DOI:
10.1039/C6RA01074A
(Paper)
RSC Adv., 2016,
6, 81654-81665
Synthesis of a self-healable and pH responsive hydrogel based on an ionic polymer/clay nanocomposite†
Received
13th January 2016
, Accepted 23rd August 2016
First published on 23rd August 2016
Abstract
This investigation reports the preparation of a pH responsive self-healing nanocomposite hydrogel based on the in situ polymerization of 2-(acryloyloxy)ethyl trimethylammonium chloride and acrylic acid using N,N′-methylenebisacrylamide as a crosslinker and ammonium persulfate (APS) as a thermal initiator in the presence of soluble starch and organically modified montmorillonite (OMMT). The hydrogel was characterized using FTIR, SEM and TEM analyses. The influence of different parameters in swelling was investigated. The self-healing ability of the hydrogel was measured in different pH media and it was observed that it can show self-healing ability in physiological pH (pH = 7.4), in acidic pH (pH = 1.2) and in saline solution. This developed hydrogel showed excellent swelling ratio, mechanical strength, and elasticity. The hydrogel exhibited modulus values (G′, G′′) in the range of 102 Pa.
1. Introduction
The design of smart materials that can respond to external stimuli has become an interesting part of research in recent days. Synthetic polymers that can respond to temperature,1 chemicals,2 pH,3 ionic strength,4 magnetic field,5 and an electric field,6 have gathered interest as stimuli responsive smart polymeric systems. These types of stimuli responsive polymers receive attention in the fields of biomedical applications like delivery of therapeutics,7 sensors and actuators,8 and tissue engineering9 due to their change in shape,10 surface characteristics,11 and solubility12 with a change in external stimulus. Apart from stimuli responsive characteristics, the polymers should also have self-healing characteristics for many applications. Self-healing is the ability of a material or a system to repair the damaged portion (external or internal) spontaneously and autonomously13 which is generally observed in living systems. Among different self-healing polymeric materials, self-healable hydrogels show promising ability to be used in biomedical applications due to their biocompatibility and similarity with tissue systems. The first reported self-healable material was based on the microencapsulation of a reactive species that could be ruptured during the damage and could polymerize or react with the system to regenerate the actual shape.14 Self-healing hydrogels can be made by using several techniques. The self-healing hydrogels are prepared by using dynamic chemistry that consists of both non-covalent chemistry and covalent chemistry.15 Physical self-healing hydrogels utilize the hydrophobic interactions,16 hydrogen bonding,17 host–guest interaction,18 electrostatic interactions,19 metal coordination,20 π–π stacking21 etc. Self-healing hydrogels based on the dynamic covalent chemistry utilize the external stimuli like pH, temperature, light22,23 etc. to form permanent covalent bonds. So far the main problem with self-healing hydrogel synthesis is that the formed hydrogel suffers from low mechanical strength which is mainly due to the formation of reversible physical bonds. This deficiency in mechanical strength can be improved by forming hybrid network that can be established by introducing covalent network and filler system inside the hydrogel. Haraguchi et al. reported the formation of nanocomposite hydrogel composed of poly(N-isopropylacrylamide) (PNIPAM) and water swellable clay (hectrite), that could show 1000% elongation before break.24 In another work Haraguchi et al. discussed about the self-healing in clay containing nano composite hydrogel where clay acted as a physical crosslinker. They studied the effect of temperature on self-healing as well as mechanical properties of self-healed hydrogel. With increase in temperature, self-healing behavior was improved significantly.25 However, achieving self-healing properties in covalently crosslinked hydrogel is relatively difficult due to the presence of irreversible crosslinks that restricts the movement of the polymer chains which is possible in case of physically crosslinked gel. Another important requirement of self-healed hydrogel is its healing efficiency in physiological pH. Most of the reported self-healable hydrogels show its healing property at acidic pH.26 It will be advantageous if the hydrogels can show self-healing property in both acidic and physiological pH region while maintaining mechanical strength.
In this work, we have developed a covalently crosslinked unique hydrogel system that have pH responsiveness, self-healing capability in acidic as well as physiological pH and high swelling ratio. This hydrogel consists of poly(acrylic acid), poly(2-(acryloyloxy)ethyl trimethylammonium chloride) and soluble starch. In this case organically modified MMT clay was used as reinforcing filler and N,N′-methylenebisacrylamide (MBA) was used as a crosslinking agent. The synthesized hydrogel was characterized by FTIR, FESEM and TEM analyses. The healing effect was confirmed by rheological study of the hydrogel along with elongation study.
2. Materials and methods
2.1. Materials
Starch was purchased from Merck, India and used as received (solubility = 50 g L−1, bulk density 300 kg m−3, pH of 2 wt% water solution is 6–7.5). N,N′-Methylenebisacrylamide (MBA) (crosslinking agent), ammonium persulphate (APS) (thermal initiator) and ethanol (EtOH) were purchased from Merck, India. All are of analytical grade. Acrylic acid (AA) monomer was purchased from Sigma-Aldrich, USA and was purified by vacuum distillation before use. 2-(Acryloyloxy)ethyl trimethylammonium chloride (AETAC) solution (80% in H2O), was purchased from Sigma-Aldrich, USA. Montmorillonite (K-10) (MMT) clay and cetyl trimethylammonium bromide (CTAB) were supplied by Sigma-Aldrich.
2.2. Methods
2.2.1. Synthesis of CTAB modified MMT-nano clay. MMT clay was modified by following the previously used method.27 Initially a measured amount of pristine MMT clay (15 g) was dispersed in 1 L of deionized water followed by addition of CTAB. The whole dispersion was heated at 60 °C for 6 h with constant stirring. After that the entire solution was centrifuged at 8000 rpm for 3 min. The water dispersion of modified MMT was washed repeatedly and filtered using Whatman filter paper until any precipitation came after addition of 0.1 (M) aqueous AgNO3 solution into the filtrate part. The filtered part was dried in vacuum oven at 80 °C for 12 h and ground in a mortar pestle to obtain the fine particle of organically modified MMT (OMMT).
2.2.2. Synthesis of hydrogel without filler. Starch based poly(AA-co-AETAC) hydrogel was prepared by free radical polymerization method in water. Initially poly(AA-co-AETAC) gel was prepared in presence of starch by using APS as a thermal initiator and MBA as a crosslinking agent, at 70 °C in a three-neck 100 mL glass reactor fitted with a constant stirring system. Total monomer concentration was maintained at 20 wt%. In a typical reaction, 2.8 g of AA was mixed with 1.2 g of AETAC in 18 mL of water in presence of 0.4 g (2 wt%) of soluble starch. After that, 0.04 g of MBA (1 wt% w.r.t the monomer, dissolved in 1 mL of water) was added to the system and stirred for homogenization. Then 0.04 g of APS (1 wt% w.r.t the monomer, dissolved in 1 mL of water) was added to the system at 70 °C under N2 atmosphere and in constant stirring condition. Detailed recipe for all the hydrogel synthesis of different compositions was given in Table 1. During the gelation reaction, initially the whole reaction mixture was prepolymerized at 70 °C for 15 min and then it was readily transferred into a cylindrical mould to give particular shape and then the polymerization was completed inside the mould. After the hydrogel formation, the whole sample was taken out from the mould and disintegrated into small cylindrical shapes and immersed in a 1
:
4 (v/v%) ethanol–water mixture for one day under constant stirring to remove the water soluble oligomers, unreacted monomers and other reactants. Then the hydrogel fragments placed inside the vacuum oven at 50 °C for 48 h to complete drying of the sample and the hydrogels were characterized by using different characterization techniques. The gel yield (%) as well as the gel content (%) was also determined.
Table 1 Recipe of hydrogel preparationa,b
Number |
Sample code |
Starch content (wt%) |
AA : AETAC (maintaining 20 wt% total monomer concentration) |
MBA content (wt% w.r.t monomer) |
OMMT content (wt% w.r.t monomer) |
Total batch volume maintained at 20 mL. APS content = 1 wt% with respect to monomer concentration. |
1 |
SBase |
2.0 |
70 : 30 |
1.0 |
0.0 |
2 |
S0 |
2.0 |
70 : 30 |
1.0 |
2.5 |
3 |
S1 |
2.0 |
70 : 30 |
1.0 |
5.0 |
4 |
S2 |
2.0 |
70 : 30 |
1.0 |
7.5 |
5 |
S3 |
2.0 |
70 : 30 |
1.5 |
5.0 |
6 |
S4 |
2.0 |
70 : 30 |
2.0 |
5.0 |
7 |
S5 |
0.0 |
70 : 30 |
1.0 |
5.0 |
8 |
S6 |
3.0 |
70 : 30 |
1.0 |
5.0 |
9 |
S7 |
2.0 |
50 : 50 |
1.0 |
5.0 |
10 |
S8 |
2.0 |
90 : 10 |
1.0 |
5.0 |
2.2.3. Synthesis of OMMT incorporated nanocomposite hydrogel by using in situ filler addition method. Starch based poly(AA-co-AETAC)/OMMT nanocomposite hydrogel was prepared by using the same method as described above by varying the content of starch (0–3 wt% of total monomer amount), OMMT (0–7.5 wt% of total monomer amount), MBA (1–2 wt% of total monomer amount) and acrylic acid (AA) to 2-(acraloyloxy)ethyl trimethylammonium chloride (AETAC) weight ratio to 50
:
50, 70
:
30 and 90
:
10 by maintaining 20 wt% monomer concentration in solution respectively. Before the preparation of the composite hydrogel OMMT dispersion was sonicated for 2 h and then the OMMT contained monomer solution was polymerized in presence of required amount of crosslinker and initiator. Recipe of the nanocomposite hydrogel preparation is shown in Table 1.
2.2.4. Determination of the yield and sol–gel content of the prepared hydrogel. The prepared hydrogel was first dried in vacuum oven to get a constant weight and then the dried insoluble fraction of hydrogel was immersed in DI water and kept it for one week with occasional shaking to remove the water soluble part from the bulk of the gel. After equilibrium swelling, the gels were further dried to a constant weight which had only the water insoluble part. Yield%, gel% and sol% of the hydrogels were determined by using following equations: |
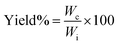 | (1) |
|
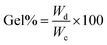 | (2) |
where, Wc = constant weight of the hydrogel after drying, Wd = constant weight of the hydrogel after equilibrium swelling and subsequent drying, Wi = the weight of total monomer taken.
2.2.5. Self-healing and shape retention study of composite hydrogel. To study the self-healing ability of the synthesized hydrogel, a cylindrical hydrogel sample (containing 5 wt% OMMT) was cut into two equal pieces having a dimension of 10 mm in diameter and 20 mm in length. One part of the hydrogel was dyed with rhodamine-B and then these hydrogel fragments were rejoined and placed in separate tightly closed vessel containing a solution of pH = 1.2, pH = 7.4, NaCl solution of 0.154 (M) (physiological saline) and 1 (M) respectively for 12 h at room temperature. After 12 h the rejoined samples were subjected to rheological study using parallel plate rheometer to determine the storage modulus (G′) and loss modulus (G′′) of the uncut and self-healed hydrogel. Elongation study of the self-healed gel containing 5 wt% OMMT was also studied. For shape recovery study the nanocomposite hydrogel of defined thickness of 20 mm was sandwiched between two parallel plates and compressed, so that the gap between the inside wall of the plates was 6 mm. After compression for 10 min the compressive force was withdrawn and the thickness of the hydrogel was measured again. Shape recovery behavior was calculated from the ratio of recovery thickness and original thickness of the sample. In another experiment for shape recovery test, the cylindrical hydrogel sample was curved to near 180° and then the force was removed for recovery.
2.3. Characterizations of the hydrogel
2.3.1. Fourier transform infrared (FTIR) spectroscopy. The as-prepared modified MMT, pure hydrogel and composite hydrogel samples were characterized by using FTIR spectrophotometer (Perkin Elmer, model spectrum-2) at the range of 500–4000 cm−1. In the FTIR experiment we had used 8 scans and 0.5 cm−1 resolution. Fine powder of all of the samples was mixed with KBr at 10
:
1 KBr to sample weight ratio and the pellet was formed by using a hydraulic press.
2.3.2. X-ray diffraction (XRD) analysis. XRD analysis of the sample was carried out on a wide angle XRD (PANalytical X-ray defractometer, model: X'part powder) with Cu Kα radiation (λ = 0.154 nm) in the range of 5–80° (2θ) at 40 kV and 30 mA.
2.3.3. Field emission scanning electron microscopy (FESEM) analysis. The surface morphology of the pure and composite hydrogel was studied using a ZEISS FESEM at an accelerating voltage of 5 kV. EDAX analysis and elemental mapping of the composite hydrogel samples were done using the same machine. The samples in the film form were placed over stub using carbon adhesive tape and coated with gold (thickness = 30 nm) before scanning.
2.3.4. Transmission electron microscopy (TEM) analysis. The distribution of OMMT particles inside the hydrogel was analyzed using the TEM (JEOL, JEM-2000E7) instrument at an accelerating voltage of 200 kV. TEM samples were prepared by cryo-microtomy in which sample of 50 nm thickness was sectioned and placed in copper grid before imaging.
2.3.5. Study of swelling/deswelling behavior of the hydrogel. The swelling ratio of the obtained hydrogel was determined gravimetrically. Presence of physical and chemical crosslinks inside the gel controlled the swelling property of the hydrogel. To observe the swelling behavior, the cylindrical gel fragments were immersed in different solutions having pH value of 1.2 and 7.4 respectively. Swelling behavior of the hydrogel was also observed in salt solutions containing different salts of varying valences and also in DI water. Weight of the hydrogel in different interval of swelling was taken as (Ws) and the swelling ratio was calculated using following equation: |
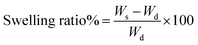 | (4) |
where, Wd was the dry weight of the hydrogel. To calculate the equilibrium swelling ratio (ESR) we have to replace the Ws value with We, which was the weight of the hydrogel in equilibrium swelled condition. Buffer solution of pH = 1.2 was prepared by mixing 0.2 (M) hydrochloric acid (HCl) with 0.2 (M) NaCl solution. Phosphate buffer of pH = 7.4 was prepared by mixing potassium dihydrogen phosphate (KH2PO4), dipotassium hydrogen phosphate (K2HPO4) and approximately 0.136 (M) (8 g in 1000 mL of water) sodium chloride (NaCl) solution. NaOH solution of 0.2 (M) was used to adjust the pH to 7.4. For the swelling study in the salt solution we used different aqueous salt solutions of same molarity like 0.1 (M) NaCl, 0.1 (M) CaCl2 and 0.1 (M) FeCl3.Deswelling behavior of the hydrogel samples was determined by observing the reduction of weight of a fully swollen hydrogel sample in a fixed time interval at 32 °C and 65% relative humidity. After fixed time interval the hydrogel sample was weighed after soaking the surface water with tissue paper.
2.3.6. pH responsiveness study. To study the pH responsiveness of the hydrogels containing different combination of monomers, buffer solutions of different pH were prepared by mixing KH2PO4, K2HPO4, HCl, NaCl and NaOH solutions in required proportions. The pH responsiveness of the hydrogel sample was determined by measuring the swelling–deswelling oscillatory behavior of the hydrogel in basic (pH = 7.4) and acidic (pH = 1.2) medium respectively. The given time interval between each swelling and deswelling cycle was 60 min.
2.3.7. Rheological study. Rheological study of the prepared hydrogel was carried out on an Anton Paar, MCR102SN81194551 parallel plate rheometer in compression mode. In this case the hydrogels were subjected to a shearing test at a strain rate variation of 0.001 to 10% with a constant angular frequency of 1 Hz.
3. Results and discussions
In this investigation we prepared a self-healable starch based poly(AA-co-AETAC)/OMMT composite hydrogel via free radical polymerization using APS as thermal initiator and MBA as crosslinker at 70 °C. We varied the content of starch (0–3 wt% of total monomer amount), OMMT (0–7.5 wt% of total monomer amount), MBA (1.0–2.0 wt% of total monomer amount) and AA to AETAC weight ratio to 50
:
50, 70
:
30 and 90
:
10 by maintaining a 20 wt% total monomer concentration (TMC) in reaction system. During this polymerization reaction two acrylic monomers were copolymerized in presence of starch using MBA as a crosslinker leading to a three dimensional polymer gel, as shown in Scheme 1. These materials were analyzed as explained below.
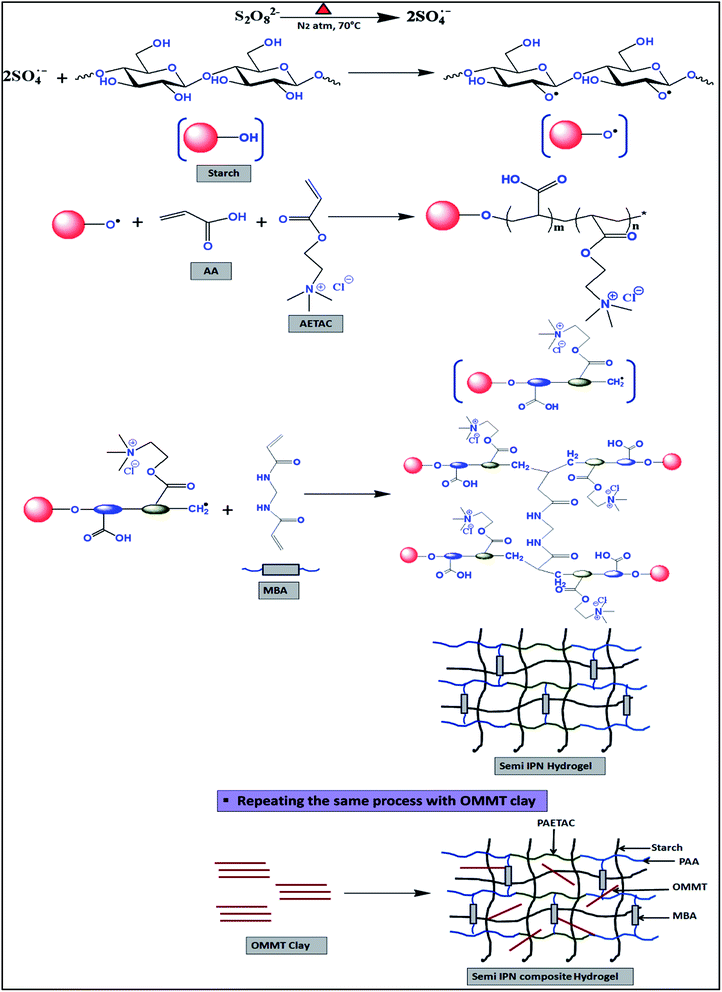 |
| Scheme 1 Preparation of starch based three component self-healing composite hydrogel. | |
3.1. Effect of clay content on gel%
To determine the effect of OMMT content on gel%, the amount of other constituents like starch, MBA, APS and AA
:
AETAC ratio were kept at 2 wt%, 1 wt%, 1 wt% and 70
:
30 maintaining 20 wt% total monomer concentration respectively. From the Table 2 it was observed that the gel% of the prepared composite hydrogel gradually increased with the content of OMMT inside the gel. It might be due to the formation of physical crosslinking between OMMT and polymer chains that affected the 3D network of the hydrogel.
Table 2 Variation in gel% with the change in the amount of OMMT content
Recipe |
SBase |
S0 |
S1 |
S2 |
Starch (wt%) |
2 |
2 |
2 |
2 |
AA : AETAC (maintaining 20 wt% TMC) |
70 : 30 |
70 : 30 |
70 : 30 |
70 : 30 |
MBA (wt%) |
1 |
1 |
1 |
1 |
APS (wt%) |
1 |
1 |
1 |
1 |
OMMT (wt%) |
0 |
2.5 |
5.0 |
7.5 |
Gel% |
79 |
84 |
90 |
95 |
3.2. FTIR analysis
FTIR analysis of MMT, OMMT, pristine starch, pure hydrogel and composite hydrogel was discussed in following section. Fig. S1 (ESI†) showed the FTIR spectra of MMT and modified MMT. FTIR spectrum of CTAB modified MMT showed characteristics absorption band at 1478 cm−1 due to the –CH3 bending vibration of the quaternary ammonium ion [RN(CH3)3]+ of CTAB. The absorption bands at 3630 cm−1 and 3395 cm−1 were due to the presence of free –OH and bound –OH of both OMMT and pristine MMT.28
Fig. 1 represents the FTIR spectrum of the prepared hydrogel and its different components. In case of starch, a broad band centered near 3416 cm−1 was due to the presence of –OH and C–OH stretching, while the absorption band at 2925 cm−1 was due to –CH2 stretching vibration. The C–O–C stretching absorption band was found near 1163 cm−1. These are the characteristics band for starch.29 FTIR spectra for the pure hydrogel showed characteristic peaks at 2925 cm−1, 1731 cm−1 and at 1394 cm−1 for –CH2 stretching, C
O stretching and in-plane deformation of–CH bond of PAA.30 The absorption bands at 1491 cm−1 and 942 cm−1 appeared due to the bending vibration of quaternary ammonium group and stretching vibration of quaternary ammonium group present in the PAETAC moiety in the copolymer. The absorption band of >C
O stretching for PAETAC was merged with >C
O stretching of PAA in copolymeric system. Along with these peaks a significant absorption band was observed at 1560 cm−1 in the FTIR spectra of the hydrogel which was attributed due to the presence of asymmetric deformation of the carboxylated group (–COO−). This indicates that the carboxylic groups of PAA were dissociated and formed a complex with –N(CH3)3+ of PAETAC through electrostatic interaction.31 In case of composite hydrogel three new vibrational peaks generate due to the presence of OMMT filler at 2948 cm−1, 2853 cm−1 and 1038 cm−1 for asymmetric and symmetric stretching vibration modes of methylene group (–CH2) of long aliphatic tail and Si–O–Si stretching.
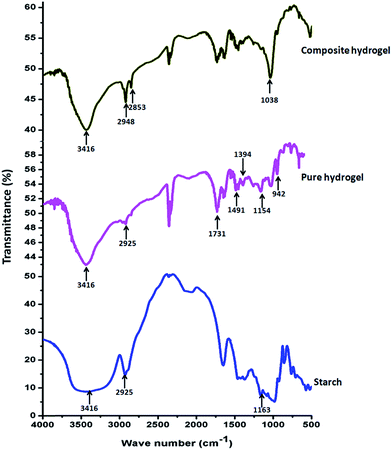 |
| Fig. 1 FTIR analysis results of pure starch, pure hydrogel and composite hydrogel. | |
3.3. SEM and EDAX analysis of hydrogel
The surface morphology of the pure hydrogel, composite hydrogel was shown in Fig. 2. From Fig. 2a it was observed that the pure hydrogel consists of a plain surface whereas upon addition of OMMT clay a change was observed in the surface morphology of the hydrogel (Fig. 2b). A rough surface morphology was evidenced after incorporation of OMMT (5 wt%). From FESEM image it was observed that at 5 wt% OMMT content distribution of filler was uniform throughout the hydrogel surface. But when the filler content was increased to 7.5 wt% (Fig. 2c), an agglomerated morphology appeared over the hydrogel surface.32 To further understanding of this phenomenon we carried out TEM analysis (Section 3.4.). Elemental mapping (Fig. 2d) and EDAX analysis (Fig. 2e) confirmed the presence of OMMT clay in the surface of the hydrogel.
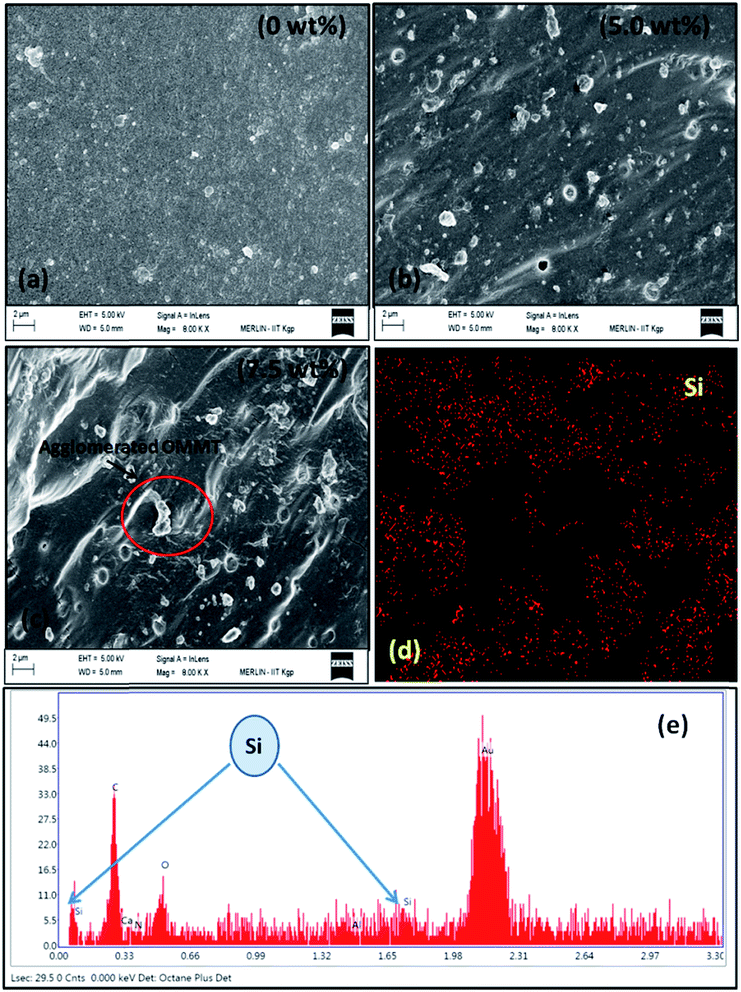 |
| Fig. 2 SEM analysis of (a) hydrogel without filler, (b) & (c) composite hydrogel with different content of OMMT, (d) bulk phase elemental mapping of the filler loaded hydrogel. (e) EDAX analysis of filler loaded hydrogel (5 wt% OMMT content). | |
3.4. TEM analysis
The TEM images of the composite hydrogel containing different amount of OMMT (2.5–7.5 wt%) were shown in Fig. 3. The thin and dark lines corresponded to the layer of the OMMT. The figure showed that at low concentration of clay (2.5%) (Fig. 3a) there was modest exfoliation of clay layer. At higher concentration (7.5 wt%) (Fig. 3c) the clay layer remained either partially exfoliated or at intercalated state. During the polymerization reaction the small monomer molecules present inside the clay layers undergo polymerization leading to high molecular weight polymers. If the elastic force created by the formed polymer molecules inside the clay layer was higher than external force given by the clay layer, then they could exfoliate the clay gallery leading to better distribution of the clay. This might occur in case of low filler loading (2.5 wt%). So from the image it was observed that, low amount of clay (2.5 wt%) loading provides uniform distribution of clay throughout the hydrogel, whereas higher filler loadings (7.5 wt%) result in poor dispersion of clay inside the hydrogel system.
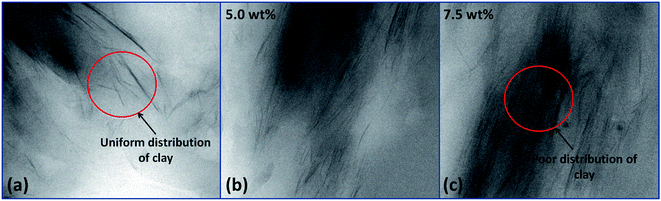 |
| Fig. 3 TEM images of hydrogel containing (a) 2.5% OMMT, (b) 5.0% OMMT and (c) 7.5% OMMT filler. | |
3.5. Swelling behavior study of hydrogel
3.5.1. Effect of crosslinker content. The effect of variation of crosslinker concentration (0.5–1.5%) at pH = 7.4 on the swelling nature of the hydrogel was shown in Fig. 4a. This indicates that with increase in crosslinker concentration swelling ratio decreased in a significant manner. This might be due to the formation of more crosslinking point at higher crosslinker ratio inside the hydrogel which reduced the free volume. This results a low diffusion of the solvent through the network.
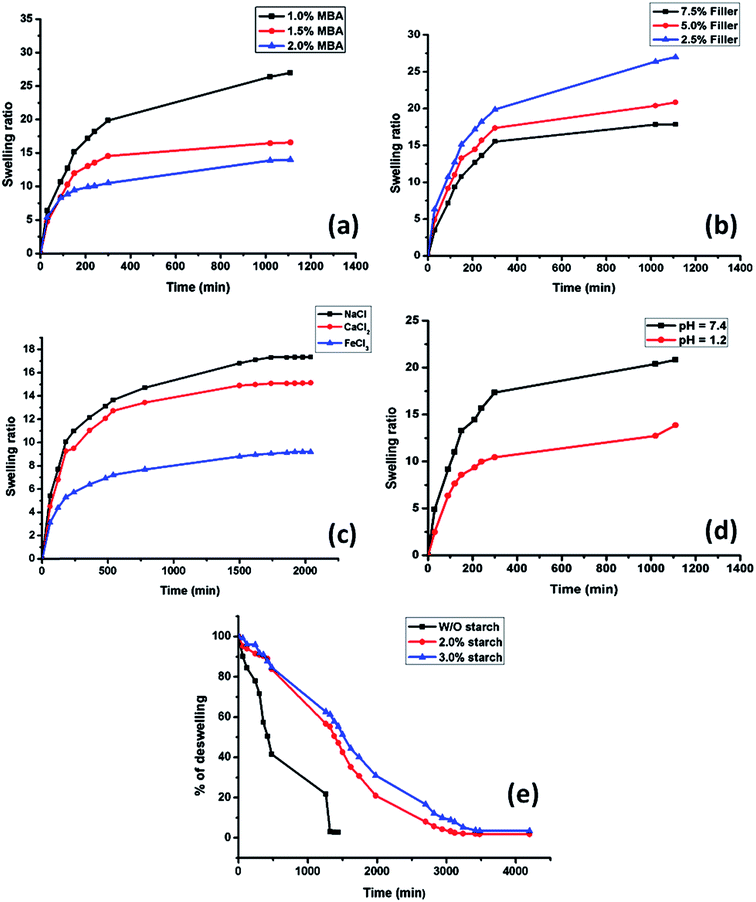 |
| Fig. 4 Swelling study of the prepared hydrogel with variation in (a) different amount of crosslinker, (b) in different amount of OMMT. (c) Swelling study of hydrogel in different salt solution. (d) Swelling study of the hydrogel containing 5 wt% OMMT at pH = 1.2 and pH = 7.4 solution. (e) Deswelling behavior of the hydrogel at varying content of starch. | |
3.5.2. Effect of clay content. The swelling behavior of the hydrogel containing different amount of OMMT at pH = 7.4 followed the similar trend as in the case of the crosslinker. The swelling ratio was decreased with increase in filler content. From Fig. 4b it was observed that when the clay content increased to 7.5 wt% from 2.5 wt% swelling ratio drastically reduced from 26 to 18. This might be due to the fact that, with increase in OMMT content, the nano clay particles acted as physical crosslinking points inside the gel along with the chemical crosslinking domains. This increase in crosslinking sites decreased the water absorbency of the gel. This type of observation was also reported by Fu et al.33 and Haraguchi et al.25
3.5.3. Effect of saline solution in swelling behavior of hydrogel. Fig. 4c showed the swelling behavior of the hydrogel in salt solution containing mono, bi and trivalent cations respectively. From the water absorption curve it was clear that with increase in valency of cation the swelling rate got decreased. In NaCl solution the hydrogel showed a swelling ratio of approximately 18, whereas in FeCl3 solution the swelling ratio decreased drastically to approximately 8. The unusual swelling behavior might be attributed due to following reasons. In multivalent salt solutions the –COO− groups of acrylic acid formed chelating complex with the bi and trivalent cations (Ca2+ and Fe3+). So the degree of crosslinking got increased with increase in contact time which resulted in a lower swelling compared to the swelling in monovalent (Na+) cationic solution which was not able to form chelate complex with –COO− ion.34
3.5.4. Effect of pH. The swelling behavior of ionic polymer containing hydrogel largely depends on the behavior of the hydrogel in different pH. In this hydrogel sample PAA exists in anionic form. The pKa value of acrylic acid is 4.26. Above the pKa value of acrylic acid, it became more hydrophilic in nature due to ionization of –COOH groups. In acidic pH all the carboxylic groups are protonated which leads to formation of hydrogen bonding between the –COOH groups. This increases the crosslink density of the hydrogel. Thus the diffusion of water gets reduced. But with increase in pH, the –COOH groups lost their protons and became negatively charged which generated repulsion between the polymeric chains. The maximum stretching of the polymeric chain with ionization depends on the percent ionization of the carboxylic groups.35 Fig. 4d showed that in acidic pH (pH = 1.2) the swelling of the hydrogel of composition S1 (Table 1) was lower than the swelling observed at pH = 7.4.
3.6. Deswelling behavior study of hydrogel
Fig. 4e indicates that the hydrogel sample without starch content showed rapid deswelling behavior but in presence of starch hydrogel showed a sustained deswelling behavior which supports the formation of semi IPN structure inside the hydrogel network.36 Due to the formation of semi IPN structure, long starch chain resists the water to diffuse out rapidly from bulk hydrogel which improved the water retention property of the hydrogel.
3.7. Study of pH responsive behavior of hydrogel
Fig. 5 showed the pH responsive behavior of the hydrogel. The hydrogel was repeatedly immersed in pH = 7.4 and pH = 1.2 respectively after a constant interval of time. From the figure it was noticed that the hydrogel showed a high swelling at pH = 7.4, whereas at pH = 1.2 it showed poor swelling. This switching on and off behavior depends on the external pH of the solution. This switch on-off behavior of the hydrogel was controlled by the presence of PAA acid in the main chain of copolymer. At acidic pH all the –COOH groups of acrylic acids were protonated and they formed intra-molecular hydrogen bonding between them which reduced their swelling. But in basic pH, most of the –COOH groups were converted to –COO−.37 These carboxylated anions repelled each other which accelerated swelling. From this study we can also check the gel strength at different pH medium. From the Fig. 5, it was observed that even after four oscillating on-off cycles, the hydrogel maintained its stability. It was also observed that with increase in the cationic monomer content, the reversible pH behavior tends to be minimized. It showed significant swelling in acidic medium also, which might be due to the existence of repulsive force between the quaternary ammonium cations which was predominant over the hydrogen bonding formed between –COOH groups.
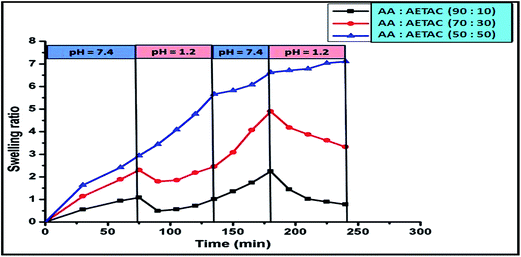 |
| Fig. 5 Study of pH responsive behavior of hydrogel with the variation of monomer composition. | |
3.8. Self-healing and shape retention study of the hydrogel
As described in Section 2.2.5., a cylindrical shaped composite hydrogel (containing 5 wt% of OMMT) of diameter of 10 mm and 40 mm length was sectioned into two parts by using sharp knife and after rejoining these two fragments (one part was dyed with rhodamine B) it was placed in the separate containers having solution of pH = 1.2, pH = 7.4 and NaCl solution of 0.154 (M) and 1 (M) at the temperature of 37 °C, which is the healing temperature of cuts in human skin. Fig. 6i indicates the joined section of the hydrogel that was healed after 12 h of standing in both acidic and physiological pH and in salt solutions. Generally chemically crosslinked hydrogels are difficult to self-heal,38 but in our work we succeeded to heal a chemically crosslinked hydrogel by taking the advantage of electrostatic attraction between the –N(CH3)3+ of the cationic monomer and –COO− of the anionic one at pH = 7.4 and also taking the advantage of hydrogen bonding at lower pH region. It was reported that most of the carboxylic groups of PAA are protonated at acidic pH region and they formed hydrogen bonding between themselves which help to generate a supramolecular bonding between the sectioned portions that helped in healing. At pH = 7.4, –COOH groups exist as –COO−, though the generation of –COO− was faster at higher pH. These –COO− groups made an interaction with the cationic –N(CH3)3+ and formed ionic bonding that took part in self-healing at physiological pH.39 We also observed the self-healing properties of the hydrogel in salt solution. It was observed that the hydrogel could heal at salt solution also and healing capacity increased with increase in salt concentration from 0.154 (M) to 1 (M). It might be due to the formation of –COO− in salt solution which caused an ionic interaction with –N(CH3)3+ ions. When this joined sample (healed at pH = 7.4) was elongated manually, it extended upto 100% without failure. This showed the strong physical bonding between the two cut surfaces of the hydrogel pieces, when they were joined at ambient temperature. It was also observed that composite hydrogel prepared without PAETAC (Fig. 6(i)e) and PAA (Fig. 6(i)f) didn't show any self-healing property at pH = 7.4. Fig. 6(ii) showed the elongation behavior of the uncut nanocomposite hydrogel which also showed a similar elongation behavior like the self-healed gel. From the Fig. 7 it was clearly observed that the cylindrical shape hydrogel could withstand high degree of various deformations like compression and bending. The interesting fact about this hydrogel system is that, it can retain its shape after high deformation that provides shape retention property to this hydrogel system. As described in Section 2.2.5., for shape retention study the composite hydrogel of 20 mm thickness was sandwiched between two parallel plates of a rheometer and then they were compressed to a certain thickness (6 mm). This situation was maintained for 10 minutes. After the withdrawal of the force, it was observed that hydrogel maintained its previous thickness. In another experiment the cylindrical hydrogel was bend upto 180° and then it was allowed to get back to its original state and it was observed that after 90 seconds it got back into its original shape without any visible change in shape. These two observations could be explained by the fact that due to the presence of high elasticity in the crosslinked gel which was generated by the effect of both chemical crosslinking (MBA crosslink) and physical crosslinking (ionic interaction and clay to polymer interaction), helped it to regain its original shape. The elastic nature of the composite hydrogel was again determined by using rheological study using parallel plate rheometer which was described in Section 3.9.
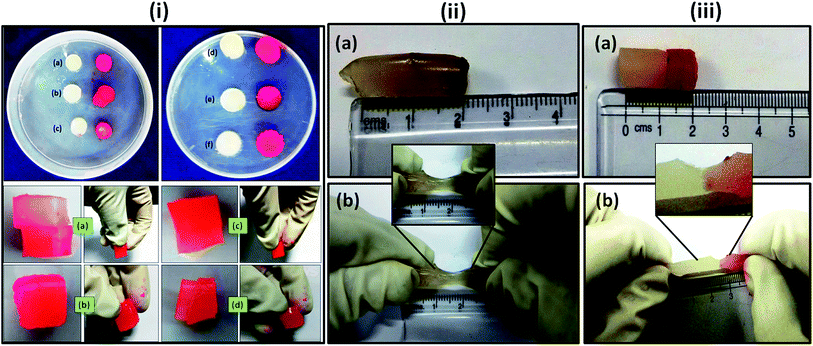 |
| Fig. 6 (i) Self-healing behavior study of prepared composite hydrogel containing 5 wt% OMMT at (a) 0.154 (M) NaCl solution, (b) 1 (M) NaCl solution, (c) pH = 1.2 and (d) pH = 7.4. (e) Composite hydrogel (5 wt% OMMT content) without PAETAC that did not heal at pH = 7.4 and (f) composite hydrogel (5 wt% OMMT content) without PAA that did not heal at pH = 7.4 (ii) elongation behavior of the uncut composite hydrogel ((a) before elongation and (b) after elongation). (iii) Elongation behavior of self-healed hydrogel ((a) before elongation and (b) after elongation) (during the experiment one part of the hydrogel was dyed with rhodamine B (red in color)). | |
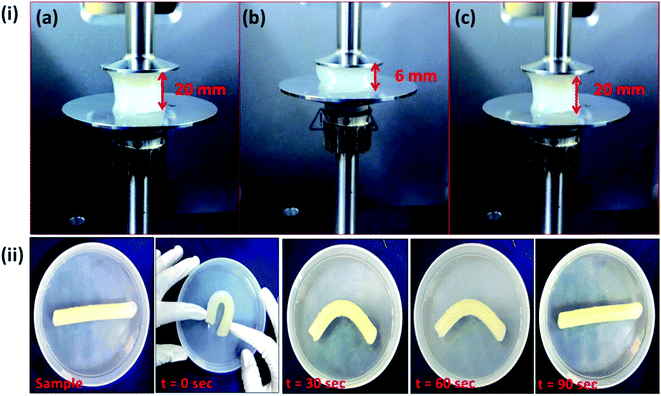 |
| Fig. 7 (i) Image of compression set study of the composite hydrogel containing 5 wt% of OMMT – (a) before compression, (b) during compression and (c) after compression. (ii) Bending test of the composite hydrogel. | |
3.9. Rheological behavior study
Rheological experiment was performed on the OMMT based composite hydrogel to quantify the rheological and viscoelastic properties of hydrogel by determining the mechanical feedback of the samples when they were subjected to deformation (compression). Fig. 8a and b depicted the isothermal response of the self-healed hydrogels as a function of frequency in terms of the storage (G′) and loss (G′′) modulus respectively. Fig. 8c showed the dynamic response (G′ and G′′) given by the pure hydrogel and composite hydrogel. From the first two figures it was observed that self-healed hydrogel showed similar storage and loss modulus compared to the pure uncut hydrogel and sometimes more G′ and G′′ values (in 1.2 pH and in 1 M NaCl solution) compared to pure hydrogel. G′ stands for the elastic component which defines the stiffness of the material, whereas viscous component is related to G′′ which is associated with the loss of energy as heat due to the internal molecular level friction. The increase in both elastic and loss modulus of the hydrogel in case of healing in acidic medium might be due to the formation of elastic hydrogen bond between the two cut surfaces, whereas salt healed hydrogel having a salt concentration of 1 M also showed higher storage as well as loss modulus than pure gel. This had been explained in Section 3.8. This was due to the formation of ionic interaction between quaternary ammonium ions and carboxylate anions. From the Fig. 8c it could be summarized that in the presence of filler elastic modulus increased significantly which indicated that the clay particle acted as an additional crosslinking agent that improved the elasticity of the hydrogel. Loss modulus also increased but not in a significant manner. The difference between the storage and loss modulus was significantly high which clearly indicated that the hydrogel had more elastic component than the viscous component.
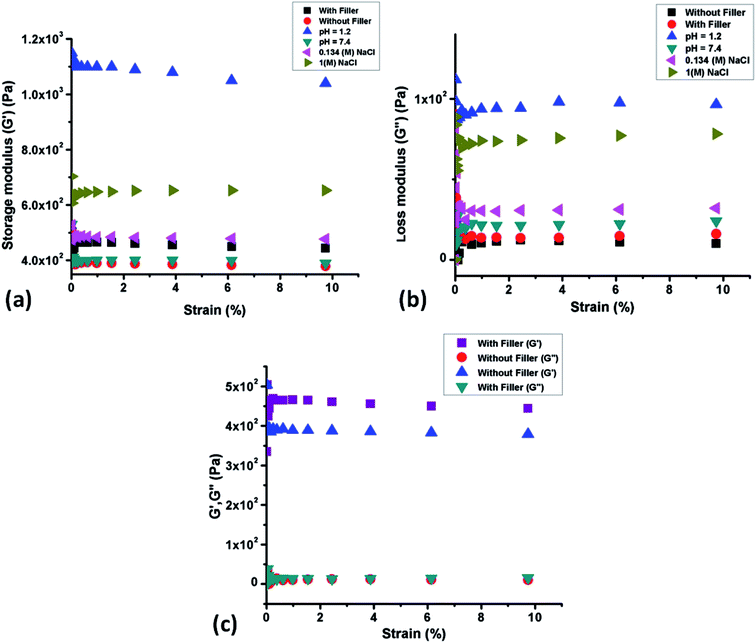 |
| Fig. 8 (a) Storage modulus (G′) change vs. strain in uncut hydrogel and self-healed hydrogel, (b) loss modulus (G′′) change vs. strain in uncut hydrogel and self-healed hydrogel and (c) plot of G′ & G′′ vs. strain for pure hydrogel and composite hydrogel containing 5.0 wt% filler. | |
4. Conclusion
In summary, a three component nanocomposite hydrogel was prepared by copolymerization of acrylic acid and 2-(acryloyloxy)ethyl trimethylammonium chloride in presence of soluble starch. The as-prepared hydrogel was characterized with FTIR, FESEM and TEM analyses which demonstrated the successful synthesis of composite hydrogel. This hydrogel showed high swelling behavior and very good self-healing nature in a wide range of pH (from 1.2 to 7.4) that simulated the human body pH. The synthesized hydrogel had very good pH responsive behavior which was increased with increase in acrylic acid content. Rheological study showed that the self-healed hydrogel exhibited comparable strength with the uncut gel. From storage modulus (G′) results, it was also observed that the prepared hydrogel had more elastic component than the viscous one. The elastic behavior increased with OMMT incorporation due to formation of physical crosslinking sites. Self-healed hydrogel showed very good elongation property (100%) without break. From compression and bending study it was observed that the prepared hydrogel had very good shape retention property which might be due to the presence of high elastic components inside the hydrogel. The above observations indicate that the synthesized ionic nanocomposite hydrogel can be used as a potential material for biomedical applications like in preparation of smart dressing materials, smart pH responsive self-healable gel coating and in biomedical devices.
Acknowledgements
SLB is thankful to Indian Institute of Technology Kharagpur for providing Institute Fellowship.
References
- A. Lendlein and R. Langer, Science, 2002, 296, 1673–1676 CrossRef PubMed.
- T. Miyata, N. Asami and T. Uragami, Nature, 1999, 399, 766–769 CrossRef CAS PubMed.
- M. Meyer, A. Philipp, R. Oskuee, C. Schmidt and E. Wagner, J. Am. Chem. Soc., 2008, 130, 3272–3273 CrossRef CAS PubMed.
- F. Peng, G. Li, X. Liu, S. Wu and Z. Tong, J. Am. Chem. Soc., 2008, 130, 16166–16167 CrossRef CAS PubMed.
- J. J. Lai, J. M. Hoffman, M. Ebara, A. S. Hoffman, C. Estournès, A. Wattiaux and P. S. Stayton, Langmuir, 2007, 23, 7385–7391 CrossRef CAS PubMed.
- T.-A. Asoh and A. Kikuchi, Chem. Commun., 2012, 48, 10019–10021 RSC.
- C. Cheng, A. J. Convertine, P. S. Stayton and J. D. Bryers, Biomaterials, 2012, 33, 6868–6876 CrossRef CAS PubMed.
- P. Techawanitchai, M. Ebara, N. Idota, T.-A. Asoh, A. Kikuchi and T. Aoyagi, Soft Matter, 2012, 8, 2844–2851 RSC.
- B. Jeong and A. Gutowska, Trends Biotechnol., 2002, 20, 305–311 CrossRef CAS PubMed.
- H. Liu and S. Wang, Sci. China: Chem., 2014, 57, 552–557 CrossRef CAS.
- B. Xue, L. Gao, Y. Hou, Z. Liu and L. Jiang, Adv. Mater., 2013, 25, 273–277 CrossRef CAS PubMed.
- Y. Kotsuchibashi, M. Ebara, K. Yamamoto and T. Aoyagi, Polym. Chem., 2011, 2, 1362–1367 RSC.
- J. A. Syrett, C. R. Becer and D. M. Haddleton, Polym. Chem., 2010, 1, 978–987 RSC.
- S. R. White, N. Sottos, P. Geubelle, J. Moore, M. R. Kessler, S. Sriram, E. Brown and S. Viswanathan, Nature, 2001, 409, 794–797 CrossRef CAS PubMed.
- Z. Wei, J. H. Yang, J. Zhou, F. Xu, M. Zrínyi, P. H. Dussault, Y. Osada and Y. M. Chen, Chem. Soc. Rev., 2014, 43, 8114–8131 RSC.
- G. Akay, A. Hassan-Raeisi, D. C. Tuncaboylu, N. Orakdogen, S. Abdurrahmanoglu, W. Oppermann and O. Okay, Soft Matter, 2013, 9, 2254–2261 RSC.
- J. Cui and A. del Campo, Chem. Commun., 2012, 48, 9302–9304 RSC.
- T. Kakuta, Y. Takashima, M. Nakahata, M. Otsubo, H. Yamaguchi and A. Harada, Adv. Mater., 2013, 25, 2849–2853 CrossRef CAS PubMed.
- J. Yang, L.-H. Deng, C.-R. Han, J.-F. Duan, M.-G. Ma, X.-M. Zhang, F. Xu and R.-C. Sun, Soft Matter, 2013, 9, 1220–1230 RSC.
- Z. Shafiq, J. Cui, L. Pastor-Pérez, V. San Miguel, R. A. Gropeanu, C. Serrano and A. del Campo, Angew. Chem., Int. Ed., 2012, 124, 4408–4411 CrossRef.
- C. Li and G. Shi, Adv. Mater., 2014, 26, 3992–4012 CrossRef CAS PubMed.
- E. Zhang, T. Wang, L. Zhao, W. Sun, X. Liu and Z. Tong, ACS Appl. Mater. Interfaces, 2014, 6, 22855–22861 CAS.
- T. Wang, J. Huang, Y. Yang, E. Zhang, W. Sun and Z. Tong, ACS Appl. Mater. Interfaces, 2015, 7, 23423–23430 CAS.
- K. Haraguchi and T. Takehisa, Adv. Mater., 2002, 14, 1120 CrossRef CAS.
- K. Haraguchi, K. Uyama and H. Tanimoto, Macromol. Rapid Commun., 2011, 32, 1253–1258 CrossRef CAS PubMed.
- H.-P. Cong, P. Wang and S.-H. Yu, Chem. Mater., 2013, 25, 3357–3362 CrossRef CAS.
- R. Das, R. Kumar, S. L. Banerjee and P. Kundu, RSC Adv., 2014, 4, 59265–59274 RSC.
- L. Wang and A. Wang, J. Hazard. Mater., 2008, 160, 173–180 CrossRef CAS PubMed.
- R. Kizil, J. Irudayaraj and K. Seetharaman, J. Agric. Food Chem., 2002, 50, 3912–3918 CrossRef CAS PubMed.
- J. Billingham, C. Breen and J. Yarwood, Vib. Spectrosc., 1997, 14, 19–34 CrossRef CAS.
- Y. Huang, J. Lu and C. Xiao, Polym. Degrad. Stab., 2007, 92, 1072–1081 CrossRef CAS.
- J.-W. Rhim, Carbohydr. Polym., 2011, 86, 691–699 CrossRef CAS.
- G. Gao, G. Du, Y. Sun and J. Fu, ACS Appl. Mater. Interfaces, 2015, 7, 5029–5037 CAS.
- G. Mahdavinia, A. Pourjavadi, H. Hosseinzadeh and M. Zohuriaan, Eur. Polym. J., 2004, 40, 1399–1407 CrossRef CAS.
- L. Shi, L. Yang, J. Chen, Y. Pei, M. Chen, B. Hui and J. Li, J. Biomater. Sci., Polym. Ed., 2004, 15, 465–474 CrossRef CAS PubMed.
- M. Boruah, P. Gogoi, A. K. Manhar, M. Khannam, M. Mandal and S. K. Dolui, RSC Adv., 2014, 4, 43865–43873 RSC.
- S. Jin, M. Liu, F. Zhang, S. Chen and A. Niu, Polymer, 2006, 47, 1526–1532 CrossRef CAS.
- D. C. Tuncaboylu, A. Argun, M. P. Algi and O. Okay, Polymer, 2013, 54, 6381–6388 CrossRef CAS.
- Y. Huang, H. Yu and C. Xiao, Carbohydr. Polym., 2007, 69, 774–783 CrossRef CAS.
Footnote |
† Electronic supplementary information (ESI) available. See DOI: 10.1039/c6ra01074a |
|
This journal is © The Royal Society of Chemistry 2016 |