DOI:
10.1039/C5RA25064A
(Paper)
RSC Adv., 2016,
6, 14682-14691
Rapid synthesis of alkylaminophenols via the Petasis borono–Mannich reaction using protonated trititanate nanotubes as robust solid–acid catalysts†
Received
25th November 2015
, Accepted 18th January 2016
First published on 21st January 2016
Abstract
An effective and rapid synthesis of alkylaminophenols using the one-pot three-component Petasis borono–Mannich (PBM) reaction was carried out using protonated trititanate (H2Ti3O7) nanotubes as a heterogeneous solid–acid catalyst. Complimentary to earlier reports, Ti–O based materials with various morphologies, such as fine particles, nanospheres, nanorods, and tubes were explored for their catalytic activity in the PBM reaction. The XRD pattern revealed the layered trititanate structure of nanotubes and nanorods; anatase and biphasic anatase–rutile structures for fine particles and nanospheres, respectively. Surface area analysis and NH3-TPD adsorption results confirmed the larger surface area and high concentration of Brønsted and Lewis acid sites present in H2Ti3O7 nanotubes. The catalytic efficiency for the PBM reaction is observed in the following order: H2Ti3O7 nanotubes > H2Ti3O7 nanorods > TiO2 nanospheres > TiO2 fine particles. The remarkable catalytic performance of H2Ti3O7 nanotubes was ascribed to a sufficient amount of hydroxy groups and high concentration of Brønsted and Lewis acid sites on the tubular surface, which are essential for adsorption and catalytic reactions. The recyclability of H2Ti3O7 catalyst is another emphasis for the proposed methodology. For the first time, we reported novel alkylaminophenols bearing 2-(pyrimidin-2-yl)-2,5-diazabicyclo[2.2.1]-heptane.
1. Introduction
The Petasis borono–Mannich (PBM) reaction is a convenient one-pot three component reaction due to broad substrate scope, easy access to building blocks and amenability to combinatorial synthesis strategies, besides structurally diverse molecular libraries.1 This PBM reaction can be useful for the synthesis of conduramines,2a,b tetrahydroisoquinoline-1-carboxylic acids,2c 1,4-benzodiazepine-3,5-diones,2d quinoxalines,2e α-amino acids,2f–k allylamines,2l 2H-chromenes,2m–o tertiary amines,2p,q 2-hydroxy-1,4-dihydrobenzoxazines,2r 1,2,3,4-tetrahydrocarbazoles,2s allenes,2t N-benzylpropargylamines,2u oxadiazolones & oxazolidinones,2v 2,5-dihydrofurans,2w α-amino alcohols,2x,y 2-amino morpholines,2z 2-hydroxymorpholines & amino diols,2aa α-hydrazinocarboxylic acids2ab and α-aryl glycines.2ac–ae In general, the reaction tends to proceed exceptionally well when there is a free hydroxyl group on the aldehyde fragment, allowing the formation of an activated boronate complex. For the first time, Petasis and co-workers reported that organoboronic acids react with amines and salicylaldehydes to give alkylaminophenols.3 Many improved procedures with new catalysts such as CuBr + bpy,4a,b BF3·OEt2,4c InBr3,2i Yb(OTf)3 + Pd(TFA)2,4d 4 Å MS4e and CoFe2O4 nanoparticles4f for PBM reaction have been reported. On the other hand for green approach, chitosan,5a glycerol,5b ionic liquids ([bmim]BF4)5c and microwave irradiation5d were employed to accelerate the PBM reaction. The PBM reaction has also been developed in water,6a,b solvent free conditions6c and in solid-phase approach.6d
Nowadays, catalytic applications of metal and metal oxide nanoparticles in various organic transformations have gained much attention due to their stability under reaction conditions, dual acid/base properties, reusability, low toxicity, and non-hygroscopic properties.7 Owing to these attractive features of nano-size metal oxide catalysts; we have focused on titanium oxide (TiO2) based nanostructures, which efficiently catalyze diverse synthetically important reactions.8 Among TiO2 nanomaterials, one-dimensional (1-D) nanostructures such as tubes, rods, and wires attracted much attention due to their unique properties viz., large surface-to-volume-ratio, better dispersibility, decreased inter-crystalline contacts and increased accessibility of acid sites.8a–c,9 1-D TiO2 nanostructures have been extensively investigated in areas such as organocatalysis, photocatalysis, electrocatalysis, sensors, lithium batteries and treatment of autoimmune diseases.10 In the field of catalysis, most of the studies for the material are directed towards the utilization of the material as a support with a large surface area.
In our recent studies, H2Ti3O7 nanotubes (TNT) and nanorods (TNR) were used as efficient solid–acid catalysts for the synthesis of tertiary α-aminophosphonates.11 In continuation of our research interest on the introduction of nano-size TiO2 catalysts in organic synthesis, for the first time we report our investigations towards the synthesis of alkylaminophenols by one-pot three-component PBM reaction of o-hydroxybenzaldehydes, secondary amines, and boronic acids in the presence of three different Ti–O based nanostructures. Further, we also extended this reaction for the synthesis of 2-(pyrimidin-2-yl)-2,5-diazabicyclo[2.2.1]-heptane derived alkylaminophenols (Scheme 1).
 |
| Scheme 1 TNT catalyzed PBM reaction. | |
2. Experimental
2.1. Hydrothermal synthesis of H2Ti3O7 nanotubes and nanorods
High purity chemicals were used for the synthesis of catalysts. Photocatalyst grade titanium dioxide (TiO2 P-25) composed of anatase 80% and rutile 20% was procured from Degussa Corporation, Germany. Titanium dioxide (anatase 99%, TiO2, LAB) was purchased from Merck, India. Analytical reagent (A.R) grade chemicals of sodium hydroxide pellets (97%), hydrochloric acid (37%), and ethanol (99.9) were supplied by Merck, India and used without any further purification. De-ionized water used for synthesis and washing process. In a typical synthesis process, TiO2 fine particles (2.5 g) dispersed in 10 M NaOH aqueous solution (200 mL) and heated either at 130 °C/20 h (for nanotubes) or 180 °C/20 h (for nanorods) in Teflon-lined autoclave (capacity 250 mL). The white precipitate obtained was subjected to washing twice with distilled water (800 mL) followed by dil. HCl (0.1 N, 200 mL), finally with ethanol (200 mL) and dried at 80 °C for 12 h.10c–g Thus obtained catalysts were denoted as TNT and TNR respectively. A portion of TNT was placed in a ceramic boat and calcined at 350 °C for 5 h at a heating rate of 2 °C min−1. The resulting catalyst is denoted as calcined TiO2 nanotubes (cTNT).
2.2. General procedure for TNT catalyzed PBM reaction
To a magnetically stirred mixture of o-hydroxybenzaldehyde (1.0 mmol), secondary amine (1.3 mmol) and boronic acid (1.2 mmol) in 1,4-dioxane was added TNT (15 mg) and the mixture was stirred at 60 °C for respective times (Tables 2 and 3). After completion of the reaction (TLC monitoring), the catalyst was separated by centrifugation and subsequent washing with EtOAc. The recovered catalyst was reused for next cycle. The combined filtrates were washed with 0.15 N HCl (5 mL, to remove the excess secondary amine), 0.1 N NaOH (3 mL, to remove the excess boronic acid) and brine, then dried over anhydrous Na2SO4, filtered, and concentrated on a rotary evaporator to give pure product (not required column purification) as an oil, which slowly turned into colourless solid. The products were structurally assigned by their IR, NMR (1H & 13C) and mass spectral (HRMS) analysis.
Table 1 Physical properties of various Ti–O based catalysts
Catalyst |
Morphology |
Crystal phase |
SBETa (m2 g−1) |
Acid concentrationb (mmol g−1) |
BASc |
LASd |
Surface area of titania nanostructures. Acid sites concentrations of the titania nanostructures were estimated from the NH3-TPD. Brønsted acid sites. Lewis acid sites and n.d. = not detected. |
TFP |
Spherical |
Anatase |
5.1 |
n.d. |
0.006 |
TNP |
Spherical |
Anatase/rutile |
51 |
n.d. |
0.445 |
TNR |
Rods |
Trititanate |
24 |
0.046 |
1.510 |
TNT |
Tubes |
Trititanate |
286 |
1.613 |
2.162 |
cTNT |
Rods, tubes and particles |
Anatase/rutile |
57 |
n.d. |
n.d. |
Table 2 Optimization of reaction parametersa
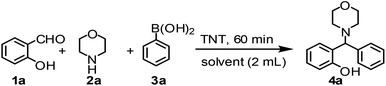
|
Entry |
1a/2a/3a (ratio) |
Solvent |
Catalyst loading (mg) |
T (°C) |
Yieldb (%) |
The bold values are the most effective conditions for the model reaction. Reaction conditions: all reactions were performed on a 1 mmol scale with 2 mL solvent for 60 min. n.d. = not detected. Isolated yield. |
1 |
1.0/1.0/1.0 |
Toluene |
20 |
60 |
75 |
2 |
1.0/1.1/1.1 |
Toluene |
20 |
60 |
78 |
3 |
1.0/1.2/1.2 |
Toluene |
20 |
60 |
81 |
4 |
1.0/1.3/1.2 |
Toluene |
20 |
60 |
89 |
5 |
1.0/1.2/1.3 |
Toluene |
20 |
60 |
83 |
6 |
1.0/1.3/1.3 |
Toluene |
20 |
60 |
89 |
7 |
1.0/1.4/1.2 |
Toluene |
20 |
60 |
89 |
8 |
1.0/1.3/1.2 |
Water |
20 |
60 |
<10 |
9 |
1.0/1.3/1.2 |
Ethanol |
20 |
60 |
<10 |
10 |
1.0/1.3/1.2 |
DMF |
20 |
60 |
n.d. |
11 |
1.0/1.3/1.2 |
DMSO |
20 |
60 |
n.d. |
12 |
1.0/1.3/1.2 |
MeCN |
20 |
60 |
63 |
13 |
1.0/1.3/1.2 |
THF |
20 |
60 |
52 |
14 |
1.0/1.3/1.2 |
— |
20 |
60 |
<10 |
15 |
1.0/1.3/1.2 |
1,4-Dioxane |
20 |
60 |
96 |
16 |
1.0/1.3/1.2 |
1,4-Dioxane |
15 |
60 |
96 |
17 |
1.0/1.3/1.2 |
1,4-Dioxane |
12.5 |
60 |
89 |
18 |
1.0/1.3/1.2 |
1,4-Dioxane |
10 |
60 |
76 |
19 |
1.0/1.3/1.2 |
1,4-Dioxane |
15 |
40 |
83 |
20 |
1.0/1.3/1.2 |
1,4-Dioxane |
15 |
RT |
51 |
21 |
1.0/1.3/1.2 |
1,4-Dioxane |
15 |
>80 |
79 |
Table 3 Coupling of o-hydroxybenzaldehydes, secondary amines and aryl/heteroaryl boronic acid catalyzed by TNT (15 mg) in 1,4-dioxanea
Entry |
Aldehyde (1a–d) |
Amine (2a–e) |
Boronic acid (3a–d) |
Product (4a–r) |
Time (min) |
Yieldb (%) |
Reaction conditions: O-hydroxybenzaldehyde (1 mmol), secondary amine (1.3 mmol), boronic acid (1.2 mmol), TNT (15 mg), 1,4-dioxane (2 mL) and at 60 °C. Isolated yield. |
1 |
 |
 |
 |
 |
60 |
96 |
2 |
 |
 |
 |
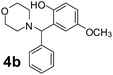 |
50 |
93 |
3 |
 |
 |
 |
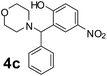 |
65 |
88 |
4 |
 |
 |
 |
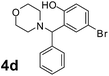 |
60 |
90 |
5 |
 |
 |
 |
 |
45 |
98 |
6 |
 |
 |
 |
 |
50 |
97 |
7 |
 |
 |
 |
 |
60 |
90 |
8 |
 |
 |
 |
 |
55 |
87 |
9 |
 |
 |
 |
 |
60 |
96 |
10 |
 |
 |
 |
 |
50 |
85 |
11 |
 |
 |
 |
 |
50 |
93 |
12 |
 |
 |
 |
 |
60 |
89 |
13 |
 |
 |
 |
 |
60 |
97 |
14 |
 |
 |
 |
 |
65 |
89 |
15 |
 |
 |
 |
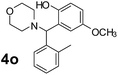 |
90 |
87 |
16 |
 |
 |
 |
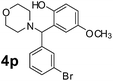 |
55 |
94 |
17 |
 |
 |
 |
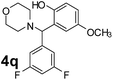 |
60 |
87 |
3. Results and discussion
3.1. Morphology, crystal structure and surface properties of Ti–O based catalysts
HR-TEM image of TNT catalyst (Fig. 1a) showed a bundle of random aligned open ended nanotubes having length between 200 to 500 nm. The TEM image of single nanotube displays (Fig. 1b) cylindrical in shape hollow inside, have four layers on the wall, the inner and outer diameters of the tubes have 3–5 nm and 8–12 nm, respectively. Fig. S1 (ESI†) shows the HR-TEM images of TiO2 fine particles (TFP), TiO2 nanospheres (TNP), TNR, and cTNT. The catalysts TFP and TNP displayed spherical particles with irregular shapes and dark spots observed due to overlapping of particles with each other. The particle size of TFP and TNP measures in the range of 60–160 nm and 23–28 nm, respectively. TNR catalyst showed the random alignment of rods and varied in length of rod >1 μm. The image of single nanorod confirms the clean surface completely filled inside. The calcined material cTNT displayed fragmented tubes, rods and particles at nanoscale which are laying one over another.
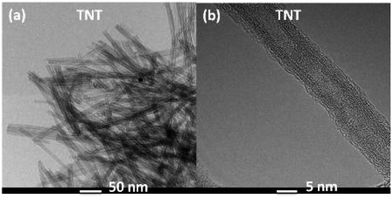 |
| Fig. 1 HR-TEM images of TNT catalyst. | |
The XRD pattern of TNT and TNR catalysts (Fig. 2) showed four characteristic peaks at 2θ = 10.2° (200), 24.1° (202), 28.3° (112) and 48.2° (303), which can be well indexed as the monoclinic structure of layered H2Ti3O7 (JCPDS no. 47-0561). A characteristic diffraction peak exhibited at 10.2° illustrated the presence of typical layered crystal structure. The broad diffraction pattern of TNT and TNR suggests the moderate crystallinity of the catalysts. The XRD pattern of TFP, TNP and cTNT catalysts (Fig. S2, ESI†) showed characteristic diffraction peaks centred at 2θ = 25.4° (101), 37.5° (004), 48.3° (200), 53.9° (105) and 55.1° (211) can be well indexed as anatase phase with tetragonal structure (JCPDS no. 21-1272). In the case of TNP and cTNT catalysts a minor peak at 2θ = 27.5° (110) appeared which confirms the presence of rutile-phase with tetragonal structure (JCPDS no. 21-1276) in addition to anatase phase.
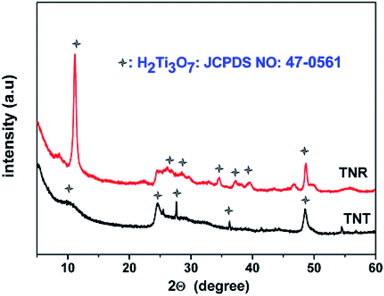 |
| Fig. 2 XRD patterns of TNT and TNR catalysts. | |
The N2-adsorption–desorption isotherms of TNT and cTNT (Fig. 3) showed typical type IV isotherms with a hysteresis loop at relative low-pressure P/P0 ≥ 0.8. According to the IUPAC classification, it confirms the mesoporous nature of TNT and cTNT.12 Surface area of the catalysts were found to decrease in the following order TNT > cTNT > TNP > TNR > TFP. The standard TiO2 nanospheres showed surface area 51 m2 g−1 is consistent with literature report.13 The surface area of TFP is 5.1 m2 g−1 which can be used as a precursor for the preparation of TNT and TNR. The BET surface area of as-synthesized TNT and TNR is 286 and 24 m2 g−1 respectively, which is ∼56 and 4 folds higher than that of TFP. The surface area of cTNT catalyst was declined to 57 m2 g−1. The above results indicate that TNT has porous and nanotubular morphology resulting in a high surface area that leads to a large volume of nitrogen adsorption both on the interior and exterior of the tubes. Fig. S3 (ESI†) shows the N2 adsorption–desorption isotherms TNP and TNR.
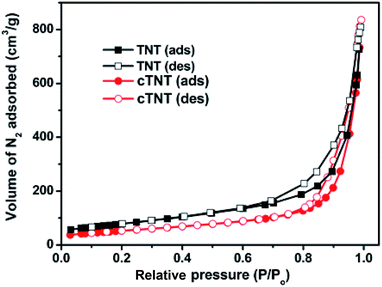 |
| Fig. 3 N2-adsorption–desorption isotherms of TNT and cTNT catalysts. | |
The pore size distribution curve of TNT by (Fig. 4) the BJH method consists of two portions with peak values of ca. 2.1–4.2 nm and 4.2–23 nm. Taking into the account of the morphology of TNT, the smaller pores relates to the inner hollow spaces of the nanotubes; while, the larger pores refers to the pores between the nanotubes.14 The cTNT exhibits pore with a larger size than TNT due to space between the deformed tubes, rods, and particles (Fig. 4). The pore size distribution curves of TNP and TNR (Fig. S4, ESI†) represents that there were no significant distribution peaks were observed for pores hence these catalysts are non-porous materials.
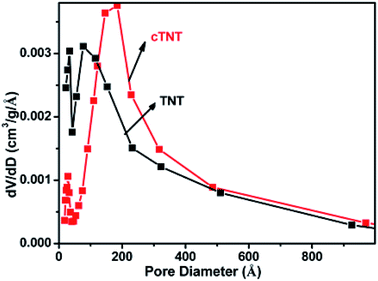 |
| Fig. 4 BJH pore size distributions of TNT and cTNT catalysts. | |
The Brønsted and Lewis acid sites on the catalysts were examined by probing desorption spectra of adsorbed ammonia using temperature programmed desorption analysis (TPD) (Fig. 5). The peak at high temperature region of TNT and TNR (302 °C for TNT, 326.1 °C for TNR)15 and peak at 276.8 °C for TNP and 288 °C for TFP were observed (except cTNT), it is ascribed to Lewis acid sites of coordinatively unsaturated Ti4+ sites present on the surface of the catalysts. Another peak noticed at the low-temperature region of TNT and TNR (160.9 °C for TNT and 148.8 °C for TNR)16 which is assigned to Brønsted acid sites on TNT and TNR catalysts. This is attributed to linear Ti–OH and bridged Ti–OH–Ti sites present in TNT and TNR. In the case of cTNT, calcination at 400 °C for 5 h induced the dehydration (loss of surface –OH groups) accompanied by phase transformation to anatase–rutile biphasic material besides partial collapse of nanotubes to particles leading to a poor concentration of acid sites. The concentration of acid sites of catalysts are depicted in Table 1.
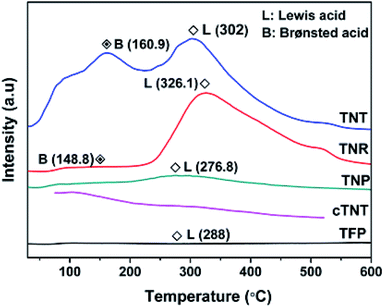 |
| Fig. 5 NH3-TPD of TFP, TNP, TNR, TNT and cTNT catalysts. | |
3.2. Catalytic activity
To develop an optimal catalyst for PBM reaction from Ti–O based catalysts, the synthesis of 2-(morpholino(phenyl)methyl) phenol (4a) via a three-component reaction of salicylaldehyde (1a), morpholine (2a) and phenylboronic acid (3a) and the reaction was conducted in toluene at 60 °C. From Fig. 6 it is clearly evidenced that, the TFP and cTNT catalysts were displayed negligible catalytic activity whereas the catalytic activities of TNP and TNR were found to be moderate. In contrast to the catalytic activity of TFP, TNP, TNR and cTNT; the TNT exhibited significant catalytic performance with 75% of yield in 60 min and with an increase in reaction time, no improvement in the product yield was observed. However, the reaction did not occur in the absence of the catalyst.
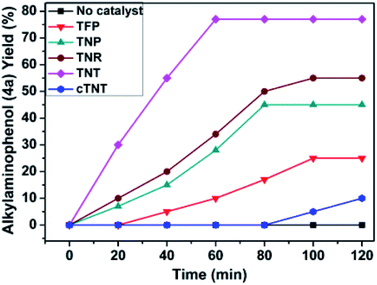 |
| Fig. 6 Time courses of the alkylaminophenol (4a) formation using various Ti–O based catalysts. Reaction conditions: catalyst (20 mg), salicylaldehyde (1 mmol), morpholine (1 mmol), phenylboronic acid (1 mmol), toluene (2 mL) and at 60 °C. | |
Catalytic performance of Ti–O based catalysts is explained in terms of the concentration of acid sites, surface area and density of –OH groups present in catalyst systems (Table 1). Both TFP and TNP mainly composed of anatase phase, the larger surface area and a higher concentration of acid sites of TNP beneficial for obtaining a higher yield than TFP. The higher catalytic activity of TNR than TNP is ascribed to the presence of –OH groups on 1-D morphology, less agglomeration and more acid sites even though the surface area of TNR is nearly 50% lower. Although, TNT catalyst has similar crystalline structure and morphology however due to the presence of hollow inner space in TNT possess higher surface area than TNR itself. Surface area, Brønsted and Lewis acid sites concentration of TNT is about 11, 35 and 2 folds, respectively higher than TNR and the presence of large amount of –OH groups in TNT enhances the catalytic activity of TNT. The cTNT catalyst showed lowest reaction yield due to the absence of acid sites and surface –OH groups. It is explained that concentration of acid sites and amount of –OH groups is extremely important than the surface area of the same type of catalyst. Literature reports also illustrate that acid catalysts promote the PBM reaction effectively.2i,4c,d,5a
On the basis of this encouraging results, efforts were made to optimize the conditions (like the ratio of the substrates, solvents, catalyst loading and temperatures) to attain the best possible yield. Investigation on the ratio of reactants indicates-that a 1.0
:
1.3
:
1.2 ratio of 1a/2a/3a gave the best result with an isolated yield 89% (Table 2, entry 4). In the present study, use of 1.3 eq. of amines and 1.2 eq. boronic acids has specific advantage i.e. o-hydroxy aldehyde conversion was increased. These excess amines and boronic acids were conveniently removed by simple acid–base work up to avoid the usage of column separation.4f Subsequently, solvent screening showed that 1,4-dioxane provided an improved yield of 96% (Table 2, entry 15), while MeCN, THF were less effective and afforded the product in 63% and 59% yields, respectively (Table 2, entries 12 and 13). Replacement of toluene with other solvents, such as water, ethanol, DMF, DMSO and solvent free conditions, either delivered quite low yields (Table 2, entries 8, 9 and 14) or gave no products (Table 2, entries 10 and 11). Further, reducing the amount of catalyst to 10 mg (Table 2, entry 18) down from 20 mg (Table 2, entry 14), had a negative effect on the yield of the reaction. A compromise of 15 mg (Table 2, entry 15), however, seemed to be sufficient. Likewise, reduction in temperature of the reaction also seemed to have a deleterious effect on the yield (Table 2, entries 19 and 20). Further elevating the reaction temperature to 80 °C led to decrease in yield (Table 2, entry 21) (possibly because of the volatility of the amine employed). As can be seen, from Table 2 the best conditions for the synthesis of 4a catalyzed by TNT is entry 16.
Using the optimized reaction conditions, a variety of structurally different salicylaldehydes, secondary amines, and boronic acids were employed as reaction substrates, to explore the scope and generality of the TNT promoted PBM reaction. The results are outlined in Table 3. At the beginning of the investigation to the salicylaldehydes substrate scope, morpholine, and phenylboronic acid were used as model substrates and salicylaldehyde; 5-OCH3/5-NO2/5-Br substituted salicylaldehydes were examined for the PBM reaction (Table 3, entries 1–4). The results indicate that no significant changes in the yields were observed with different substituents on salicylaldehyde. In contrast, the reaction with benzaldehyde; 3-OH/2-OCH3/2-Cl substituted benzaldehydes were failed to participate in this reaction it indicates the importance of ortho hydroxy group on the aldehyde fragment. To expand the scope of secondary amine substrates, a combination of salicylaldehyde–phenyboronic acid-secondary amine was chosen and various amines were examined (Table 3, entries 1 & 5–7). The results indicate that cyclic (pyrrolidine/morpholine) and acyclic (diethyl/N-benzylmethyl) secondary amines gave excellent yields of products under the standard reaction conditions (Table 3, entries 1 and 5–7). However, no product was observed when diphenyl/benzyl amine as substrates and trace amount of product was obtained with a diisopropyl amine. Subsequently, a variety of boronic acids were also examined for the PBM reaction using salicylaldehyde and morpholine as model substrates (Table 3, entries 1 & 8–11). The reaction proceeded smoothly with phenylboronic acid and 2-Me/3-Br/3,5-di-F substituted phenyl boronic acids and gave the corresponding alkylamino phenol in good yield (Table 3, entries 1 & 8–10). In the case of 2,3-dihydrobenzofuran-5-ylboronic acid (Table 1, entry 11), 93% product yield was obtained. No desired product was observed when employed furan-3-ylboronic acid and pyridin-3-ylboronic acid.17
The applicability of optimized reaction conditions was further extended to the synthesis of novel 2-(pyrimidin-2-yl)-2,5-diazabicyclo[2.2.1]-heptane bearing alkylaminophenols (9a–h) using as synthesized 2-(pyrimidin-2-yl)-2,5-diazabicyclo[2.2.1]-heptane (8), o-hydroxybenzaldehydes and boronic acids. 2-(Pyrimidin-2-yl)-2,5-diazabicyclo[2.2.1]-heptane (8) can be synthesized from tert-butyl 2,5-diazabicyclo[2.2.1]-heptane-2-carboxylate hydrochloride (5) and 2-chloropyrimidine (6) in the presence of triethylamine in toluene at reflux temperatures for five hours to form tert-butyl-5-(pyrimidin-2-yl)-2,5-diaza-bicyclo[2.2.1]-heptane-2-carboxylate (7) and subsequently deprotection of tert-butyl carboxylate group of 7 with dioxane–HCl in dichloromethane (Scheme 2) (Table 4).
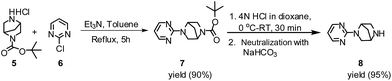 |
| Scheme 2 Synthesis of 2-(pyrimidin-2-yl)-2,5-diazabicyclo[2.2.1]-heptane. | |
Table 4 Coupling of o-hydroxybenzaldehydes, 2-(pyrimidin-2-yl)-2,5-diazabicyclo[2.2.1]-heptane and aryl/heteroaryl boronic acid catalyzed by TNT (15 mg) in 1,4-dioxanea
Entry |
Aldehyde (1a, b) |
Boronic acid (3a, b & 3f–h) |
Product (9a–h) |
Time (min) |
Yieldb (%) |
Reaction conditions: O-hydroxybenzaldehyde (1 mmol), 2-(pyrimidin-2-yl)-2,5-diazabicyclo[2.2.1]-heptane (1.3 mmol), boronic acid (1.2 mmol), TNT (15 mg), 1,4-dioxane (2 mL) and at 60 °C. Isolated yield. |
1 |
 |
 |
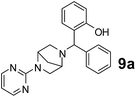 |
90 |
81 |
2 |
 |
 |
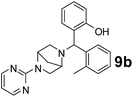 |
120 |
79 |
3 |
 |
 |
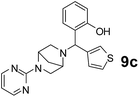 |
90 |
82 |
4 |
 |
 |
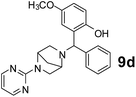 |
120 |
79 |
5 |
 |
 |
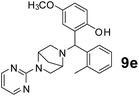 |
180 |
75 |
6 |
 |
 |
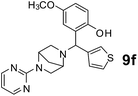 |
120 |
75 |
7 |
 |
 |
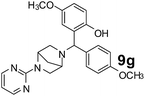 |
150 |
80 |
8 |
 |
 |
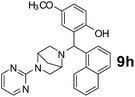 |
180 |
70 |
From these results, a plausible mechanism is proposed for the process of TNT catalyzed PBM reaction. Initially, a nucleophilic addition of the amine to o-hydroxy aldehyde produced the key iminium intermediate (4). Co-ordination between the oxygen anion of phenolate and the boron atom of boronic acid lead to the formation of a tetracoordinate borate (5) intermediate. Subsequently, the aryl carbanion moiety of boronic acid favorably attacked the iminium ion. Finally, hydrolysis took place to form the desired product (6) by the loss of a boric acid (Fig. 7).
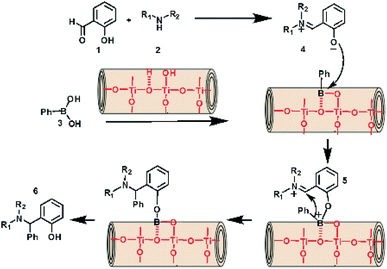 |
| Fig. 7 Plausible mechanism for TNT catalyzed PBM reaction. | |
For practical applications of heterogeneous catalysts, the level of reusability is a very important factor. The recyclability of TNT catalyst was investigated in the coupling reaction of salicylaldehyde, morpholine, and phenylboronic acid. After each run, the TNT catalyst was separated by centrifugation, washed twice with EtOAc and dried in an oven at 100 °C for 6 h. The recovered TNT catalyst was reused for five times in this PBM reaction and smooth loss of activity was observed from the 4th cycle (Table 5). It may be due to the decrease in concentration of acid sites on the catalyst surface. Which is confirmed by NH3-TPD experiment, the concentration of Brønsted and Lewis acid sites of TNT after the 5th cycle was found to be 0.826 and 1.721 mmol g−1, respectively (Fig. 8).
Table 5 TNT catalyst recycling studies in the coupling of salicylaldehyde, morpholine, and phenylboronic acida
Run |
1 |
2 |
3 |
4 |
5 |
Reaction conditions: salicylaldehyde (1 mmol), morpholine (1.3 mmol), phenylboronic acid (1.2 mmol), TNT (15 mg), 1,4-dioxane (2 mL), at 60 °C and for 60 min. Isolated yield. |
Yieldb (%) |
96 |
96 |
96 |
93 |
86 |
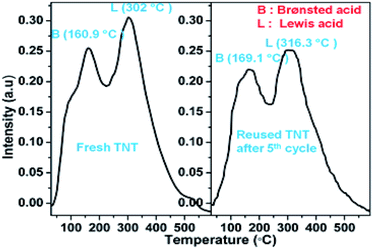 |
| Fig. 8 NH3-TPD profile of (a) fresh TNT and (b) reused TNT after the fifth cycle. | |
4. Conclusions
We demonstrated here that PBM reaction can run in the presence of Ti–O based solid–acid catalysts. Among them, H2Ti3O7 nanotubes showed the remarkable catalytic performance due to the presence of sufficient Brønsted and Lewis acid sites and –OH groups on the large surface area of nanotubes. This method offers several advantages including: (1) good to excellent yields for a variety of substrates in short reaction times, (2) simple work-up procedure (not required column purification), (3) catalyst is readily prepared from available low cost starting materials, (4) catalyst has very good stability in air, moisture and elevated temperatures and (5) high recoverability and the reusability of the catalyst. Further, it is worthy to mention that first time we have shown the synthesis of alkylaminophenols from 2-(pyrimidin-2-yl)-2,5-diazabicyclo[2.2.1]-heptane.
Acknowledgements
Financial support from Department of Atomic Energy, Board of Research in Nuclear Sciences (2012/37C/33/BRNS), Mumbai, India and Department of science and technology (SR/FT/CS-013/2010/DST), New Delhi, India is gratefully acknowledged. B. R. P. Reddy thanks to the CSIR-UGC, New Delhi, India for the award of Junior Research Fellowship. Authors thankful to co-ordinator, DST-FIST, Department of Physics, YV University for providing XRD facility. Authors are thankful to Dr M. Karthik and Dr V. Roddatis of CIC ENERGIGUNE, Spain for their support in the characterization of catalysts.
References
-
(a) N. Kumagai, G. Muncipinto and S. L. Schreiber, Angew. Chem., Int. Ed., 2006, 45, 3635–3638 CrossRef CAS PubMed;
(b) G. Muncipinto, T. Kaya, J. A. Wilson, N. Kumagai, P. A. Clemons and S. L. Schreiber, Org. Lett., 2010, 12, 5230–5233 CrossRef CAS PubMed;
(c) T. E. Nielsen and S. L. Schreiber, Angew. Chem., Int. Ed., 2008, 47, 48–56 CrossRef CAS PubMed.
-
(a) P. Ghosal and A. K. Shaw, J. Org. Chem., 2012, 77, 7627–7632 CrossRef CAS PubMed;
(b) A. Rajender and B. V. Rao, Tetrahedron Lett., 2013, 54, 2329–2331 CrossRef CAS;
(c) M. Chrzanowska, A. Grajewska, Z. Meissner, M. Rozwadowska and I. Wiatrowska, Tetrahedron, 2012, 68, 3092–3097 CrossRef CAS;
(d) S. Noushini, M. Mahdavi, L. Firoozpour, S. Moghimi, A. Shafiee and A. Foroumadi, Tetrahedron, 2015, 71, 6272–6275 CrossRef CAS;
(e) M. Ayaz, J. Dietrich and C. Hulme, Tetrahedron Lett., 2011, 52, 4821–4823 CrossRef CAS PubMed;
(f) P. G. Cornier, C. M. L. Delpiccolo, D. B. Boggian and E. G. Mata, Tetrahedron Lett., 2013, 54, 4742–4745 CrossRef CAS;
(g) P. F. Kaiser, Q. I. Churches and C. A. Hutton, Aust. J. Chem., 2007, 60, 799–810 CrossRef CAS;
(h) N. A. Petasis and I. A. Zavialov, J. Am. Chem. Soc., 1997, 119, 445–446 CrossRef CAS;
(i) Y. Li and M. H. Xu, Org. Lett., 2012, 14, 2062–2065 CrossRef CAS PubMed;
(j) T. Koolmeister, M. Sodergren and M. Scobie, Tetrahedron Lett., 2002, 43, 5965–5968 CrossRef CAS;
(k) H. Jourdan, G. Gouhier, L. V. Hijfte, P. Angibaud and S. R. Piettre, Tetrahedron Lett., 2005, 46, 8027–8031 CrossRef CAS;
(l) N. A. Petasis and I. Akritopoulou, Tetrahedron Lett., 1993, 34, 583–586 CrossRef CAS;
(m) Q. Wang and M. G. Finn, Org. Lett., 2000, 2, 4063–4065 CrossRef CAS PubMed;
(n) G. W. Kabalka, B. Venkataiah and B. C. Das, Synlett, 2004, 2194–2196 CrossRef CAS;
(o) N. A. Petasis and A. N. Butkevich, J. Organomet. Chem., 2009, 694, 1747–1753 CrossRef CAS PubMed;
(p) J. Wang, P. Li, Q. Shen and G. Song, Tetrahedron Lett., 2014, 55, 3888–3891 CrossRef CAS;
(q) K. J. Koroluk, D. A. Jackson and A. P. Dicks, J. Chem. Educ., 2012, 89, 796–798 CrossRef CAS;
(r) L. Chouguiat, R. Boulcina, B. Carboni, A. Demonceau and A. Debache, Tetrahedron Lett., 2014, 55, 5124–5128 CrossRef CAS;
(s) S. Neogi, A. Roy and D. Naskar, J. Comb. Chem., 2010, 12, 617–629 CrossRef CAS PubMed;
(t) D. A. Mundal, K. E. Lutz and R. J. Thomson, J. Am. Chem. Soc., 2012, 134, 5782–5785 CrossRef CAS PubMed;
(u) H. Feng, H. Jia and Z. Sun, J. Org. Chem., 2014, 79, 11812–11818 CrossRef CAS PubMed;
(v) S. T. L. Quement, T. Flagstad, R. J. T. Mikkelsen, M. R. Hansen, M. C. Givskov and T. E. Nielsen, Org. Lett., 2012, 14, 640–643 CrossRef PubMed;
(w) C. X. Cui, H. Li, X. J. Yang, J. Yang and X. Q. Li, Org. Lett., 2013, 15, 5944–5947 CrossRef CAS PubMed;
(x) N. A. Petasis and I. A. Zavialov, J. Am. Chem. Soc., 1998, 120, 11798–11799 CrossRef CAS;
(y) G. K. S. Prakash, M. Mandal, S. Schweizer, N. A. Petasis and G. A. Olah, Org. Lett., 2000, 2, 3173–3176 CrossRef CAS PubMed;
(z) T. Regnier, F. Berree, O. Lavastre and B. Carboni, Green Chem., 2007, 9, 125–126 RSC;
(a
a) F. Berree, A. Debache, Y. Marsac, B. Collet, P. G. L. Bleiz and B. Carboni, Tetrahedron, 2006, 62, 4027–4037 CrossRef CAS;
(a
b) D. E. Portlock, D. Naskar, L. West and M. Li, Tetrahedron Lett., 2002, 43, 6845–6847 CrossRef CAS;
(a
c) N. A. Petasis, A. Goodman and I. A. Zavialov, Tetrahedron, 1997, 53, 16463–16470 CrossRef CAS;
(a
d) D. Font, M. Heras and J. M. Villalgordo, Tetrahedron, 2008, 64, 5226–5235 CrossRef CAS;
(a
e) N. J. McLean, H. Tye and M. Whittaker, Tetrahedron Lett., 2004, 45, 993–995 CrossRef CAS.
- N. A. Petasis and S. Boral, Tetrahedron Lett., 2001, 42, 539–542 CrossRef CAS.
-
(a) R. Frauenlob, C. Garcia, G. A. Bradshaw, H. M. Burke and E. Bergin, J. Org. Chem., 2012, 77, 4445–4449 CrossRef CAS PubMed;
(b) R. Frauenlob, C. Garcia, S. Butler and E. Bergin, Appl. Organomet. Chem., 2014, 28, 432–435 CrossRef CAS;
(c) S. Stas and K. A. Tehrani, Tetrahedron, 2007, 63, 8921–8931 CrossRef CAS;
(d) T. Beisel and G. Manolikakes, Org. Lett., 2013, 15, 6046–6049 CrossRef CAS PubMed;
(e) X. Shi, D. Hebrult, M. Humora, W. F. Kiesman, H. Peng, T. Talreja, Z. Wang and Z. Xin, J. Org. Chem., 2012, 77, 1154–1160 CrossRef CAS PubMed;
(f) A. M. Kulkarni, K. S. Pandit, P. V. Chavan, U. V. Desai and P. P. Wadgaonkar, RSC Adv., 2015, 5, 70586–70594 RSC.
-
(a) S. S. Reddy, B. R. P. Reddy and P. V. G. Reddy, Tetrahedron Lett., 2015, 56, 4984–4989 CrossRef CAS;
(b) T. Rosholm, P. M. P. Gois, R. Franzen and N. R. Candeias, ChemistryOpen, 2015, 4, 39–46 CrossRef CAS PubMed;
(c) J. S. Yadav, B. V. Subba Reddy and P. N. Lakshmi, J. Mol. Catal. A: Chem., 2007, 274, 101–104 CrossRef CAS;
(d) M. Follmann, F. Gaul, T. Schafer, S. Kopec and P. Hamley, Synlett, 2005, 1009–1011 CrossRef CAS.
-
(a) N. R. Candeias, L. F. Veiros, C. A. M. Afonso and P. M. P. Gois, Eur. J. Org. Chem., 2009, 1859–1863 CrossRef CAS;
(b) N. R. Candeias, P. M. S. D. Cal, V. Andre, M. T. Duarte, L. F. Veiros and P. M. P. Gois, Tetrahedron, 2010, 66, 2736–2745 CrossRef CAS;
(c) L. Ying, W. Limin, S. Yuanyuan and Y. Jianjun, Chin. J. Chem., 2010, 28, 2039–2044 CrossRef;
(d) S. R. Klopfenstein, J. J. Chen, A. Golebiowski, M. Li, S. X. Peng and X. Shao, Tetrahedron Lett., 2000, 41, 4835–4839 CrossRef CAS;
(e) A. Golbiowski, S. R. Klopfenstein, J. J. Chen and X. Shao, Tetrahedron Lett., 2000, 41, 4841–4844 CrossRef.
-
(a) A. Dandia, V. Parewa, S. L. Gupta, A. Sharma, K. S. Rathore, A. Sharma and A. Jain, Catal. Commun., 2015, 61, 88–91 CrossRef CAS;
(b) S. Bajpai, S. Singh and V. Srivastava, RSC Adv., 2015, 5, 28163–28170 RSC;
(c) F. Nemati, A. Elhampour, H. Farrokhi and M. B. Natanzi, Catal. Commun., 2015, 66, 15–20 CrossRef CAS;
(d) E. Mosaddegh and A. Hassankhani, Catal. Commun., 2015, 71, 65–69 CrossRef CAS;
(e) A. Farrokhi, K. Ghodrati and I. Yavari, Catal. Commun., 2015, 63, 41–46 CrossRef CAS;
(f) R. Yang, T. Tao, Y. Dai, Z. Chen, X. Zhang and Q. Song, Catal. Commun., 2015, 60, 96–99 CrossRef CAS.
-
(a) M. Kitano, K. Nakajima, J. N. Kondo, S. Hayashi and M. Hara, J. Am. Chem. Soc., 2010, 132, 6622–6623 CrossRef CAS PubMed;
(b) M. Kitano, E. Wada, K. Nakajima, S. Hayashi, S. Miyazaki, H. Kobayashi and M. Hara, Chem. Mater., 2013, 25, 385–393 CrossRef CAS;
(c) E. Wada, M. Kitano, K. Nakajima and M. Hara, J. Mater. Chem. A, 2013, 1, 12768–12774 RSC;
(d) M. L. Kantam, S. Laha, J. Yadav and B. Sreedhar, Tetrahedron Lett., 2006, 47, 6213–6216 CrossRef CAS;
(e) M. L. Kantam, S. Laha, J. Yadav, B. M. Choudary and B. Sreedhar, Adv. Synth. Catal., 2006, 348, 867–872 CrossRef CAS;
(f) S. M. Baghbanian, M. Farhang and R. Baharfar, Chin. Chem. Lett., 2011, 22, 555–558 CrossRef CAS;
(g) M. H. Sarvari, Tetrahedron, 2008, 64, 5459–5466 CrossRef;
(h) M. Z. Kassaee, H. Masrouri, F. Movahedi and R. Mohammadi, Helv. Chim. Acta, 2010, 93, 261–264 CrossRef CAS;
(i) S. De, S. Dutta, A. K. Patra, A. Bhaumik and B. Saha, J. Mater. Chem., 2011, 21, 17505–17510 RSC;
(j) S. S. Prasad, S. H. Jayaprakash, K. U. Rao, N. B. Reddy, P. C. R. Kumar and C. S. Reddy, Org. Commun., 2014, 7, 98–105 CAS;
(k) R. Saladino, U. Ciambecchini, C. Crestini, G. Costanzo, R. Negri and E. D. Mauro, ChemBioChem, 2003, 4, 514–521 CrossRef CAS PubMed;
(l) S. Rana, M. Brown, A. Dutta, A. Bhaumik and C. Mukhopadhyay, Tetrahedron Lett., 2013, 54, 1371–1379 CrossRef CAS;
(m) R. Sarkar and C. Mukhopadhyay, Tetrahedron Lett., 2013, 54, 3706–3711 CrossRef CAS;
(n) A. Amoozaadeh, S. Golian and S. Rahmani, RSC Adv., 2015, 5, 45974–45982 RSC.
-
(a) T. Kasuga, M. Hiramatsu, A. Hoson, T. Sekino and K. Niihara, Langmuir, 1998, 14, 3160–3163 CrossRef CAS;
(b) X. Chen and S. S. Mao, Chem. Rev., 2007, 107, 2891–2959 CrossRef CAS PubMed;
(c) C. N. R. Rao, A. Govindaraj, F. L. Deepak, N. A. Gunari and M. Nath, Appl. Phys. Lett., 2001, 78, 13–26 CrossRef.
-
(a) S. H. Kang, S. H. Choi, M. Kang, J. Y. Kim, H. S. Kim, T. Hyeon and Y. E. Sung, Adv. Mater., 2008, 20, 54–58 CrossRef CAS;
(b) H. Zhu and Q. Li, Nanoscale Res. Lett., 2013, 8, 230–235 CrossRef PubMed;
(c) D. P. Kumar, M. V. Shankar, M. M. Kumari, G. Sadanandan, B. Srinivas and V. D. Kumari, Chem. Commun., 2013, 49, 9443–9446 RSC;
(d) D. P. Kumar, N. L. Reddy, M. V. Shankar, M. M. Kumari, B. Srinivas and V. D. Kumari, J. Catal. Catal., 2014, 1, 13–20 Search PubMed;
(e) D. P. Kumar, N. L. Reddy, M. M. Kumari, B. Srinivas, V. D. Kumari, V. Roddatis, O. Bondarchunk, M. Karthik, B. Nepplian and M. V. Shankar, Sol. Energy Mater. Sol. Cells, 2015, 136, 157–165 CrossRef;
(f) M. M. Kumari, D. P. Kumar, P. Haridoss, V. D. Kumari and M. V. Shankar, Int. J. Hydrogen Energy, 2015, 40, 1665–1674 CrossRef;
(g) D. P. Kumar, N. L. Reddy, B. Srinivas, V. D. Kumari, V. Roddatis, O. Bondarchunk, M. Karthik, Y. Ikuma and M. V. Shankar, Sol. Energy Mater. Sol. Cells, 2016, 146, 63–71 CrossRef;
(h) T. Sree Latha, L. Dakshayani, D. Praveen Kumar, M. V. Shankar and C. Madhava Reddy, RSC Adv., 2016, 6, 8870–8880 RSC.
-
(a) B. R. P. Reddy, P. V. G. Reddy and B. N. Reddy, New J. Chem., 2015, 39, 9605–9610 RSC;
(b) B. R. P. Reddy, M. V. K. Reddy, P. V. G. Reddy, D. P. Kumar and M. V. Shankar, Tetrahedron Lett., 2016, 57, 696–702 CrossRef CAS.
-
(a) C. Liao, H. P. Wang and H. P. Lin, Int. J. Photoenergy, 2013, 2013, 1–7 CrossRef;
(b) R. Doong, S. Chang and C. Tsai, Appl. Catal., B, 2013, 129, 48–55 CrossRef CAS.
- O. Rosseler, M. V. Shankar, M. Du, L. Schmidlin, N. Keller and V. Keller, J. Catal., 2010, 269, 179–190 CrossRef CAS.
-
(a) D. V. Bavykin, V. N. Parmon, A. A. Laokin and F. C. Walsh, J. Mater. Chem., 2004, 14, 3370–3377 RSC;
(b) S. Zu, A. Du, J. Liu, J. Ng and D. D. Sun, Int. J. Hydrogen Energy, 2011, 36, 6560–6568 CrossRef.
-
(a) L. Chmielarz, P. Kustrowski, M. Zbroja, W. Lasocha and R. Dziembaj, Catal. Today, 2004, 90, 43–49 CrossRef CAS;
(b) T. Matsuda, M. Asanuma and E. Kikuchi, Appl. Catal., 1988, 38, 289–299 CrossRef CAS;
(c) C. Gannoun, R. Delaigle, P. Eloy, D. P. Debecker, A. Ghorbel and A. E. Gaigneaux, Catal. Commun., 2011, 15, 1–5 CrossRef CAS;
(d) C. Gannouna, A. Turkia, H. Kochkara, R. Delaiglec, P. Eloyc, A. Ghorbela and E. M. Gaigneauxca, Appl. Catal., B, 2014, 147, 58–64 CrossRef.
-
(a) M. E. Manriquez, T. Lopez, R. Gomez and T. Navarrete, J. Mol. Catal. A: Chem., 2004, 220, 229–237 CrossRef CAS;
(b) A. V. Grigorieva, V. V. Yuschenko, I. I. Ivanova, E. A. Goodilin and Y. D. Tretyakov, J. Nanomater., 2012, 2012, 7 Search PubMed.
- S. Voisin, A. Bouillon, J. C. Lancelot, A. Lesnard, H. Oulyadi and S. Rault, Tetrahedron Lett., 2006, 47, 2165–2169 CrossRef.
Footnote |
† Electronic supplementary information (ESI) available: General experimental details, catalysts characterization, characterization data and copies of the NMR (1H &13C) spectra of all synthesized compounds, HRMS spectra of 4e, 4p, 9a and 9d; and EI-mass spectra of 4a and 4b. See DOI: 10.1039/c5ra25064a |
|
This journal is © The Royal Society of Chemistry 2016 |