulfuric acid. | |
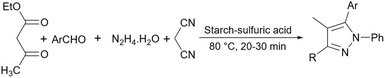 |
| Scheme 14 Synthesis of 1,4-dihydropyrano[2,3-c]-pyrazole-5-carbonitriles using starch-sulfuric acid. | |
2.3.3 Synthesis of phthalazines. Phthalazines, benzopyridazines, or benzo-orthodiazines are members of heterocyclic families that are present in many natural products with extensive proven or potential biological applications like anti-cancer activity.84 With this in mind, in 2011, Shaterian and Rigi disclosed another application for starch-sulfuric acid as a novel catalyst for the preparation of 2H-indazolo[2,1-b]phthalazine-trione derivatives (Scheme 15).85 Their presented four-component reaction included hydrazine, phthalic anhydride, dimedone, and benzaldehyde as substrates.
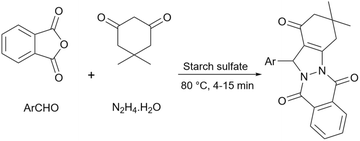 |
| Scheme 15 Synthesis of 2H-indazolo[2,1-b]phthalazine-triones using starch-sulfuric acid. | |
2.3.4 Synthesis of xanthene. Compounds containing xanthene and benzoxanthene units have shown many diverse properties like anti-bacterial and anti-inflammatory activities, the ability to be used as fluorescent dyes, and promising applications in laser technology.86–88 In 2015, Shaterian and Rigi unveiled a new method for the synthesis of xanthene and quinazoline derivatives (Scheme 16).89 Apart from different precursors, the presented protocols for the two reactions were almost similar including using starch sulfate as a biodegradable solid acid.
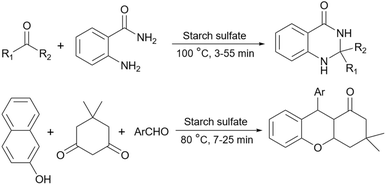 |
| Scheme 16 Synthesis of xanthene and quinazoline derivatives using starch-sulfuric acid. | |
In 2017, our team at the University of Kashan proposed an effective procedure for the preparation of 14-aryl-14H-dibenzo[a,j]xanthenes using sulfonated starch nanoparticles as a promising heterogeneous catalyst (Scheme 17).90 The reaction proceeded in a solvent-free condition at 110 °C with satisfactory yields for a wide range of substrates. To prepare starch nanoparticles, the bulk starch went through a two-step process. In the first step starch was treated with acid and then base under an argon atmosphere and rigorous stirring. Then, potassium persulfate was added to the resulting mixture, and in the end, the starch nanoparticle was separated from the solution using an ice bath.
 |
| Scheme 17 Synthesis of 14-aryl-14H-dibenzo[a,i]xanthenes using sulfonated starch nanoparticles. | |
2.3.5 Synthesis of dihydropyrimidinone. Since Biginelli's first report about the condensation of dicarbonyl compounds with aldehydes and urea or thiourea for the synthesis of dihydropyrimidinones in 1893,91 there have been many reports about these important compounds in medicinal chemistry.92 In 2013, Rezaei et al. disclosed a novel catalytic method for the preparation of these compounds using starch-sulfuric acid as a catalyst (Scheme 18). They used their modified starch as an eco-friendly and reusable catalyst for the famous Biginelli reaction.93
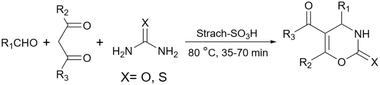 |
| Scheme 18 Synthesis of dihydropyrimidinones using starch sulfuric acid. | |
2.3.6 Synthesis of quinolines. Quinoline or benzopyridine is one the most important N-based heterocyclic compounds with many medicinal, bioorganic, and industrial chemistry applications.94 Shaabani and co-workers proposed a sulfonated starch-catalyzed Friedländer reaction for the synthesis of quinoline derivatives using 2-amino aryl ketones and β-diketones as substrates (Scheme 19).95 They also prepared and used sulfonated cellulose for this reaction, but no significant difference between the catalytic effects of cellulose sulfuric acid and starch sulfuric acid was observed.
 |
| Scheme 19 Synthesis of quinolines using starch sulfuric acid. | |
2.3.7 Synthesis of quinoxalines and benzimidazoles. Quinoxalines and benzimidazoles like many other nitrogen-containing heterocycles have a great place in synthetic and medicinal chemistry. Literature review shows that there are many reports about the synthesis of quinoxalines96,97 and benzimidazole derivatives.98,99 For instance, in 2018, Farrokhi and co-workers disclosed a new method for the synthesis of quinoxalines and benzimidazoles using Fe2O3@starch-1,4-piperazinediylbis(methylene)bisphosphonic acid as Brønsted acid (Scheme 20).100
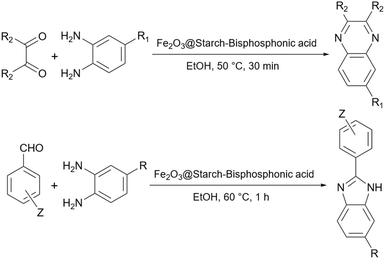 |
| Scheme 20 Synthesis of quinoxalines and benzimidazoles using Fe2O3@starch-1,4-piperazinediylbis (methylene) bisphosphonic acid. | |
2.3.8 Synthesis of chromenoquinoline. Quinolines and chromenes are considered important families in heterocyclic chemistry. However, the literature review shows that chromenoquinoline compounds that contain both these units at their structure have many interesting properties.68 For example, some of these compounds have shown the ability to act like fluorescence sensors.101 Recently, Sayahi et al. reported a sulfonic acid functionalized magnetic starch as a novel and efficient catalyst for the synthesis of chromeno[4,3-b]quinoline-6,8(9H)-dione derivatives (Scheme 21).102 To prepare the catalyst they prepared Fe3O4 nanoparticles, modified its surface with tetraethyl orthosilicate (TEOS), and attached these nanoparticles to the surface of starch. In the next step, the surface of the prepared magnetic starch was coated with allyltrimethoxysilane. Finally, the 4-styrenesulfonic acid was attached to the surface as the last layer through polymerization (Scheme 22).
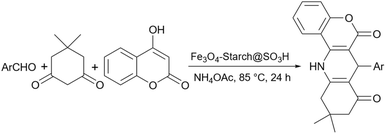 |
| Scheme 21 Synthesis of chromenoquinoline using Fe3O4-starch@SO3H. | |
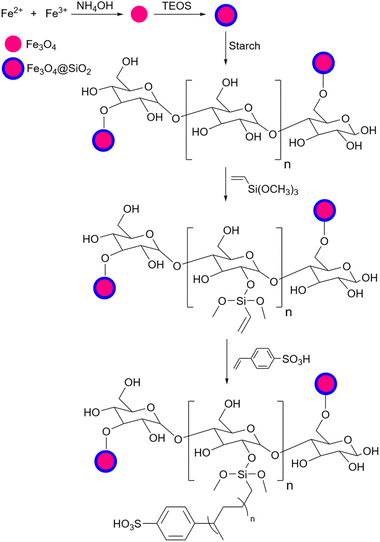 |
| Scheme 22 Preparation of Fe3O4-starch@SO3H. | |
2.3.9 Synthesis of formamides and acetamides. Converting primary and secondary amines to their related formamide and acetamide products has always been interesting to chemists.103,104 This is because amide bonds are a significant part of many compounds including some important polymers, drugs, dyes, and agrochemical compounds.105–108 Very recently, Wani et al. employed sulfated starch as an efficient catalyst to transform the simple amines to their corresponding amides (Scheme 23).109
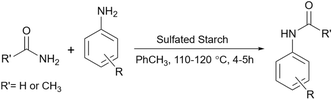 |
| Scheme 23 Synthesis of formamides and acetamides using sulfated starch. | |
2.4 Basic starch
Although simple starch can act like a Brønsted acid to advance the reaction this is not very helpful for those reactions that need basic catalysts. To use starch as a catalyst for these types of reactions, researchers have used basic agents including NaOH, and p-phenylenediamine (PPD) to give basic properties to the starch. The papers in which basic starch has been used as a catalyst will be presented in the next sections.
2.4.1 Synthesis of hydroquinazolinones. Quinazolinones are interesting heterocyclic compounds with a wide range of biological and pharmaceutical activities.110,111 For example, there are many drugs containing quinazolinones such as quinethazone, metolazone, raltitrexed, and fenquizone.112 Tamaddon and co-workers reported two protocols for the synthesis of dihydroquinazolines using alkaline starch as a catalyst (Scheme 24).113,114 In their reports, they used 2-aminobenzonitrile, 2-aminobenzamide, and isatoic anhydride as precursors. To prepare alkaline starch, they ground corn starch with NaOH to achieve a yellowish powder. The achieved powder was washed with ethanol, dried in a vacuum oven, and used in the mentioned reactions.
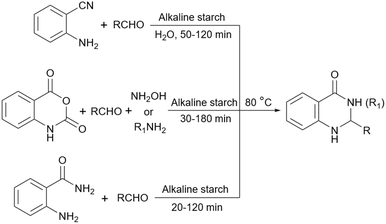 |
| Scheme 24 Synthesis of hydroquinazolinones using alkaline starch. | |
2.4.2 Synthesis of pyrazolones. Like many heterocyclic compounds, there are many reports about pyrazolones as useful compounds.115,116 For example, there is a wide range of FDA-approved drugs containing pyrazolone nucleus including edaravone, matamizole, nifenazone, and eltrombopag.117 Very recently, Ramshini and his co-workers published a paper about using magnetic aminated starch as a suitable catalyst for the synthesis of 4-[(indol-3-yl)-arylmethyl]-1-phenyl-3-methyl-5-pyrazolone derivatives (Scheme 25).118 The authors used Fe3O4-starch-p-phenylenediamine nanocomposites to convert indole, 1-phenyl-1H-pyrazol-5-ol, and different aldehydes to the related pyrazolones. To prepare the catalyst, the researcher converted the simple starch to the tosylated starch and then used p-phenylenediamine as a nucleophile to substitute the tosylate groups. Finally, the intended catalyst was prepared using the precipitation of iron nanoparticles onto the aminated starch prepared from the last step (Scheme 26).
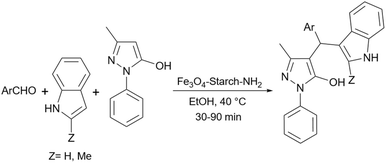 |
| Scheme 25 Synthesis of diheteroaryl thioethers using starch NPs-arginine. | |
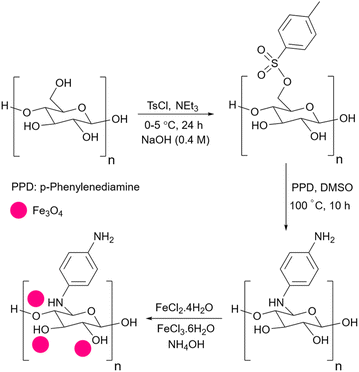 |
| Scheme 26 Preparation of magnetic aminated starch nanocomposite. | |
2.5 Starch as support
As mentioned in previous sections, simple starch can be used for many organic reactions, however, there are still some reactions including coupling, reduction, and oxidation, and some heterocyclic reactions that simple starch can not cope with. For this reaction, researchers have used starch as catalyst support to help the catalyst increase the reaction yield of the reaction. These catalytic systems will be introduced in the coming parts.
2.5.1 Synthesis of azines. Very recently, our group reported another starch-based structure as a catalyst for organic reaction.119 We successfully converted some aldehydes and hydrazine sulfate as substrates to the desired azines using starch nanoparticles modified with 1-propyl-3-methylimidazolium chloride ([PMIM]Cl) as catalysts (Scheme 27). The yield of products was satisfying and the reaction proceeded well at room temperature. To prepare the catalyst, first, we created N-methyl-N-propyltriethoxysilane imidazolium chloride as an ionic liquid (IL) part from the reaction between methyl imidazole and 3-chloropropyltriethoxysilane under reflux conditions. Then, we attached the prepared ionic liquid from the last step to the surface of the starch to reach the starch-IL NPs under reflux conditions in ethanol as solvent (Scheme 28).
 |
| Scheme 27 Synthesis of diheteroaryl thioethers using starch-IL NPs. | |
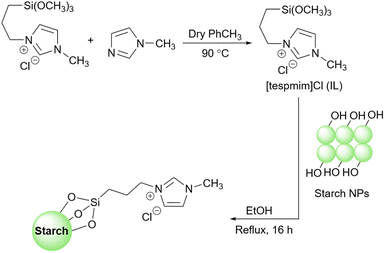 |
| Scheme 28 Preparation of starch-IL NPs. | |
2.5.2 Synthesis of diheteroaryl thioethers. After proving the capabilities of starch nanoparticles as good catalysts for the synthesis of heteroaryl thioethers,60 our group screened some functionalized starch nanoparticles to find another suitable catalyst for this interesting disulfide product. In the same year, we unveiled another similar protocol based on using arginine-decorated starch nanoparticles as a novel catalyst for the same reaction (Scheme 29).120 We used 3-chloropropyltrimethoxysilane as a linker to connect arginine amino acid to the surface of starch nanoparticles (Scheme 30).
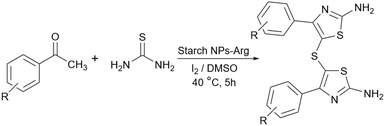 |
| Scheme 29 Synthesis of diheteroaryl thioethers using starch NPs-arginine. | |
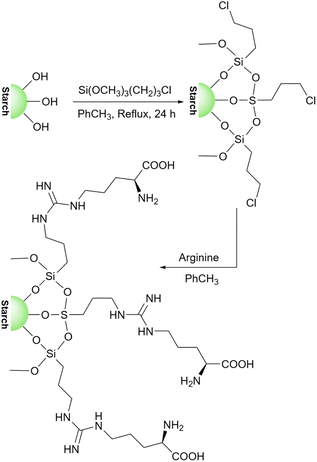 |
| Scheme 30 The steps for the synthesis of arginine-nanostarch. | |
2.5.3 Synthesis of triazoles. Triazoles(1,2,3-triazole and 1,2,4-triazole) and their derivatives are well-known compounds in synthetic chemistry.121 Many important properties have been attributed to these compounds such as anti-viral, anti-microbial, anti-cancer, anti-depressant, analgesic, and anti-inflammatory activities.122 To the best of my knowledge, there are three published papers about the synthesis of triazoles using starch as catalyst support. As the first one, Pourjavadi et al. published copper 1,2,3-triazoles synthesis using a magnetic starch nanocomposite (Scheme 31).123 The results were good and they managed to reach good to excellent yields. To prepare the catalyst, they synthesized silica-coated Fe3O4 nanoparticles as the core of the catalyst and functionalized its surface by 3-(trimethoxysilyl)propylmethacrylate (MPS). Then, alongside starch, a mixture of acrylamide and N,N-methylenebisacrylamide was used to provide a Fe3O4@SiO2@cross-linked polymeric starch nanocomposite. Finally, CuCl2 was added to the prepared magnetic starch to provide the intended Fe3O4@SiO2@starch-CuCl2.
 |
| Scheme 31 Synthesis of triazoles using Fe3O4@SiO2@MNP@starch-CuCl2. | |
In 2018, Bonyasi et al. proposed starch@CuFe2O4 as a magnetic reusable catalyst for the synthesis of 1,2,3-triazoles from benzyl bromide, sodium azide, and phenylacetylene (Scheme 32).124 To attach the prepared CuFe2O4 nanoparticles to the surface of starch, they functionalized the nanoparticles and starch using (3-chloropropyl)triethoxysilane and (3-mercaptopropyl)triethoxysilane, respectively (Scheme 33).
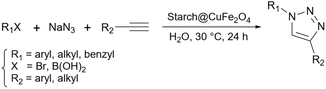 |
| Scheme 32 4H-Pyran synthesis catalyzed by starch@CuFe2O4. | |
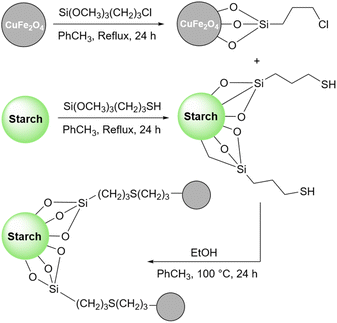 |
| Scheme 33 Steps for the preparation of starch@CuFe2O4. | |
Inspired by previous work, very recently, Gupta et al. used surface functionalized starch with copper(II) acetate as a heterogeneous catalyst to promote the synthesis of 1,4-disubstituted-1,2,3-triazoles (Scheme 34).125 To stabilize the copper salt on the surface of the starch, they modified the surface of the starch with a Schiff base. To do this, first, they attached a 3-aminopropyl(trimethoxy)silane molecule to the starch and then converted the amine group on this molecule to the corresponding imine by adding 2-acetylpyridine to the prepared 3-aminopropyl starch (Scheme 35). Based on the proposed mechanism by authors for the synthesis of triazoles, the key step in this transformation is the reduction of Cu(II) to Cu(I) which facilitates the connection between an azide and terminal alkyne substrates to produce the related triazole derivatives.
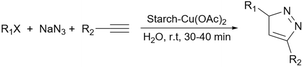 |
| Scheme 34 Synthesis of triazoles using starch-Cu(OAc)2. | |
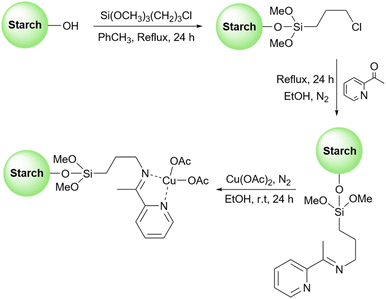 |
| Scheme 35 Steps for the preparation of modified starch@Schiff base-Cu(OAc)2. | |
2.5.4 Synthesis of aminotetrazoles. 5-Aminotetrazole derivatives have been used as important synthons in some multicomponent reactions to achieve more complex polycyclic compounds.126 Recently, Nasiri and co-workers published a paper about preparing and using Fe3O4-nanostarch@AgNPs as a catalyst for the synthesis of various arylaminotetrazole derivatives (Scheme 36).127 To prepare the starch nanoparticles, the authors employed an acidic hydrolysis method using H2SO4 as the acid. In addition to the synthesis of different 5-aminotetrazoles, they tested their catalyst for some other reactions including the oxidation of benzyl alcohol to benzaldehyde by hydrogen peroxide as oxidant and the reduction of 4-nitrophenol, Congo red, rhodamine B, and methylene blue using NaBH4 as reductant.
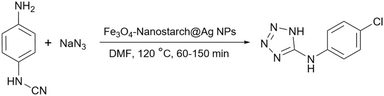 |
| Scheme 36 Synthesis of 5-arylamino-1H-tetrazole using Fe3O4-nanostarch@AgNPs. | |
2.5.5 Synthesis of allyl indoles. Because indoles are a pharmacologically important class of compounds, converting simple indoles to their more complex derivatives has attracted significant attention.128–131 Indole alkaloids are among the important indole derivatives that can be prepared by the alkylation reaction at the indole C3 position.132,133 In 2019, the Mallick research group developed a new protocol including using starch-supported cuprous iodide nanoparticles as catalysts for the 3-allylation reaction of N-substituted indoles (Scheme 37).134 The authors proposed that the reaction proceeds with an electrophilic mechanism assisted by CuI nanoparticles as a catalyst.
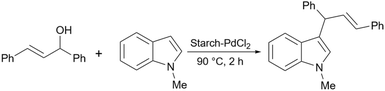 |
| Scheme 37 Synthesis of allyl indoles using alkaline starch-PdCl2. | |
2.5.6 Synthesis of indazoles. There are usually two tautomeric forms for indazoles: 1H-indazole and 2H-indazole. From a thermodynamical point of view, the first one is more stable (Fig. 7).135 Apart from stability, not only these compounds are prevalent in nature but also the synthetic compounds containing these structures have shown considerable biological properties.136 In 2014, Sodhi and colleagues presented a new starch-based catalyst for the synthesis of 2H-indazoles from the reaction between 2-bromo-benzaldehydes, various primary amines, and sodium azide.137 The catalyst they used was an amine-functionalized silica/starch-Cu(acac)n (Scheme 38). To prepare this catalyst, in the first step, they bound the starch to the surface of silica which was activated by SOCl2. Then, 3-aminopropyl(trimethoxy)silane is attached to the surface of starch and silica to prepare the support for the final step. Finally, amine-functionalized silica/starch was treated with M(acac)n to reach the desired amine-functionalized silica/starch-M(acac)n catalyst (Scheme 39). In addition to indazoles, the authors used this catalyst for the catalyzed thioetherification of different aryl halides using thiourea and the results were promising.
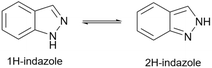 |
| Fig. 7 Two tautomeric forms of indazoles. | |
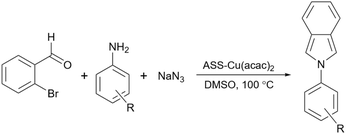 |
| Scheme 38 Synthesis of indazole derivatives using amine-functionalized silica/starch-Cu(acac)n. | |
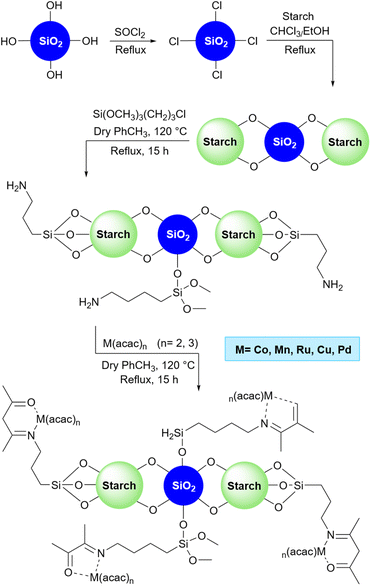 |
| Scheme 39 Preparation of amine-functionalized silica/starch-M(acac)n. | |
2.5.7 Synthesis of triarylmethane. The triarylmethane unit is an important structural part of triarylmethane dyes.138 Among synthetic methods, the Friedel–Crafts alkylation reaction is probably the most common method for the synthesis of triarylmethanes.139,140 In 2015, Rafiee and Khodayari reported a novel method for the Friedel–Crafts alkylation of benzhydrols substrates using starch-Fe3O4–H3PW12O40 composite as an efficient catalyst (Scheme 40).141
 |
| Scheme 40 Synthesis of triarylmethane using starch-Fe3O4-H3PW12O40. | |
2.5.8 Synthesis of 4-aminotoluenes. As one of the earliest research about using starch as a catalyst for the synthesis of aniline from nitrobenzene,142 one can refer to Liu's paper. His research group prepared and used a silica-supported starch-L-glutamic acid–Fe complex for the hydrogenation of nitrobenzene, cyclohexanone, and octene.143 After Liu's work about the hydrogenation of nitrobenzenes using silica-supported starch-glutamic acid–Fe as a catalyst, there have been more published papers about this reaction. For example, in 2017, Chairam and co-workers showed the reduction of 4-nitrophenol to 4-aminophenol using mung bean starch-Au nanoparticles and NaBH4 as the catalyst and reducing agent, respectively.144 In 2018, Luo's research group modified maize starch with 2-(dimethylamine)ethyl methacrylate and N,N′-methylenebisacrylamide by using a free radical polymerization process. Then, they used this modified starch as a support to precipitate gold nanoparticles. They employed the prepared catalyst for the hydrogenation reaction of p-nitro anisole (Scheme 41).145
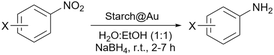 |
| Scheme 41 Synthesis of 4-aminotoluenes using starch@Au. | |
In 2019, Gholinejad and colleagues prepared starch support containing gold nanoparticles for the reduction of nitroarenes to their corresponding amines using NaBH4 as the reducing agent.146 To stabilize the gold nanoparticle on the surface of starch they modified the surface of starch with (3-chloropropyl)triethoxysilane so that it can act as a linker and hold the gold nanoparticles.
As another example of converting of nitroaromatic compounds to the corresponding amines, Nasiri et al. prepared and employed nanocomposites containing Fe3O4-starch-Cu nanoparticles as catalysts.147 The authors also tested their catalyst for the reduction of some dyes such as Congo red, rhodamine B, and methylene blue and the results were promising. Recently, Heravi's research group published another protocol for the hydrogenation of nitroaromatics using sulfur-doped graphitic carbon nitride decorated with starch, Fe3O4, and Ag.148 They managed to proceed with the reaction in aqueous media with excellent yield for many different nitrobenzene derivatives. Very recently, Lan et al. showed the potential catalytic activity of kaolin@chitosan-starch-Au for the reduction of nitrobenzene derivatives under mild conditions using sodium borohydride as a reductant.149
2.5.9 Synthesis of propargylamines. Propargylamines have many applications in synthetic and medicinal chemistry. For example, they are essential building blocks for the synthesis of many biologically active compounds and also natural products such as lactams, and peptides.150 To the best of my knowledge, there are only two reports about using starch as support for the synthesis of propargylamines. The first one belongs to Gholinejad's group (Scheme 42).151 In 2016, they reported copper nanoparticles supported starch microparticles as a catalyst for the synthesis of propargylamines from the reaction of benzaldehyde, phenylacetylene, and piperidine. To prepare the catalyst, the authors dissolved corn starch in distilled water and acetone to prepare the starch microparticles. Then, they separated high molecular weight starch, dissolved it in acidic water (pH = 2.5), and added acetone to the prepared solution. Finally, they added glutaraldehyde to the solution and after stirring for 12 hours, dried the achieved solution to reach the starch microparticles.
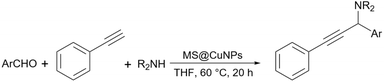 |
| Scheme 42 Synthesis of propargylamines using MS@CuNPs. | |
In the second report about using starch as support for the synthesis of propargylamines, Saadati et al. presented a modified starch with a linker to stabilize copper nanoparticles and use this copper-loaded support as a catalyst for the synthesis of propargylamine derivatives.152 Very recently, Tajbakhsh and co-workers provided a protocol for the synthesis of propargylamines through a one-pot coupling reaction using copper(II)-immobilized on starch-coated nanomagnetite (Scheme 43).153 Based on the optimization data reported by the authors, the simple starch can proceed with this reaction with 70% yield, an efficiency even better than simple Fe3O4. However, when Fe3O4@starch-Acr@Cu(II) was used as the catalyst, the yield of the reaction increased to 99% and the consuming time for the reaction to be complete decreased to about half an hour. The authors proposed a plausible mechanism in which Cu(II) is first coordinated with π electrons of acetylene to produce the acetylide-Cu(II) complex. In this condition, copper promotes the attack of acetylide on the reactive iminium intermediate to obtain the desired propargylamine.
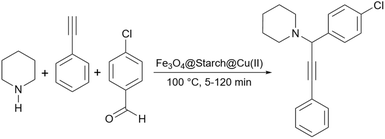 |
| Scheme 43 Synthesis of propargylamines using Fe3O4@starch-Acr@Cu(II). | |
2.5.10 Synthesis of 2,5-diformylfuran. 2,5-Diformylfurans, which can be prepared from different methods like the oxidation 5-hydroxymethylfurfural, have many potential applications in pharmaceuticals, organic conductors, and polymer chemistry.154 In 2019, Hong and co-workers prepared corn starch-anchored CuBr as a recyclable catalyst for the oxidation of 5-hydroxymethylfurfural to 2,5-diformylfuran (Scheme 44).155 To stabilize the CuBr on the surface of starch, first, they attached a 3-aminopropyltriethoxysilane to the surface of starch to obtain an amine-functionalized surface. Then, they added 2-pyridinecarboxaldehyde to the achieved amine from the previous step to make a Schiff base to anchor the CuBr particles (Scheme 45). Almost simultaneously with this report, they published another protocol for the oxidation of 5-(hydroxymethyl)furfural using a starch-supported catalyst.156 This time, they modified with 1-(triethoxysilyl)propyl-3-methylimidazolium chloride as an ionic liquid. Then, they used Al(NO3)3 as a metallic ion to stabilize the surface of the modified starch and employed the final product as a catalyst for the synthesis of 2,5-diformylfuran (Scheme 46).
 |
| Scheme 44 Synthesis of 2,5-diformylfuran using starch-CuBr. | |
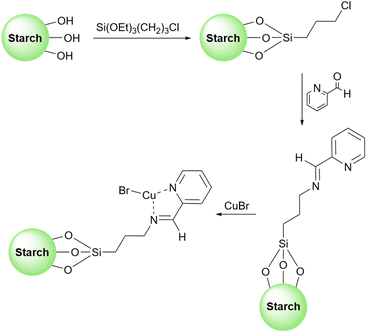 |
| Scheme 45 Synthesis of expanded starch@Schiff base-CuBr. | |
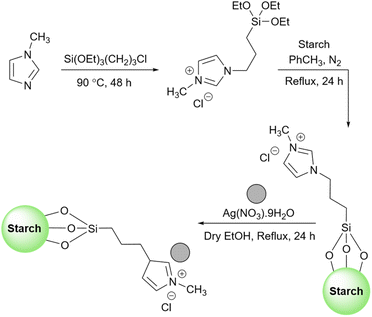 |
| Scheme 46 Synthesis of expanded starch-IL-Al(NO3)3 catalyst. | |
As mentioned earlier, 5-hydroxymethylfurfural (HMF) can be used as a precursor for the synthesis of 2,5-diformylfuran. However, the literature review shows using HMF as substrates is not always the case and there is a good number of reports about preparing HMF as the final product.157,158 One of these reports is about preparing 5-hydroxymethylfurfural using a starch-based catalyst. In 2017, Matharu et al. published a paper about the conversion of fructose to HMF using expanded starch@ionic liquid-FeCl3 as a catalyst.159 To expand the surface of starch, the authors used N,N′-disuccinimidyl carbonate and to attach iron chloride to the surface of expanded starch, they modified the surface of expanded starch with N-(3-aminopropyl), N-aryl imidazolium chloride.
2.5.11 Synthesis of imidazothiadiazoles. Imidazothiadiazoles and their derivatives have gained much attention in recent years, especially because of their wide biological activities.160–162 In 2019, Bahadorikhalili and colleagues provided a protocol for the preparation of magnetic starch as a support for ionic liquid-β-cyclodextrin (βCD-IL-starch-Fe3O4) (Scheme 47).163 They used this catalyst for the synthesis of imidazo[2,1-b][1,3,4]thiadiazol-5-amine derivatives. The authors proposed that the ionic liquid part of the catalyst has the main catalytic role in this transformation (Fig. 8).
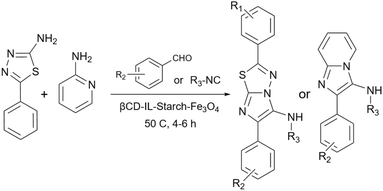 |
| Scheme 47 Synthesis of imidazothiadiazoles using βCD-IL-starch-Fe3O4 as a catalyst. | |
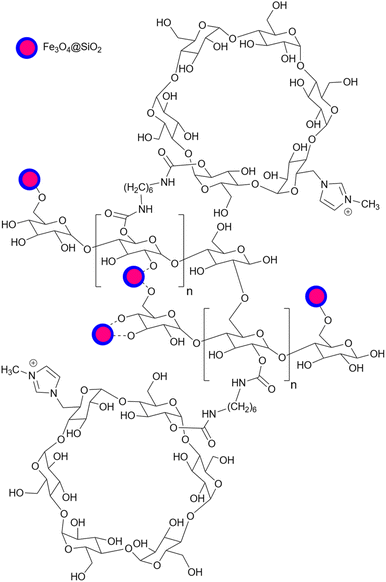 |
| Fig. 8 The proposed structure of βCD-IL-starch-Fe3O4. | |
2.5.12 Synthesis of dihydropyrimidine. Dihydropyrimidines are widely considered the “privileged structures”, some molecular frameworks with versatile binding properties.164 There are many biologically active pyrimidine-based compounds and alongside purine, pyrimidine is one of the building blocks of DNA and RNA.165,166 These structures can be found in several candidate drugs.167 To facilitate the preparation of these important heterocyclic compounds, Foroughifar's research group introduced magnetic starch support loaded with aspartic acid (Scheme 48).168 To prepare the mentioned catalyst, MnFeCaFe2O4@starch@aspartic acid, first, they prepared a MnFeCaFe2O4 as the core of the catalyst through the coprecipitation of related salts. To attach the starch to the surface of the prepared core, they activated MnFeCaFe2O4 by NH4OH and have the activated magnetic core stirred with starch in boiling water. To load the aspartic acid on the prepared magnetic starch support, the authors put the aspartic acid and the magnetic support under reflux conditions for 24 hours and dried the product in a vacuum (Scheme 49).
 |
| Scheme 48 Synthesis of dihydropyrimidine derivatives using MnFeCaFe2O4@starch@aspartic acid. | |
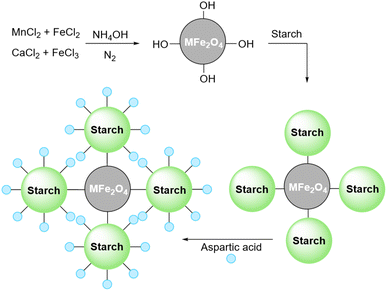 |
| Scheme 49 Preparation of MnFeCaFe2O4@starch@aspartic acid. | |
2.5.13 Synthesis of quinoxalines. As mentioned before, the synthesis of quinoxalines from 1,2-dicarbonyl and 1,2-diamine substrates can be catalyzed by acidic starch.100 However, 3 years before that research, the same research group had reported a paper about facilitating this reaction by a starch-Schiff base catalyst containing oxo zirconium (Scheme 50).169 Based on the table the authors provided to compare their work with previous research about using zirconium for the synthesis of quinoxaline, Fe3O4@starch-Schiff base-ZrOL2 was the best catalyst in terms of efficiency and the amount of required catalyst. The steps for the synthesis of the catalyst have been shown in Scheme 51.
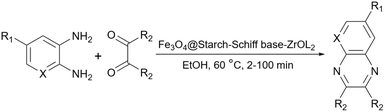 |
| Scheme 50 Synthesis of quinoxaline derivatives using Fe3O4@starch-Schiff base-ZrOL2. | |
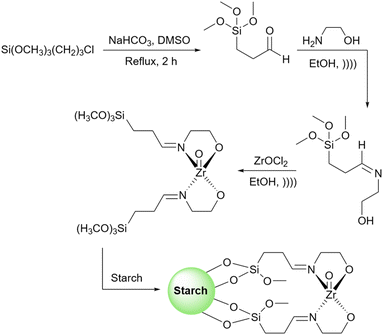 |
| Scheme 51 Preparation of MnFeCaFe2O4@starch@aspartic acid. | |
2.5.14 Synthesis of isocoumarins. Isocoumarins are a type of lactonic natural product that can be widely found in different parts of nature such as fungi, lichens, liverworts, bacteria, molds, higher plants, marine sponges, and even insect pheromones, and venom.170 Recently, Marandi and co-workers presented an indium-loaded Fe3O4@starch core–shell as a green biocatalyst for the synthesis of pyrazole-fused isocoumarins derivatives (Scheme 52).171 They used apple seeds as a cheap source of starch.
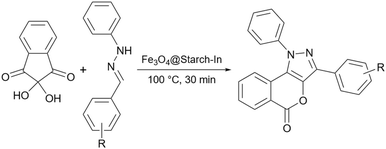 |
| Scheme 52 Synthesis of isocoumarin derivatives using Fe3O4@starch-In. | |
2.5.15 Hydration of terminal alkynes. The hydration of terminal alkynes is one of the common methods for preparing carbonyls. There are many published research papers about converting terminal alkynes to their related methyl carbonyl compounds.172–174 Recently, Mousavi-Mashhadi and Shiri successfully managed to prepare Fe3O4@starch-Au and use this core–shell structure as a heterogeneous catalyst for the hydration of terminal alkynes and nitriles (Scheme 53).175
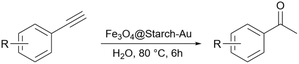 |
| Scheme 53 Synthesis of methyl carbonyls from terminal alkynes derivatives using Fe3O4@starch-Au. | |
2.5.16 Formylation of iodo benzene. As a greenhouse gas, the increase of environmental carbon dioxide is one of the main causes of global warming.176 With this fact in mind, the chemical fixation of carbon dioxide is an attractive research field.177–181 To provide another useful method to consume carbon dioxide, the research group of Jian came up with a new method to convert ido benzene to the corresponding benzaldehyde using Pd-nanoparticles grafted onto amino-functionalized nanostarch as a catalyst (Scheme 54).182 To prepare the catalyst, first, the author functionalized the surface of starch with 3-aminopropyltrimethoxysilane. Then, the Li2PdCl4 solution was added to the amino starch followed by adding NaBH4 to the mixture to convert Pd(II) to Pd(0) (Scheme 55).
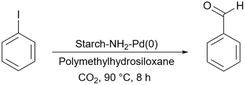 |
| Scheme 54 Synthesis of benzaldehyde from iodo benzene using starch-Pd(0). | |
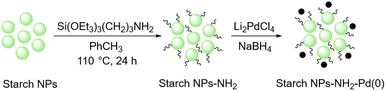 |
| Scheme 55 Preparation of starch-NH2-Pd(0). | |
2.5.17 Synthesis of pyranopyrimidinone and xanthene. Similar to xanthene, pyranopyrimidines have attracted much attention because of their application as anti-tumor, anti-bacterial, and anti-Alzheimer agents.183 In 2020, the Amininia research group presented a nano starch-ZnO composite as a novel heterogeneous catalyst for the synthesis of pyranopyrimidinone and xanthene derivatives under microwave irradiation (Scheme 56).184 In addition to the synthetic protocol, the authors tested prepared pyranopyrimidines and xanthene derivatives against M. luteus and P. aeruginosa and the results were promising. Very recently, Azad Poshtmakhi and colleagues reported another protocol for the synthesis of pyranopyrimidines using silver-loaded magnetic starch composite (Scheme 57).72 To prepare the catalyst they coated Fe3O4 nanoparticles with hot water starch solution (HWSS) and then decorated the product with Ag nanoparticles through heating it in a solution of Ag ion and glucose.
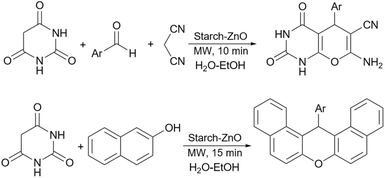 |
| Scheme 56 Synthesis of pyrano pyrimidinones and xanthenes using starch-ZnO composite. | |
 |
| Scheme 57 Synthesis of pyranopyrimidinones using Fe3O4@HWSS@Ag composite. | |
2.5.18 Coupling reactions. Coupling reactions are a class of organic transformations that allow two hydrocarbons to join together through the creation of a new C–C sigma bond. The reaction between an organic halide (R–X) and a main group organometallic compound (R′M) is the backbone of cross-coupling reactions. If R and R′ are the same this reaction is called a homo-coupling reaction and otherwise it is called a hetero-coupling reaction.185,186Biphenyl derivatives are one the most common products of coupling reactions. There are many synthetic methods for the synthesis of biphenyl compounds because of their synthetic and biological importance.187 Simple biphenyls are pretty non-reactive because they don't have any active functional group. However, their derivatives with active functional groups are more reactive, and because of this higher reactivity, they have a wide range of applications in different fields.188 Among synthetic protocols for coupling reactions, the Suzuki reaction is one of the famous methods for the synthesis of biphenyl compounds.189
In 2017, Baran introduced a starch-based palladium catalyst for the Suzuki coupling reactions (Scheme 58).190 His protocol includes making the connection between the surface of starch and 3-aminopropyltriethoxysilane (APTES) as an amine linker. Then, 2,4-dihydroxybenzaldehyde as an aldehyde agent can be added to the prepared starch-APTES to reach the desired Schiff base. Finally, adding Na2PdCl4 to the prepared Schiff base from the last step leads to the starch-PdCl2 catalyst (Scheme 59).
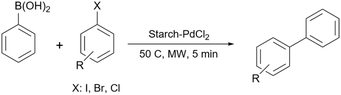 |
| Scheme 58 Synthesis of biphenyls using starch-PdCl2. | |
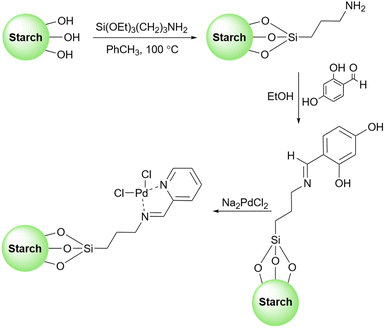 |
| Scheme 59 Steps for the preparation of starch-PdCl2. | |
In 2018, Baran's group disclosed another method for the palladium-catalyzed synthesis of biphenyls through a Suzuki-cross-coupling reaction (Scheme 60).191 They prepared a mixed composition of starch and chitosan by adding starch to the acidic solution containing chitosan. Then, the Na2PdCl4 was added dropwise to a mixture containing the prepared composite of starch/chitosan, and the solution was stirred for the next 3 hours. Finally, NaNBH4 was added to the solution to reduce the Pd(II) to Pd(0) and palladium nanoparticles precipitated on the surface of the mixed carbohydrate composite.
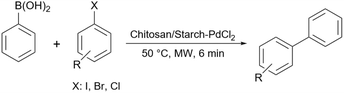 |
| Scheme 60 Synthesis of hydroquinazolinones using alkaline starch. | |
In 2012, Khalafi-Nezhad and Panahi reported a silica starch loaded with palladium nanoparticles as an efficient heterogeneous catalyst for the synthesis of p-teraryls using the Suzuki reaction (Scheme 61).192 They attached the starch to the silica chloride which was prepared by the reaction between silicon dioxide (silica gel) and thionyl chloride. Then, they added the palladium acetate to the achieved silica starch from the previous step to obtain the palladium nanoparticles-loaded silica starch (Scheme 62).
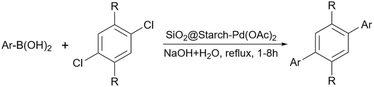 |
| Scheme 61 Synthesis of hydroquinazolinones using SiO2@starch-Pd(OAC)2. | |
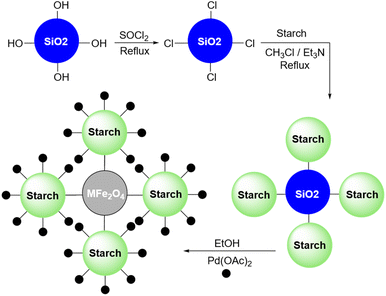 |
| Scheme 62 Preparation of SiO2@starch-Pd(OAC)2. | |
Five years later, this research group used the same catalyst for the Pd-catalyzed Buchwald–Hartwig C–N cross-coupling reaction (Scheme 63).193 They showed that Pd nanoparticles on silica-starch were powerful enough to promote the C–N cross-coupling reaction between aryl halides and amines almost as good as the carbon–carbon coupling reaction that they reported 5 years ago.
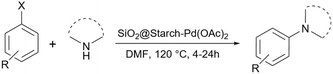 |
| Scheme 63 Synthesis of N-aryl amines using SiO2@starch-Pd(OAC)2. | |
In 2018, Khalafi and Panahi reported another application for their Pd nanoparticles on the silica-starch catalyst (Scheme 64).194 This time the authors used a magnetic version of their catalyst by replacing simple silica with magnetic silica (Fe3O4@SiO2) (Scheme 65). They used this magnetic version of the palladium nanoparticles-loaded silica-starch as a catalyst for the Heck reaction between iodobenzene and styrene.
 |
| Scheme 64 Palladium-catalyzed Heck reaction between iodobenzene and styrene. | |
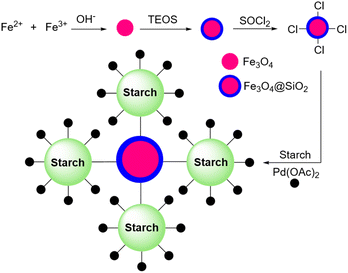 |
| Scheme 65 Preparation of SiO2@starch-Pd(OAC)2. | |
Rezapour et al. reported another palladium-loaded starch catalyst for the cross-coupling reaction (Scheme 66).195 This research group used a palladium niacin complex immobilized on starch-coated maghemite nanoparticles (γ-Fe2O3@starch@niacin-Pd(II)) for some of the homo- and cross-coupling reactions (Suzuki, Ullmann, and Sonogashira reactions (Scheme 67)).
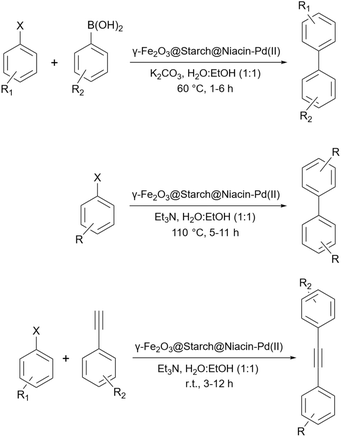 |
| Scheme 66 Palladium-catalyzed Suzuki, Ullmann, and Sonogashira reactions. | |
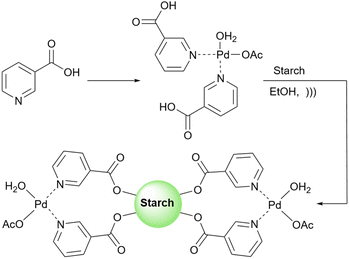 |
| Scheme 67 Preparation of γ-Fe2O3@starch@niacin-Pd(II) as a catalyst for coupling reactions. | |
Arghan and colleagues reported a magnetic starch loaded with cobalt nanoparticles as a catalyst for the Mizoroki–Heck and the Suzuki–Miyaura reactions (Scheme 68).196 To prepare the catalyst they coated the Fe3O4 nanoparticles with starch and then modified the surface of starch with APTES. Using 2,2′-furil, they managed to achieve the related Schiff base. Finally, they added cobalt salt as Co(OAc)2 ·4H2O to the solution containing the Schiff base and prepared the desired catalyst (Scheme 69).
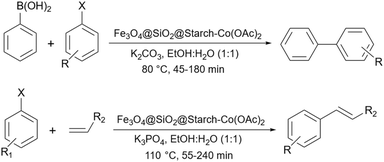 |
| Scheme 68 Cobalt-catalyzed Suzuki–Miyaura and Mizoroki–Heck coupling reactions. | |
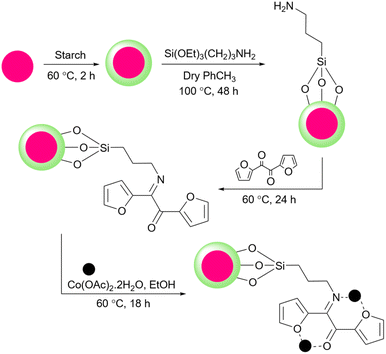 |
| Scheme 69 Preparation of Fe3O4@starch-Co(OAc)2. | |
Veisi et al. presented another starch-based palladium catalyst for the coupling reaction. They prepared a Fe3O4@chitosan-starch core–shell decorated with palladium nanoparticles as a versatile for the Suzuki–Miyaura coupling and also reduction of nitrophenol to aminophenol reactions under ultrasonic conditions (Scheme 70).197 Based on the mechanism proposed by the authors, the key role in these transformations is on the palladium's shoulder which can be changed from Pd(0) to Pd(II) and make the coupling and reduction reactions possible.
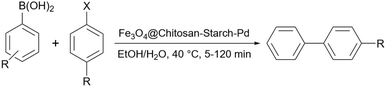 |
| Scheme 70 Suzuki reaction of 4-methylbromobenzene with phenyl boronic acid using Fe3O4@Chitosan-starch/Pd. | |
Recently, Koukabi and Arghan used a little bit different magnetic catalyst compared to their previous work196 to promote Mizoroki–Heck and Suzuki–Miyaura reactions.198 They prepared the Fe3O4@starch core–shell like before, however, instead of modifying it with a linker they just added Co(OAc)2·4H2O to the solution containing this magnetic structure to prepare their intended Fe3O4@starch-Co(II) catalyst. As we expect and also the authors proposed in their paper, Co(II) can change to Co(IV), to disconnect the Ar–X bond and help the coupling reaction to occur. Very recently, Li et al. another starch Schiff base as a support for palladium for the Suzuki coupling reactions (Scheme 71).49 They used almost the same technique that we have seen before for the preparation of starch Schiff base190 using APTES as a linker and 2-aminobenzaldehyde as a required carbonyl group.
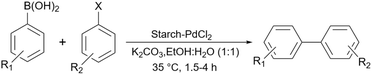 |
| Scheme 71 Suzuki–Miyaura coupling reactions of aryl halides with arylboronic acids using starch-PdCl2 as a catalyst. | |
In addition to the mentioned examples of published work about using starch as support for coupling reactions, there is more work in this field that we can talk about. These protocols are gathered in Table 1.
Table 1 Coupling reactions catalyzed by metal-supported starch
Entry |
Year |
Reaction type |
Catalyst |
Refs. |
1 |
2005 |
Suzuki, Heck, and Sonogashira |
Starch@Schiff base-Pd(OAc)2 |
199 |
2 |
2008 |
Suzuki, Heck, and Sonogashira |
Mesoporous starch-Pd(OAc)2 |
200 |
3 |
2010 |
Miyaura–Suzuki |
Starch-PdCl2 |
201 |
4 |
2011 |
Sonogashira |
SiO2@starch@Pd(OAc)2 |
202 |
5 |
2013 |
Miyaura–Heck |
Starch@ethylene diamine-PdCl2 |
203 |
6 |
2017 |
Suzuki–Miyaura |
Starch@polyethylenimine@Pd(0) |
204 |
7 |
2020 |
Heck and Tsuji-Trost |
Fe3O4@starch-PdCl2 |
205 |
2.5.19 Oxidation reactions.
2.5.19.1 Oxidation of alkanes. Because of the presence of the carbonyl group, aryl carbonyls can be used as synthetic precursors to reach many products.206 There are many reports about the synthesis of aryl carbonyl from aryl alkanes207–209 including our previous work.210 In 2018, Sodhi and Paul prepared a starch-based catalyst to convert aryl alkane substrates to related aryl carbonyls. To do the job, they prepared and used a Co(acac)2 supported amine-functionalized silica/starch as a catalyst alongside t-BuOOH as an oxidant agent (Scheme 72).211
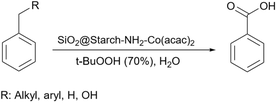 |
| Scheme 72 Oxidation of aryl alkane using silica/starch-NH2@Co(acac)2 as a catalyst. | |
2.5.19.2 Oxidation of alcohols. The oxidation of alcohol is one of the most popular and key reactions in organic chemistry and many related reports are published every year.212–216 The literature review shows there are some published research papers about using starch as catalyst support for the oxidation reactions of alcohol.217 For example, one could point out the work that has been done by Yang's research group. This group introduced a novel method for the preparation of the starch-Schiff base Co(II) complex and used this complex as a catalyst to oxidize cyclohexanes.218 Another starch-based catalyst for the oxidation of alcohols emerged in 2013. Verma and colleagues published a research paper about the vanadium-catalyzed oxidation reaction of alcohols (Scheme 73).219 They used an oxo-vanadium Schiff base grafted on the surface of a functionalized starch as support. To prepare the catalyst, the authors functionalized the hydroxyl groups of the corn starch with 3-mercaptopropyltrimethoxysilane. Then, they prepared a Schiff base from amino ethanol and 2,4-dihydroxy benzaldehyde and added Vo(acac)2 to reach the related vanadium complex. After modifying the prepared vanadium complex with propargyl bromide, they connect this modified vanadium complex to the modified starch prepared in the first step (Scheme 74). In the same year, this group provided another catalyst for the oxidation reaction of alcohols. They prepared palladium nanoparticles grafted on nanocrystalline starch to convert primary and secondary alcohols to the related aldehydes and ketones (Scheme 75).220
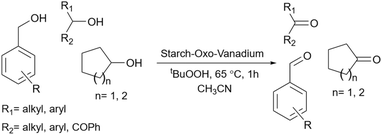 |
| Scheme 73 Preparation of starch-oxo-vanadium Schiff base. | |
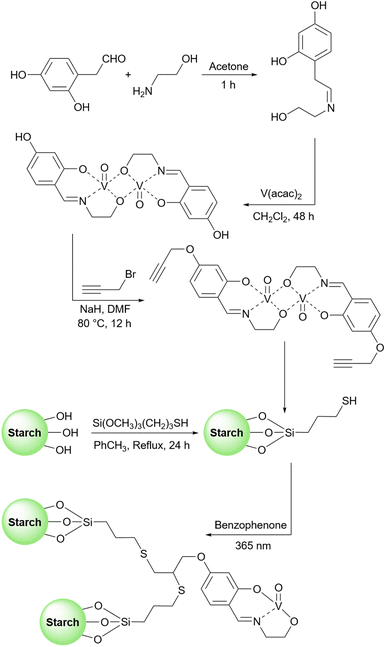 |
| Scheme 74 Preparation of starch-oxo-vanadium Schiff base. | |
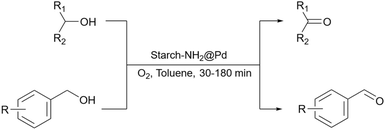 |
| Scheme 75 Preparation of silica/starch-NH2@Pd(0). | |
Sharma et al. proposed another method to produce grafted metallic palladium on the surface of starch and used this structure as a catalyst for the oxidation of alcohols (Scheme 76).221 First, they prepared Fe3O4 particles and then modified their surface using 1,6-hexanediamine. Next, they coated the amine-functionalized magnetic nanoparticles from the last step with starch chloride. Finally, Pd(OAc)2 was added to the solution containing starch-coated Fe3O4 particles and used NaBH4 to reduce Pd(II) to Pd(0). The authors also used the prepared catalyst for other reactions including reduction of nitroarenes and synthesis of functionalized amines from aldehydes at room temperature (Scheme 77).
 |
| Scheme 76 Preparation of Fe3O4@starch–NH2–Pd. | |
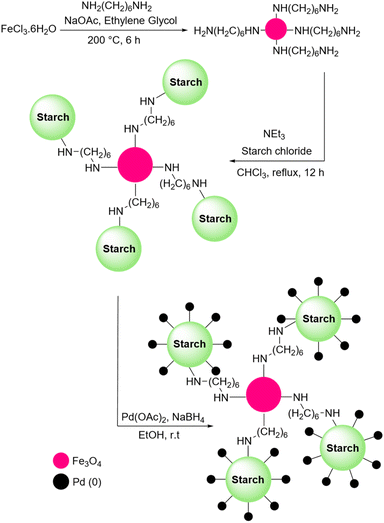 |
| Scheme 77 Preparation of Fe3O4@starch–NH2–Pd(0). | |
Again in 2013, another method based on using starch as support was proposed, this time by the research group of Ebitani.222 They prepared some kind of platinum/gold alloy nanoparticles-supported hydrotalcite and used this catalyst to oxidize polyols at room temperature. The results showed the different percentages of Pt/Au can affect the amount of conversion of glycerol substrate to its oxidized forms like glyceric acid, tartronic acid, glycolic acid, and oxalic acid.
2.5.19.3 Oxidation of aldehydes. In 2019, Li's research group proposed a novel way for the synthesis of benzoic acid derivatives based on using starch as support (Scheme 78).223 They added HAuCl4·4H2O to the solution containing starch to reach a gold nanoparticle-supported starch and used that as a catalyst alongside H2O2 as an oxidant to convert benzaldehydes to their corresponding benzoic acids. According to the authors, in this catalytic system, starch doesn't have any specific direct influence on the outcome but works as a ligand and reducing agent for gold nanoparticles which play the main role as a catalyst.
 |
| Scheme 78 Oxidation of benzaldehyde using of starch@Au. | |
Very recently, Ravichandran et al. introduced another starch-based catalyst to convert benzaldehyde to corresponding carboxylic acid.224 They prepared Fe3O4@starch@Ag via the grinding method and used this nanocomposite as a catalyst for the oxidation of benzaldehyde. Also, the published results indicate that the catalyst is capable of decreasing some common dyes including Methylene blue and Methyl red.
2.5.19.4 Epoxidation of alkenes. Epoxides can be very common intermediates in many organic reactions and alkenes are one of the major sources to reach epoxide.225,226 Vegetable oils are one of those compounds that contain double bounds and from a commercial point of view, the epoxidation of these bonds is very important. The reason is that epoxidized vegetable oils have many applications for the synthesis of polyurethane foams, synthetic detergents, coatings agents, and lubricant compounds.227 In 2015, Pan's research group presented a starch-Schiff base-Mo(acac)2 catalyst for the epoxidation of stillingia oil as a vegetable oil using tert-butyl hydroperoxide (TBHP) as an oxidizing agent (Scheme 79).228
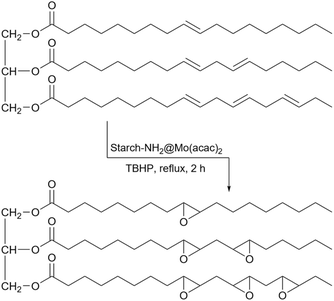 |
| Scheme 79 Epoxidation of stillingia oil using starch-NH2@Mo(acac)2. | |
2.5.19.5 Oxidation of sulfoxides. Sulfoxides are valuable targets in synthetic and medicinal chemistry and the oxidation of sulfide is one of the easiest ways to prepare these compounds. In 2017, Hong and Yan prepared a starch@Schiff base-Mo(acac)2 as a catalyst to convert sulfides to sulfoxide using t-BuOOH as an oxidant (Scheme 80).229
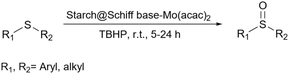 |
| Scheme 80 Oxidation of sulfides to sulfoxides using starch@Schiff base-Mo(acac)2. | |
2.5.19.6 Synthesis of cyclic carbonates. There are many reports about using carbon dioxide as an undesirable molecule to prepare much more precious compounds. One of these reactions is converting epoxides to their related cyclic carbonate.230–233 In 2012, Kumar et al. published prepared and employed a starch-based catalyst to convert substituted epoxides to cyclic carbonates (Scheme 81). To do that, they prepared a starch Schiff base and attached Ni(acac)2 to the surface of the Schiff base.234
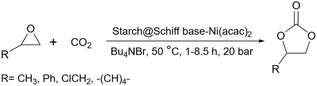 |
| Scheme 81 Synthesis of cyclic carbonates from epoxides using starch@Schiff base-Ni(acac)2. | |
2.5.19.7 Oxidative cyanation of tertiary amines. The oxidative cyanation of tertiary amines is an interesting method for the synthesis of a-aminonitriles.235–237 In 2011, Verma et al. reported a ruthenium-catalyzed cyanation of aromatic tertiary amines (Scheme 82).238 They prepared a starch@ionic liquid-RuCl4 as a novel catalyst for this transformation. To prepare the ionic liquid layer around the starch, the authors took advantage of the reaction between 1-methylimidazole and 3-trimethoxysilylpropyl chloride. Then, they attached the prepared 1-methyl-3-(silylpropyl)imidazolium chloride from the last step to the surface of starch under reflux conditions in toluene and used the achieved starch@ionic liquid to reach the intended starch@ionic liquid-RuCl4.
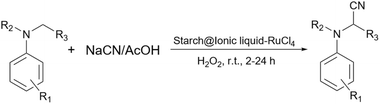 |
| Scheme 82 Oxidative cyanation of tertiary amines using starch@ionic liquid-RuCl4. | |
3. Conclusion
In this review, the applications of starch as catalyst and catalyst support have been discussed. Literature review shows that starch has been used in different forms for a wide range of organic reactions. The application of starch in these reactions as a catalyst can be summarised based on the type of starch, i.e., unfunctionalized and functionalized starch. Unfunctionalized starch could be used as simple or attached to magnetic particles and functionalized starch could be employed with simple acidic/basic modification of the surface of starch or anchored to a complex structure using an appropriate linker. In either case, starch can have a simple or core–shell structure. Based on what is presented here, simple starch like raw starch or starch nanoparticles are good candidates to promote acid–base reactions like multi-component organic transformations. Also, modified starch with suitable functional groups like acidic or basic starch can do the same job even with greater efficiency in some cases. However, to do more difficult reactions including coupling and oxidation reactions that the presence of metals is almost necessary, starch or its modified versions can be used as efficient support to hold the desired metal or metal oxide like molecular pincers and facilitate the process. Like any other catalyst starch has its disadvantages including low tolerance to a wide range of temperatures, and its tendency to absorb and hold water. However, properties such as being cheap, environmentally friendly, and easy to prepare can help balance the situation. All in all, regardless of whether we use starch, as a catalyst or catalyst support, this biodegradable and environmentally friendly biopolymer is a very good option for accelerating many organic reactions.
Conflicts of interest
There are no conflicts to declare.
References
- B.-E. Channab, A. El Idrissi, M. Zahouily, Y. Essamlali and J. C. White, Int. J. Biol. Macromol., 2023, 238, 124075 CrossRef CAS PubMed.
- M. Emeje, Chemical Properties of Starch, IntechOpen, London, 2020 Search PubMed.
- K. Korompokis, K. Verbeke and J. A. Delcour, Compr. Rev. Food Sci. Food Saf., 2021, 20, 5965–5991 CrossRef CAS PubMed.
- A. Bahaji, J. Li, Á. M. Sánchez-López, E. Baroja-Fernández, F. J. Muñoz, M. Ovecka, G. Almagro, M. Montero, I. Ezquer, E. Etxeberria and J. Pozueta-Romero, Biotechnol. Adv., 2014, 32, 87–106 CrossRef CAS PubMed.
- S. Kim, A. Biswas, M. Singh, S. C. Peterson and S. Liu, J. Cereal Sci., 2012, 56, 720–725 CrossRef CAS.
- A. Zarski, K. Bajer and J. Kapuśniak, Polymers, 2021, 13, 832 CrossRef CAS PubMed.
- V. K. Khlestkin, S. E. Peltek and N. A. Kolchanov, Carbohydr. Polym., 2018, 181, 460–476 CrossRef CAS PubMed.
- N. A. Abd El-Ghany, M. H. A. Elella, H. M. Abdallah, M. S. Mostafa and M. Samy, J. Polym. Environ., 2023, 31, 2792–2825 CrossRef CAS.
- N. Masina, Y. E. Choonara, P. Kumar, L. C. Du Toit, M. Govender, S. Indermun and V. Pillay, Carbohydr. Polym., 2017, 157, 1226–1236 CrossRef CAS PubMed.
- M. Haroon, L. Wang, H. Yu, N. M. Abbasi, Z.-A. Zain-ul-Abdin, M. Saleem, R. U. Khan, R. S. Ullah, Q. Chen and J. Wu, RSC Adv., 2016, 6, 78264–78285 RSC.
- J. Watcharakitti, E. E. Win, J. Nimnuan and S. M. Smith, Polymers, 2022, 14, 2023 CrossRef CAS PubMed.
- Y. F. Zuo, J. Gu, Z. Qiao, H. Tan, J. Cao and Y. Zhang, Int. J. Biol. Macromol., 2015, 72, 391–402 CrossRef CAS PubMed.
- A. Gilet, C. Quettier, V. Wiatz, H. Bricout, M. Ferreira, C. Rousseau, E. Monflier and S. Tilloy, Green Chem., 2018, 20, 1152–1168 RSC.
- H. Bakouri and K. Guemra, Int. J. Biol. Macromol., 2019, 125, 1118–1127 CrossRef CAS PubMed.
- S. H. Clasen, C. M. O. Müller, A. L. Parize and A. T. N. Pires, Carbohydr. Polym., 2018, 180, 348–353 CrossRef CAS PubMed.
- B. Klein, N. L. Vanier, K. Moomand, V. Z. Pinto, R. Colussi, E. Da Rosa Zavareze and A. R. G. Dias, Food Chem., 2014, 155, 167–173 CrossRef CAS PubMed.
- Y.-R. Zhang, X.-L. Wang, G.-M. Zhao and Y.-Z. Wang, Carbohydr. Polym., 2012, 87, 2554–2562 CrossRef CAS.
- A. S. Ayoub and S. S. H. Rizvi, J. Plast. Film Sheeting, 2009, 25, 25–45 CrossRef CAS.
- H.-S. Kim, D.-K. Hwang, B.-Y. Kim and M.-Y. Baik, Food Chem., 2012, 130, 977–980 CrossRef CAS.
- D. Cui, M. Liu, L. Wu and Y. Bi, Int. J. Biol. Macromol., 2009, 44, 294–299 CrossRef CAS PubMed.
- F. Akman, A. S. Kazachenko, N. Y. Vasilyeva and Y. N. Malyar, J. Mol. Struct., 2020, 1208, 127899 CrossRef CAS.
- S. Dhital, G. Dolan, J. R. Stokes and M. J. Gidley, Food Funct., 2014, 5, 579–586 RSC.
- Y. Qin, H. Zhang, Y. Dai, H. Hou and H. Dong, Materials, 2019, 12, 1705 CrossRef CAS PubMed.
- S. Wang and L. Copeland, Crit. Rev. Food Sci. Nutr., 2015, 55, 1081–1097 CrossRef CAS PubMed.
- H. Cheng, L. Chen, D. J. McClements, T. Yang, Z. Zhang, F. Ren, M. Miao, Y. Tian and Z. Jin, Trends Food Sci. Technol., 2021, 114, 70–82 CrossRef CAS.
- A. Gamage, A. Liyanapathiranage, A. Manamperi, C. Gunathilake, S. Mani, O. Merah and T. Madhujith, Sustainability, 2022, 14, 6085 CrossRef CAS.
- X. Hou, H. Wang, Y. Shi and Z. Yue, Carbohydr. Polym., 2023, 302, 120392 CrossRef CAS PubMed.
- D. Le Corre and H. Angellier-Coussy, React. Funct. Polym., 2014, 85, 97–120 CrossRef CAS.
- S. Mohd Asharuddin, N. Othman, W. A. H. Altowayti, N. Abu Bakar and A. Hassan, Environ. Technol. Innovation, 2021, 23, 101637 CrossRef CAS.
- A. Rodrigues and M. Emeje, Carbohydr. Polym., 2012, 87, 987–994 CrossRef CAS.
- O. O. Ayodele and F. A. Dawodu, Energy Technol., 2014, 2, 912–920 CrossRef CAS.
- D. Soto, J. Urdaneta, K. Pernía, O. León, A. Muñoz-Bonilla and M. Fernandez-García, Starch, 2016, 68, 37–46 CrossRef CAS.
- I. Ihsanullah, M. Bilal and A. Jamal, Chem. Rec., 2022, 22, e202100312 CrossRef CAS PubMed.
- J. Tang, Q. Zhang, J. Zhou, H. Fang, H. Yang and F. Wang, Food Chem., 2021, 365, 130448 CrossRef CAS PubMed.
- S. Kang, W. Liu, Y. Wang, Y. Wang, S. Wu, S. Chen, B. Yan and X. Lan, J. Taiwan Inst. Chem. Eng., 2022, 135, 104383 CrossRef CAS.
- F. Wang, R. Ma and Y. Tian, Carbohydr. Polym., 2022, 287, 119297 CrossRef CAS PubMed.
- L. García-Guzmán, G. Cabrera-Barjas, C. G. Soria-Hernández, J. Castaño, A. Y. Guadarrama-Lezama and S. Rodríguez Llamazares, Polysaccharides, 2022, 3, 136–177 CrossRef.
- W.-Y. Lou, M.-H. Zong and Z.-Q. Duan, Bioresour. Technol., 2008, 99, 8752–8758 CrossRef CAS PubMed.
- J. Clohessy and W. Kwapinski, Appl. Sci., 2020, 10, 918 CrossRef CAS.
- S. H. Y. S. Abdullah, N. H. M. Hanapi, A. Azid, R. Umar, H. Juahir, H. Khatoon and A. Endut, Renew. Sustainable Energy Rev., 2017, 70, 1040–1051 CrossRef CAS.
- I. Melián-Cabrera, Ind. Eng. Chem. Res., 2021, 60, 18545–18559 CrossRef.
- O. O. Kunle, Starch source and its impact on pharmaceutical applications, in Chemical Properties of Starch, ed. M. Emeje, IntechOpen, 2020 Search PubMed.
- B. O. Fraser-Reid, K. Tatsuta and J. Thiem, Glycoscience: Chemistry and Chemical Biology, Springer-Verlag, Berlin, Heidelberg, 2008 Search PubMed.
- C. C. J. Loh, Nat. Rev. Chem., 2021, 5, 792–815 CrossRef CAS PubMed.
- M. Jaymand, Int. J. Biol. Macromol., 2022, 197, 111–120 CrossRef CAS PubMed.
- K. Arabpourian and F. K. Behbahani, Application of starch in the synthesis of N-substituted pyrroles by a simple and green route, in Greener Synthesis of Organic Compounds, Drugs and Natural Products, ed. A. Nag, CRC Press, Boca Raton, 2022, pp. 191–194 Search PubMed.
- J. Safari, Z. Zarnegar, M. Sadeghi and F. Azizi, Curr. Org. Chem., 2016, 20, 2926–2932 CrossRef CAS.
- C. Shi, Q. Huang, R. Zhang, X. Liang, F. Wang, Z. Liu, M. Liu, H. Hu and Y. Yin, RSC Adv., 2021, 11, 39758–39767 RSC.
- X. Li, Z. Zhou, Y. Wang, J. Dong, X. Jia, Z. Hu, Q. Wei, W. Zhang, Y. Jiang, J. Zhang and Y. Dong, Int. J. Biol. Macromol., 2023, 233, 123596 CrossRef CAS PubMed.
- A. D. Gupta, K. P. Rawat, V. Bhadauria and H. Singh, Carbohydr. Polym., 2021, 269, 117763 CrossRef CAS PubMed.
- S. C. Philkhana, F. O. Badmus, I. C. Dos Reis and R. Kartika, Synthesis, 2021, 53, 1531–1555 CrossRef CAS PubMed.
- S. S. Gholap, Eur. J. Med. Chem., 2016, 110, 13–31 CrossRef CAS PubMed.
- J. Safari and M. Sadeghi, Res. Chem. Intermed., 2016, 42, 8175–8183 CrossRef CAS.
- J. Safari, Z. Abedi-Jazini, Z. Zarnegar and M. Sadeghi, J. Nanopart. Res., 2015, 17, 495 CrossRef.
- Z. Zarnegar, M. Sadeghi, R. Alizadeh and J. Safai, J. Mol. Liq., 2018, 255, 76–79 CrossRef CAS.
- B. N. Ravi, J. Keshavayya, N. M. Mallikarjuna, V. Kumar and S. Kandgal, J. Mol. Struct., 2020, 1204, 127493 CrossRef CAS.
- M. Farouk Elsadek, B. Mohamed Ahmed and M. Fawzi Farahat, Molecules, 2021, 26, 1449 CrossRef PubMed.
- M. E. Khalifa, Acta Chim. Slov., 2018, 65, 1–22 CrossRef CAS PubMed.
- J. Safari and M. Sadeghi, Monatsh. Chem., 2017, 148, 745–749 CrossRef CAS.
- H. Rostami Monjezi, Z. Zarnegar and J. Safari, J. Saudi Chem. Soc., 2019, 23, 973–979 CrossRef CAS.
- M. Ahmadzadeh, M. Sadeghi and J. Safari, J. Chem., 2021, 2021, 1–11 CrossRef.
- M. Kangani, N. Hazeri and M.-T. Maghsoodlou, Iran J. Org. Chem., 2015, 7, 1591–1595 Search PubMed.
- A. A. Mohammadi, M. R. Asghariganjeh and A. Hadadzahmatkesh, Arab. J. Chem., 2017, 10, S2213–S2216 CrossRef CAS.
- Z. Benzekri, S. Sibous, H. Anahmadi, F. El hajri, D. E. Mekkaoui, R. Hsissou, A. Ouasri, A. Souizi, A. Rhandour and S. Boukhris, J. Mol. Struct., 2023, 1281, 135064 CrossRef CAS.
- N. Hazeri, M. T. Maghsoodlou, F. Mir, M. Kangani, H. Saravani and E. Molashahi, Chin. J. Catal., 2014, 35, 391–395 CrossRef CAS.
- S. Agarwal, A. Sethiya, J. Soni, N. Sahiba and P. Teli, Appl. Organometal. Chem., 2022, 36, e6604 CrossRef CAS.
- D. Tejedor, S. Delgado-Hernández, R. Diana-Rivero, A. Díaz-Díaz and F. García-Tellado, Molecules, 2019, 24, 2904 CrossRef PubMed.
- S. Mal and M. Jana, ChemistrySelect, 2022, 7, e202103525 CrossRef CAS.
- M. Kamalzare, M. Bayat and A. Maleki, R. Soc. Open Sci., 2020, 7, 200385 CrossRef CAS PubMed.
- D. S. Aher, K. R. Khillare, L. D. Chavan and S. G. Shankarwar, Monatsh. Chem., 2022, 153, 79–85 CrossRef CAS.
- S. V. Akolkar, N. D. Kharat, A. A. Nagargoje, D. D. Subhedar and B. B. Shingate, Catal. Lett., 2020, 150, 450–460 CrossRef CAS.
- D. Dharmendra, P. Chundawat, Y. Vyas and C. Ameta, J. Chem. Sci., 2022, 134, 47 CrossRef CAS.
- S. Razikazemi, K. Rad-Moghadam and S. Toorchi-Roudsari, New J. Chem., 2018, 42, 12476–12485 RSC.
- R. M. Ward, Q. Gao, H. de Bruyn, R. G. Gilbert and M. A. Fitzgerald, Biomacromolecules, 2006, 7, 866–876 CrossRef CAS PubMed.
- A. Mohammadzadeh, A. P. Marjani and A. Zamani, S. Afr. J. Chem., 2020, 73, 55–63 CrossRef CAS.
- F. Karimi Rad and F. K. Behbahani, Curr. Org. Synth., 2016, 14, 22–39 CrossRef.
- F. M. Moghaddam, S. Aghili, M. Daneshfar, H. Moghimi and Z. Daneshfar, Res. Chem. Intermed., 2023, 49, 1507–1543 CrossRef CAS.
- A. Bouhaoui, M. Eddahmi, M. Dib, M. Khouili, A. Aires, M. Catto and L. Bouissane, ChemistrySelect, 2021, 6, 5848–5870 CrossRef CAS.
- S. Doi, J. H. Clark, D. J. Macquarrie and K. Milkowski, Chem. Commun., 2002, 2632–2633 RSC.
- R. Rezaei and M. R. Sheikhi, Res. Chem. Intermed., 2015, 41, 1283–1292 CrossRef CAS.
- N. Q. H. Doan, N. T. K. Nguyen, V. B. Duong, H. T. T. Nguyen, L. B. Vong, D. N. Duong, N.-T. T. Nguyen, T. L. T. Nguyen, T. T. H. Do and T. N. Truong, ACS Omega, 2022, 7, 33963–33984 CrossRef CAS PubMed.
- F. Hatamjafari, Helv. Chim. Acta, 2013, 96, 1560–1563 CrossRef CAS.
- R. H. Vekariya, K. D. Patel and H. D. Patel, Iran J. Org. Chem., 2015, 7, 1581–1589 Search PubMed.
- J. Sangshetti, S. K. Pathan, R. Patil, S. Akber Ansari, S. Chhajed, R. Arote and D. B. Shinde, Bioorg. Med. Chem., 2019, 27, 3979–3997 CrossRef CAS PubMed.
- H. R. Shaterian and F. Rigi, Starch, 2011, 63, 340–346 CrossRef CAS.
- M. Ahmad, T. A. King, D.-K. Ko, B. H. Cha and J. Lee, J. Phys. D: Appl. Phys., 2002, 35, 1473–1476 CrossRef CAS.
- A. N. Dadhania, V. K. Patel and D. K. Raval, C. R. Chim., 2012, 15, 378–383 CrossRef CAS.
- L. G. Wang, I. Munhenzva, M. Sibrian-Vazquez, J. O. Escobedo, C. H. Kitts, F. R. Fronczek and R. M. Strongin, J. Org. Chem., 2019, 84, 2585–2595 CrossRef CAS PubMed.
- H. R. Shaterian and F. Rigi, Res. Chem. Intermed., 2015, 41, 721–738 CrossRef CAS.
- J. Safari, P. Aftabi, M. Ahmadzadeh, M. Sadeghi and Z. Zarnegar, J. Mol. Struct., 2017, 1142, 33–39 CrossRef CAS.
- P. Biginelli and P. Gazz, Chim. Ital., 1893, 23, 360–416 Search PubMed.
- L. H. S. Matos, F. T. Masson, L. A. Simeoni and M. Homem-de-Mello, Eur. J. Med. Chem., 2018, 143, 1779–1789 CrossRef CAS PubMed.
- R. Rezaei, S. Malek, M. R. Sheikhi and M. K. Mohammadi, Chem. J. Mold., 2013, 8, 101–106 CAS.
- S. M. Prajapati, K. D. Patel, R. H. Vekariya, S. N. Panchal and H. D. Patel, RSC Adv., 2014, 4, 24463–24476 RSC.
- A. Shaabani, A. Rahmati and Z. Badri, Catal. Commun., 2008, 9, 13–16 CrossRef CAS.
- L. Biesen and T. J. J. Müller, Adv. Synth. Catal., 2021, 363, 980–1006 CrossRef CAS.
- J. A. Pereira, A. M. Pessoa, M. N. D. S. Cordeiro, R. Fernandes, C. Prudêncio, J. P. Noronha and M. Vieira, Eur. J. Med. Chem., 2015, 97, 664–672 CrossRef CAS PubMed.
- R. S. Keri, V. Adimule, P. Kendrekar and B. S. Sasidhar, Top. Catal., 2022 DOI:10.1007/s11244-022-01562-0.
- V. A. S. Pardeshi, N. S. Chundawat, S. I. Pathan, P. Sukhwal, T. P. S. Chundawat and G. P. Singh, Synth. Commun., 2021, 51, 485–513 CrossRef CAS.
- A. Farrokhi, M. Jafarpour and F. Feizpour, ChemistrySelect, 2018, 3, 1234–1241 CrossRef CAS.
- F. Cai, B. Hou, S. Zhang, H. Chen, S. Ji, X.-C. Shen and H. Liang, J. Mater. Chem. B, 2019, 7, 2493–2498 RSC.
- M. H. Sayahi, F. Shamkhani, M. Mahdavi and S. Bahadorikhalili, Starch, 2021, 73, 2000257 CrossRef CAS.
- R. M. Lanigan, P. Starkov and T. D. Sheppard, J. Org. Chem., 2013, 78, 4512–4523 CrossRef CAS PubMed.
- P. Nagaraaj and V. Vijayakumar, Org. Chem. Front., 2019, 6, 2570–2599 RSC.
- M. Kamal, T. Jawaid, U. A. Dar and S. A. Shah, Amide as a potential pharmacophore for drug designing of novel anticonvulsant compounds, in Chemistry of Biologically Potent Natural Products and Synthetic Compounds, ed. S.-U. Islam and J. A. Banday, Wiley, 2021, pp. 319–342 Search PubMed.
- W. Ma, B. Xu, R. Sun, Y.-J. Xu and J.-F. Ge, J. Mater. Chem. B, 2021, 9, 2524–2531 RSC.
- Y. Ma, J. Li, M. Tian, Y. Liu and A. Wei, Ind. Crops Prod., 2020, 155, 112770 CrossRef CAS.
- K. Yan, J. Wang, Z. Wang and L. Yuan, Chem. Commun., 2023, 59, 382–400 RSC.
- R. Wani and H. K. Chaudhari, Org. Prep. Proced. Int., 2023, 1–12 CrossRef.
- A. G. Al-kaf, Quinazolinone and Quinazoline Derivatives, IntechOpen, London, 2020 Search PubMed.
- D. Wang and F. Gao, Chem. Cent. J., 2013, 7, 95 CrossRef PubMed.
- H. R. Lobo, B. S. Singh and G. S. Shankarling, Catal. Commun., 2012, 27, 179–183 CrossRef CAS.
- F. Tamaddon and M. KazemiVarnamkhasti, Carbohydr. Res., 2017, 437, 9–15 CrossRef CAS PubMed.
- F. Tamaddon and M. Varnamkhasti, Curr. Catal., 2017, 6, 57–66 CrossRef CAS.
- X. Bao, X. Wang, J.-M. Tian, X. Ye, B. Wang and H. Wang, Org. Biomol. Chem., 2022, 20, 2370–2386 RSC.
- G. Mustafa, M. Zia-ur-Rehman, S. H. Sumrra, M. Ashfaq, W. Zafar and M. Ashfaq, J. Mol. Struct., 2022, 1262, 133044 CrossRef CAS.
- Z. Zhao, X. Dai, C. Li, X. Wang, J. Tian, Y. Feng, J. Xie, C. Ma, Z. Nie, P. Fan, M. Qian, X. He, S. Wu, Y. Zhang and X. Zheng, Eur. J. Med. Chem., 2020, 186, 111893 CrossRef CAS PubMed.
- A. Ramshini, S. M. Nezhad, S. A. Pourmousavi, E. Nazarzadeh Zare, M. Pourjafar and E. Sharifi, Catalysts, 2023, 13, 908 CrossRef CAS.
- T. Khosousi, M. Ahmadzadeh, N. Afrouz and M. Sadeghi, Results Chem., 2023, 6, 101063 CrossRef CAS.
- Z. Zarnegar, H. R. Monjezi and J. Safari, J. Mol. Struct., 2019, 1193, 14–23 CrossRef CAS.
- J. Dai, S. Tian, X. Yang and Z. Liu, Front. Chem., 2022, 10, 891484 CrossRef CAS PubMed.
- M. M. Matin, P. Matin, M. R. Rahman, T. Ben Hadda, F. A. Almalki, S. Mahmud, M. M. Ghoneim, M. Alruwaily and S. Alshehri, Front. Mol. Biosci., 2022, 9, 864286 CrossRef CAS PubMed.
- A. Pourjavadi, A. Motamedi, S. H. Hosseini and M. Nazari, RSC Adv., 2016, 6, 19128–19135 RSC.
- R. Bonyasi, M. Gholinejad, F. Saadati and C. Nájera, New J. Chem., 2018, 42, 3078–3086 RSC.
- P. Gupta, P. Kumar, B. Syal and T. Shamim, Res. Chem. Intermed., 2022, 48, 4601–4615 CrossRef CAS.
- A. V. Dolzhenko, Heterocycles, 2017, 94, 1819 CrossRef CAS.
- A. Nasiri, M. A. Khalilzadeh and D. Zareyee, J. Coord. Chem., 2022, 75, 256–279 CrossRef CAS.
- S. Kumar and Ritika, Futur. J. Pharm. Sci., 2020, 6, 121 CrossRef.
- V. Sharma, P. Kumar and D. Pathak, J. Heterocyclic Chem., 2010 Search PubMed.
- D. F. Taber and P. K. Tirunahari, Tetrahedron, 2011, 67, 7195–7210 CrossRef CAS PubMed.
- M.-Z. Zhang, Q. Chen and G.-F. Yang, Eur. J. Med. Chem., 2015, 89, 421–441 CrossRef CAS PubMed.
- A. A. Atia and M. Kimura, Tetrahedron, 2021, 90, 132213 CrossRef CAS.
- G. N. Vaidya, S. K. Lokhande, S. D. Shinde, D. P. Satpute, G. Narang and D. Kumar, Green Chem., 2022, 24, 4921–4927 RSC.
- S. Mallick, P. Mukhi, P. Kumari, K. R. Mahato, S. K. Verma and D. Das, Catal. Lett., 2019, 149, 3501–3507 CrossRef CAS.
- F. C. Teixeira, H. Ramos, I. F. Antunes, M. J. M. Curto, M. T. Duarte and I. Bento, Molecules, 2006, 11, 867–889 CrossRef CAS PubMed.
- S.-G. Zhang, C.-G. Liang and W.-H. Zhang, Molecules, 2018, 23 Search PubMed.
- R. K. Sodhi, A. Changotra and S. Paul, Catal. Lett., 2014, 144, 1819–1831 CrossRef CAS.
- T. Gessner and U. Mayer, Triarylmethane and diarylmethane dyes, in Ullmann's Encyclopedia of Industrial Chemistry, Wiley-VCH Verlag GmbH & Co. KGaA, Weinheim, Germany, 2000 Search PubMed.
- R. Kshatriya, V. P. Jejurkar and S. Saha, Eur. J. Org Chem., 2019, 2019, 3818–3841 CrossRef CAS.
- X. Liu, X. Wu, L. Zhang, X. Lin and D. Huang, Synthesis, 2020, 52, 2311–2329 CrossRef CAS.
- E. Rafiee and M. Khodayari, J. Mol. Catal. A: Chem., 2015, 398, 336–343 CrossRef CAS.
- J. Safari, Z. Zarnegar, M. Sadeghi and A. Enayati-Najafabadi, J. Mol. Struct., 2016, 1125, 772–776 CrossRef CAS.
- H.-W. Liu, K. Huang, J.-D. Mi, Y. Jia, M.-Y. Huang and Y.-Y. Jiang, Polym. Adv. Technol., 2003, 14, 355–359 CrossRef CAS.
- S. Chairam, W. Konkamdee and R. Parakhun, J. Saudi Chem. Soc., 2017, 21, 656–663 CrossRef CAS.
- L. Qi, Z. Luo and X. Lu, Green Chem., 2018, 20, 1538–1550 RSC.
- M. Gholinejad, N. Dasvarz, M. Shojafar and J. M. Sansano, Inorg. Chim. Acta, 2019, 495, 118965 CrossRef CAS.
- A. Nasiri, M. A. Khalilzadeh and D. Zareyee, Inorg. Nano-Met. Chem., 2021, 1–15 Search PubMed.
- S. Taheri, M. M. Heravi and P. Mohammadi, Diamond Relat. Mater., 2022, 126, 109078 CrossRef CAS.
- Y. Lan, H. Veisi, F. Ebrahimi, S. Hemmati and B. Karmakar, Inorg. Chem. Commun., 2023, 156, 111136 CrossRef CAS.
- K. Lauder, A. Toscani, N. Scalacci and D. Castagnolo, Chem. Rev., 2017, 117, 14091–14200 CrossRef CAS PubMed.
- M. Gholinejad, B. Karimi, A. Aminianfar and M. Khorasani, ChemPlusChem, 2015, 80, 1573–1579 CrossRef CAS PubMed.
- F. Saadati, M. Gholinejad, H. Janmohammadi and S. Shaybanizadeh, Lett. Org. Chem., 2018, 15, 79–86 CrossRef CAS.
- M. Tajbakhsh, F. Mazhari and M. Mavvaji, Org. Prep. Proced. Int., 2022, 1–14 Search PubMed.
- Q. N. B. Nguyen, H. A. N. Le, P. D. Ly, H. B. Phan and P. H. Tran, Chem. Commun., 2020, 56, 13005–13008 RSC.
- M. Hong, J. Min, S. Wu, J. Li, J. Wang, L. Wei, Z. Ling, K. Li and S. Wang, Appl. Organomet. Chem., 2020, 34, e5411 CrossRef CAS.
- M. Hong, S. Wu, J. Li, J. Wang, L. Wei and K. Li, Catal. Commun., 2020, 136, 105909 CrossRef CAS.
- M. A. A. B. Abdul Rani, N. A. Karim and S. K. Kamarudin, Int. J. Energy Res., 2022, 46, 18996–19050 CrossRef CAS.
- B. Agarwal, K. Kailasam, R. S. Sangwan and S. Elumalai, Renew. Sustainable Energy Rev., 2018, 82, 2408–2425 CrossRef CAS.
- A. S. Matharu, S. Ahmed, B. Almonthery, D. J. Macquarrie, Y.-S. Lee and Y. Kim, ChemSusChem, 2018, 11, 716–725 CrossRef CAS PubMed.
- S. Askin, H. Tahtaci, C. Türkeş, Y. Demir, A. Ece, G. Akalın Çiftçi and Ş. Beydemir, Bioorg. Chem., 2021, 113, 105009 CrossRef CAS PubMed.
- S. Cascioferro, G. L. Petri, B. Parrino, D. Carbone, N. Funel, C. Bergonzini, G. Mantini, H. Dekker, D. Geerke, G. J. Peters, G. Cirrincione, E. Giovannetti and P. Diana, Eur. J. Med. Chem., 2020, 189, 112088 CrossRef PubMed.
- A. Cristina, D. Leonte, L. Vlase, L. C. Bencze, S. Imre, G. Marc, B. Apan, C. Mogoșan and V. Zaharia, Molecules, 2018, 23, 2425 CrossRef PubMed.
- S. Bahadorikhalili, S. Ansari, H. Hamedifar and M. Mahdavi, Int. J. Biol. Macromol., 2019, 135, 453–461 CrossRef CAS PubMed.
- J. Kim, H. Kim and S. B. Park, J. Am. Chem. Soc., 2014, 136, 14629–14638 CrossRef CAS PubMed.
- F. Sánchez-Sancho, M. Escolano, D. Gaviña, A. G. Csáky, M. Sánchez-Roselló, S. Díaz-Oltra and C. Del Pozo, Pharmaceuticals, 2022, 15, 948 CrossRef PubMed.
- R. Kaur, S. Chaudhary, K. Kumar, M. K. Gupta and R. K. Rawal, Eur. J. Med. Chem., 2017, 132, 108–134 CrossRef CAS PubMed.
- Z. Moutaoukil, C. Ronco and R. Benhida, J. Saudi Chem. Soc., 2022, 26, 101398 CrossRef CAS.
- N. Afradi, N. Foroughifar, H. Pasdar and M. Qomi, Res. Chem. Intermed., 2019, 45, 3251–3271 CrossRef CAS.
- M. Jafarpour and A. Rezaeifard, Transition Met. Chem., 2016, 41, 205–211 CrossRef CAS.
- A. Saeed, Eur. J. Med. Chem., 2016, 116, 290–317 CrossRef CAS PubMed.
- A. Marandi, E. Nasiri, N. Koukabi and F. Seidi, Int. J. Biol. Macromol., 2021, 190, 61–71 CrossRef CAS PubMed.
- Z.-W. Chen, D.-N. Ye, Y.-P. Qian, M. Ye and L.-X. Liu, Tetrahedron, 2013, 69, 6116–6120 CrossRef CAS.
- S. Hou, H. Yang, B. Cheng, H. Zhai and Y. Li, Chem. Commun., 2017, 53, 6926–6929 RSC.
- S. B. Ötvös, Z. Szécsényi and F. Fülöp, ACS Sustainable Chem. Eng., 2019, 7, 13286–13293 CrossRef.
- S. A. Mousavi-Mashhadi and A. Shiri, J. Iran. Chem. Soc., 2022, 19, 4523–4534 CrossRef CAS.
- M. D. Garba, M. Usman, S. Khan, F. Shehzad, A. Galadima, M. F. Ehsan, A. S. Ghanem and M. Humayun, J. Environ. Chem. Eng., 2021, 9, 104756 CrossRef CAS.
- V. Aomchad, À. Cristòfol, F. Della Monica, B. Limburg, V. D'Elia and A. W. Kleij, Green Chem., 2021, 23, 1077–1113 RSC.
- R. Dalpozzo, N. Della Ca’, B. Gabriele and R. Mancuso, Catalysts, 2019, 9, 511 CrossRef.
- Q. Liu, L. Wu, R. Jackstell and M. Beller, Nat. Commun., 2015, 6, 5933 CrossRef PubMed.
- S. W. Knowlden and B. V. Popp, Organometallics, 2022, 41, 1883–1891 CrossRef CAS.
- T. M. Perrone, A. S. Gregory, S. W. Knowlden, N. R. Ziemer, R. N. Alsulami, J. L. Petersen and B. V. Popp, ChemCatChem, 2019, 11, 5814–5820 CrossRef CAS.
- S. Kumar, S. Verma and S. L. Jain, Tetrahedron Lett., 2015, 56, 2430–2433 CrossRef CAS.
- M. Cherif, M. Horchani, Y. O. Al-Ghamdi, S. G. Almalki, Y. E. Alqurashi, H. Ben Jannet and A. Romdhane, J. Mol. Struct., 2020, 1220, 128685 CrossRef CAS.
- A. Amininia, K. Pourshamsian and B. Sadeghi, Russ. J. Org. Chem., 2020, 56, 1279–1288 CrossRef CAS.
- N. Kaur and G. Kaur, Mater. Today Proc., 2022, 48, 1283–1300 CrossRef CAS.
- L.-C. Campeau and N. Hazari, Organometallics, 2019, 38, 3–35 CrossRef CAS PubMed.
- Z. J. Jain, P. S. Gide and R. S. Kankate, Arab. J. Chem., 2017, 10, S2051–S2066 CrossRef CAS.
- J.-A. García-López and M. F. Greaney, Chem. Soc. Rev., 2016, 45, 6766–6798 RSC.
- I. Maluenda and O. Navarro, Molecules, 2015, 20, 7528–7557 CrossRef CAS PubMed.
- T. Baran, J. Colloid Interface Sci., 2017, 496, 446–455 CrossRef CAS PubMed.
- T. Baran, N. Yılmaz Baran and A. Menteş, Appl. Organomet. Chem., 2018, 32, e4075 CrossRef.
- A. Khalafi-Nezhad and F. Panahi, J. Organomet. Chem., 2012, 717, 141–146 CrossRef CAS.
- F. Panahi, F. Daneshgar, F. Haghighi and A. Khalafi-Nezhad, J. Organomet. Chem., 2017, 851, 210–217 CrossRef CAS.
- M. Tukhani, F. Panahi and A. Khalafi-Nezhad, ACS Sustainable Chem. Eng., 2018, 6, 1456–1467 CrossRef CAS.
- E. Rezapour, M. Jafarpour and A. Rezaeifard, Catal. Lett., 2018, 148, 3165–3177 CrossRef CAS.
- M. Arghan, N. Koukabi and E. Kolvari, Appl. Organomet. Chem., 2019, e5075 CrossRef.
- H. Veisi, Z. Joshani, B. Karmakar, T. Tamoradi, M. M. Heravi and J. Gholami, Int. J. Biol. Macromol., 2021, 172, 104–113 CrossRef CAS PubMed.
- N. Koukabi and M. Arghan, Res. Chem. Intermed., 2022, 48, 4553–4577 CrossRef CAS.
- M. J. Gronnow, R. Luque, D. J. Macquarrie and J. H. Clark, Green Chem., 2005, 7, 552 RSC.
- V. L. Budarin, J. H. Clark, R. Luque, D. J. Macquarrie and R. J. White, Green Chem., 2008, 10, 382–387 RSC.
- S. Liu, Q. Zhou and H. Jiang, Chin. J. Chem., 2010, 28, 589–593 CrossRef CAS.
- A. Khalafi-Nezhad and F. Panahi, Green Chem., 2011, 13, 2408 RSC.
- S. Verma, J. Le Bras, S. L. Jain and J. Muzart, Dalton Trans., 2013, 42, 14454–14459 RSC.
- L. Fu, W. Deng, L. Liu and Y. Peng, Appl. Organomet. Chem., 2017, 31, e3853 CrossRef.
- D. Patra, S. Panja and A. Saha, Eur. J. Org Chem., 2020, 2020, 878–883 CrossRef CAS.
- Modern Carbonyl Chemistry, ed. J. Otera, Wiley-VCH, Weinheim, 1st edn, 2008 Search PubMed.
- K. Moriyama, M. Takemura and H. Togo, Org. Lett., 2012, 14, 2414–2417 CrossRef CAS PubMed.
- L. Tanwar, J. Börgel and T. Ritter, J. Am. Chem. Soc., 2019, 141, 17983–17988 CrossRef CAS PubMed.
- G. Urgoitia, R. SanMartin, M. Herrero and E. Domínguez, Catalysts, 2018, 8, 640 CrossRef.
- A. Heydari, M. Sheykhan, M. Sadeghi and I. Radfar, Inorg. Nano-Met. Chem., 2017, 47, 248–255 CrossRef CAS.
- R. K. Sodhi and S. Paul, Curr. Organocatal., 2018, 4, 196–208 CrossRef.
- L. Chen, J. Tang, L.-N. Song, P. Chen, J. He, C.-T. Au and S.-F. Yin, Appl. Catal., B, 2019, 242, 379–388 CrossRef CAS.
- M. N. Kopylovich, A. P. Ribeiro, E. C. Alegria, N. M. Martins, L. M. Martins and A. J. Pombeiro, Catalytic oxidation of alcohols, in Advances in Organometallic Chemistry, 2015, pp. 91–174 Search PubMed.
- S. Najafishirtari, K. Friedel Ortega, M. Douthwaite, S. Pattisson, G. J. Hutchings, C. J. Bondue, K. Tschulik, D. Waffel, B. Peng, M. Deitermann, G. W. Busser, M. Muhler and M. Behrens, Chemistry, 2021, 27, 16809–16833 CrossRef CAS PubMed.
- H. Puetz, E. Puchľová, K. Vranková and F. Hollmann, Catalysts, 2020, 10, 952 CrossRef CAS.
- A. S. Sharma, H. Kaur and D. Shah, RSC Adv., 2016, 6, 28688–28727 RSC.
- Y. Zhao, X. Yang, L. Zhan, S. Ou and J. Tian, J. Mater. Chem., 2011, 21, 4257 RSC.
- D. Yang, L. Gao and W. Zhao, Catal. Lett., 2008, 126, 84–88 CrossRef CAS.
- S. Verma, J. Le Bras, S. L. Jain and J. Muzart, Appl. Catal., A, 2013, 468, 334–340 CrossRef CAS.
- S. Verma, D. Tripathi, P. Gupta, R. Singh, G. M. Bahuguna, L. N. Shivakumar K, R. K. Chauhan, S. Saran and S. L. Jain, Dalton Trans., 2013, 42, 11522–11527 RSC.
- H. Sharma, M. Bhardwaj, M. Kour and S. Paul, Mol. Catal., 2017, 435, 58–68 CrossRef CAS.
- D. Tongsakul, S. Nishimura and K. Ebitani, ACS Catal., 2013, 3, 2199–2207 CrossRef CAS.
- H. Chong, G. Gao and G. Li, Chin. J. Chem. Phys., 2019, 32, 747–752 CrossRef CAS.
- R. Ravichandran, K. Annamalai, A. Annamalai and S. Elumalai, Colloids Surf., A, 2023, 664, 131117 CrossRef CAS.
- I. Ahmad, Shagufta and S. Rehman, Tetrahedron, 2022, 104, 132604 CrossRef CAS.
- J. Mielby and S. Kegnæs, Catal. Lett., 2013, 143, 1162–1165 CrossRef CAS.
- S. M. Danov, O. A. Kazantsev, A. L. Esipovich, A. S. Belousov, A. E. Rogozhin and E. A. Kanakov, Catal. Sci. Technol., 2017, 7, 3659–3675 RSC.
- M. Hong, M.-Y. Yao and H. Pan, RSC Adv., 2015, 5, 91558–91563 RSC.
- M. Hong and J.-M. Yan, Phosphorus, Sulfur Silicon Relat. Elem., 2017, 192, 985–988 CrossRef CAS.
- H. Büttner, L. Longwitz, J. Steinbauer, C. Wulf and T. Werner, Recent developments in the synthesis of cyclic carbonates from epoxides and CO2, in, Chemical Transformations of Carbon Dioxide, ed. X.-F. Wu and M. Beller, Springer International Publishing, Cham, 2018, pp. 89–144 Search PubMed.
- J. W. Comerford, I. D. V. Ingram, M. North and X. Wu, Green Chem., 2015, 17, 1966–1987 RSC.
- A. A. Marciniak, K. J. Lamb, L. P. Ozorio, C. J. Mota and M. North, Curr. Opin. Green Sustainable Chem., 2020, 26, 100365 CrossRef.
- M. North, R. Pasquale and C. Young, Green Chem., 2010, 12, 1514 RSC.
- S. Kumar, S. L. Jain and B. Sain, Catal. Today, 2012, 198, 204–208 CrossRef CAS.
- V. Panwar, P. Kumar, A. Bansal, S. S. Ray and S. L. Jain, Appl. Catal., A, 2015, 498, 25–31 CrossRef CAS.
- S. Saranya, M. Neetha, T. Aneeja and G. Anilkumar, Adv. Synth. Catal., 2020, 362, 4543–4551 CrossRef CAS.
- H. Shen, X. Zhang, Q. Liu, J. Pan, W. Hu, Y. Xiong and X. Zhu, Tetrahedron Lett., 2015, 56, 5628–5631 CrossRef CAS.
- S. Verma, S. L. Jain and B. Sain, ChemCatChem, 2011, 3, 1329–1332 CrossRef CAS.
Footnote |
† This review is dedicated to the late Prof. Dr Javad Safari for his passion and devotion to educating and leading a new generation of Iranian chemists. |
|
This journal is © The Royal Society of Chemistry 2024 |