DOI:
10.1039/C5RA19529B
(Paper)
RSC Adv., 2016,
6, 7914-7922
Fabrication and characterization of a collagen coated electrospun poly(3-hydroxybutyric acid)–gelatin nanofibrous scaffold as a soft bio-mimetic material for skin tissue engineering applications
Received
22nd September 2015
, Accepted 28th December 2015
First published on 6th January 2016
Abstract
Wound healing is a global health care problem. The use of a suitable dressing material by means of a nanofibrous scaffold with traditionally important medicine can help to repair the damaged skin tissue. An ideal wound dressing material should mimic the function of an extracellular matrix with its improved physiochemical, biological and antimicrobial properties. In this study, the significance features of a collagen coated electrospun poly(3-hydroxybutyric acid)–gelatin nanofibrous scaffold with a bioactive Coccinia grandis extract (CPE) meets the requirements for a wound dressing material. The nanofibrous scaffold with collagen has an attraction for fibroblast, which increases cell adhesion and proliferation. The fabricated nanofibrous scaffold with collagen was characterized physio-chemically using Fourier transform infrared (FTIR) spectroscopy, scanning electron microscopy (SEM), and it showed acceptable antibacterial property with both Gram positive and Gram negative bacteria. The thermal and in vitro stability of the nanofibrous scaffold was studied and it was found to have stability more than that required for a wound dressing material. The nanofibrous scaffold supports good swelling property with better porosity for oxygen permeability. The mechanical property of the nanofibrous scaffold showed a Young's modulus of 2.99 ± 0.16 MPa. The biocompatibility of the nanofibrous scaffold exhibits increased cell adhesion and proliferation of both NIH 3T3 fibroblast and human keratinocytes (HaCaT) cell line. The in vitro fluorescence staining of the nanofibrous matrix using Calcein AM and DAPI exhibits the cell material interaction of the collagen coated nanofibrous scaffold corresponding to increased cell adhesion and proliferation. This approach with a nanofibrous scaffold coated with collagen can be a promising tool in skin tissue engineering and can be useful as a wound dressing material in skin tissue engineering applications.
Introduction
The advancement in tissue engineering provides a combination of multidisciplinary fields for the development of a matrix scaffold enriched with therapeutic products for the repair, restoration and regeneration of the damaged cells or tissues.1 The use of nanofibrous scaffolds in tissue engineering applications involves the support of the cells by mimicking the function of the extracellular matrix (ECM).2 The nanofibrous scaffold has excellent structural similarity to the ECM with its ability to provide a substratum for cell migration into the defective sites.3 The efficiency of the nanofibrous scaffold in wound healing has several advantages in retaining certain properties to allow good oxygen permeability, flexibility, high surface to volume ratio and biocompatibility with sequential reformation in the wound healing process.4 The triple helical structure with a high glycine amino acid content of collagen is most important for the attachment and proliferation of cells throughout the collagen matrix, which mimics the function of the native cells.5,6 The collagen coated nanofibrous scaffold exhibits significant function over the extracellular matrix (ECM) because of its matrix scaffolding and resistance to allow cell–cell interaction,7 cell–ECM interaction8 and high mechanical property.9,10 The electrospun biomaterial enhances the ability of the nanofibre due to the high surface to volume ratio with its porous structure, which favors both the physical and biological properties of the nanofibrous scaffold.11
Poly(3-hydroxybutyric acid) (PHB) is a hydrophobic polymer with excellent applications in the field of medicine because of its biodegradability and biocompatibility.12 The combination of PHB has been used for various products such as sutures, stents, nerve guides and wound dressing materials.13 PHB is a common metabolite in all higher living organisms, which provides proof of its non-toxicity.14 The electrospinning of collagen causes denaturation and results in the formation of gelatin, a major constituent of the ECM.15 The gelatin with its excellent biocompatibility, non-antigenicity, and adhesiveness properties has wide applications in biomedical and pharmaceutical fields.16
The traditionally important compound from the plant possesses a bioactive constituent that acts as a conventional medicine. Ancient villagers used numerous crude extracts for the healing of skin diseases and wounds. The modern world sometimes relies on the indigenous traditional medicine for their primary health needs.17 In Coccinia grandis, commonly known as ivy gourd, all the plant parts are traditionally important for skin related problems. The bioactive Coccinia grandis leaf extract (CPE) exhibits enhanced antioxidant properties to treat ulcers, diabetes, and antibacterial activity.18
The biomaterial with ideal properties cannot be attained alone with natural and synthetic polymers. The individual polymers have their drawbacks associated with improved physical, mechanical and biological properties. To overcome these drawbacks with the individual polymer, the electrospinning of a nanofibrous scaffold by blending two polymers with a surface coating of collagen has been explored to assimilate all the properties in the design of a nanofibrous scaffold for tissue engineering applications.19
In this context, some researchers have blended gelatin with various synthetic polymers such as poly(ε-caprolactone) (PCL),20–22 polyvinyl alcohol (PVA),23,24 poly(L-lactide) (PLLA),25 polyglycolic acid (PGA)26 and poly(3-hydroxybutyrate-co-3-hydroxyvalerate) (PHBV)27 for various tissue engineering applications. However, the use of poly(3-hydroxybutyric acid) (PHB) blended with gelatin and a uniform coating of collagen over the nanofibrous scaffold has been investigated thoroughly for biocompatibility, cell adhesion, cell proliferation, cell attachment and spatial distribution of the cells throughout the nanofibrous matrix with NIH 3T3 fibroblasts and human keratinocytes (HaCaT) cells.
The current study was driven towards the fabrication, characterization and application of collagen coated bioactive nanofibrous scaffold for tissue engineering. Poly(3-hydroxybutyric acid) (P) and gelatin (G) were electrospun to form a nanofibrous scaffold with the incorporation of a bioactive extract (CBE) to provide antibacterial properties. Furthermore, the developed PG-CPE nanofibrous scaffold was coated with the Arothron stellatus collagen (COL) solution to obtain a PG-CPE-COL scaffold as a wound dressing material for tissue engineering applications.
Materials and methods
Materials
The Arothron stellatus fish was collected from the Bay of Bengal in Nagapattinam, Tamil Nadu, India. The leaves of Coccinia grandis (Cucurbitaceae) were collected from Tiruvarur, Tamil Nadu, India, and authenticated with the help of a plant taxonomist Dr N. Veerappan, Associate Professor, Sir Theayagaraya College, Chennai, Tamil Nadu, India. Poly(3-hydroxybutyric acid), gelatin, 1,1,1,3,3,3-hexafluoro-2-propanol, 3-(4,5-dimethylthiazol-2-yl)-2,5-diphenyl tetrazolium bromide (MTT), 4,6-diamino-2-phenylindol (DAPI), Calcein AM, Dulbecco's modified Eagle's medium (DMEM), fetal calf serum (FCS), and supplementary antibiotics for tissue culture were purchased from Sigma Aldrich, India. The NIH 3T3 fibroblast and human keratinocytes (HaCaT) cell lines were obtained from the National Centre for Cell Science (NCCS), Pune, India. Other chemicals and culture wares were purchased from Sigma Aldrich, unless specified otherwise.
Methods
Extraction of collagen from Arothron stellatus skin. The fish skin obtained was washed, chopped and the fat was removed, and the skin then soaked in 0.5 M acetic acid for 3 days. The loosened thin epidermal layer was removed from the skin and the swollen dermal skin pieces were homogenized with 0.5 M acetic acid using a homogenizer (Ultra Turax T-50, IKA Werke, Germany). The homogenized skin was centrifuged at 8000 rpm for 30 min at 4 °C. The precipitated salt was dialyzed against a 0.1 M acetic acid solution to obtain Arothron stellatus skin collagen. The collagen was washed with distilled water, and then lyophilized (Operon Co., Korea).28
Extraction of Coccinia grandis plant extracts (CPE). Healthy and fresh leaves of Coccinia grandis were collected and their surface was cleaned with Millipore-filtered water, shade dried, and finely powdered with the help of an electric blender. An ethanol extract was prepared by subjecting 10 g of the finely powdered leaf in a Soxhlet apparatus. The aqueous extract was concentrated using a rotary evaporator apparatus under reduced pressure and the CPE was stored at 4 °C.29
Electrospinning nanofibrous scaffold containing plant extract coated with collagen (PG-CPE-COL). To prepare the PG-CPE nanofibre scaffold, a 4 wt% concentration of polymer solution was prepared by dissolving 0.4 g of poly(3-hydroxybutyric acid) and gelatin in 10 mL 1,1,1,3,3,3-hexafluoro-2-propanaol. The dissolved solution obtained after constant stirring for 12 hours was mixed to obtain a blended solution of poly(3-hydroxybutyric acid) and gelatin at a ratio of 1
:
1 with 0.5 mg mL−1 of CPE, which was electrospun with 24 G needle connected to the positive terminal of the high-voltage DC power supply (ZEONICS, Bangalore, India). The polymer solution was extruded at 1.5 mL h−1 using a computer-controlled syringe pump and was subjected to an electric potential of 1.5 kV cm−1. The fibers were collected onto a grounded aluminum substrate around a rotary drum placed at a distance of 12 cm perpendicular to the needle. The 4 wt% collagen solution in 0.1 M acetic acid was prepared. Further, the electrospun PG-CPE scaffold was coated with the prepared collagen solution and dried at room temperature (37 °C) to obtain the PG-CPE-COL scaffold. The prepared scaffolds were stored at room temperature until further use.14
Characterization
Fourier transform infrared spectroscopy (FTIR). Fourier transform infrared (FTIR) measurements were carried out to determine the functional groups present in the prepared PG, PG-CPE and PG-CPE-COL nanofibrous scaffolds. The spectra were obtained at a resolution of 4 cm−1 over the frequency range of 4000–600 cm−1 using an ABB 3000 spectrometer with Grams as the operating software.14 Thermogravimetric analysis (TGA) and differential scanning calorimetry (DSC) of the nanofibrous scaffolds were performed using universal V4.4A TA instruments.30
Scanning electron microscopy (SEM). The scaffolds were mounted on brass studs and gold coated using an ion coater (Emitech brand). The surface morphology of the scaffolds was visualized using F E I Quanta FEG 200-HRSEM operating at an accelerating voltage of 5–20 kV. Fifty different fibers were measured using the UTHSCA Image tool software to determine the average diameter of the fibers for different concentrations of the polymer solution.30
Porosity
The porosity of the nanofibrous scaffold was determined via a liquid displacement method using ethanol as the displacement liquid because of its easy penetration through the pores of the scaffolds, which will not induce shrinking or swelling as a non-solvent of the polymers.31 A known weight (W) of the sample was immersed in a graduated cylinder containing a known volume (V1) of ethanol. The sample was kept in ethanol for 5 min, and a series of brief evacuation–repressurization cycles were then conducted to force the ethanol into the pores of the scaffold. The process was repeated until the air bubbles stopped forming. The total volume of the ethanol and the ethanol-impregnated scaffolds were then recorded as V2. The difference in the volume was calculated as V2 − V1. The scaffolds impregnated in ethanol were removed from the cylinder, and the residual ethanol volume was recorded as V3. The porosity of the dressing was obtained using the following equation: |
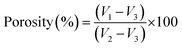 | (1) |
In vitro enzymatic degradation
Nanofibrous scaffolds were exposed to collagenase enzyme (Sigma #C0773) to assess the biological stability of the material and its degradation rate. A known weight of each sample in triplicate was taken and they were air dried overnight at room temperature. All the samples were exposed to the collagenase enzyme (100 units per mL) for 24 h at 37 °C (pH 7.4). After 24 h, all the test samples were removed from the incubator, dried by blotting and left to air dry for 24 h at room temperature. The percentage weight loss and final mass were calculated as a simple ratio. The enzyme solutions were prepared in a phosphate buffer solution (PBS, pH = 7.4). The extent of biomaterial degradation was determined gravimetrically through the weight loss.32
Swelling behavior
The in vitro swelling behavior of the nanofibrous scaffold were investigated by cutting the scaffold into a square piece (10 × 10 mm2) and immersing it in PBS (pH 7.4) at room temperature until the film reached swelling saturation. The weights of the scaffolds were determined after removing the surface wetness using a filter paper. The equilibrium-swelling ratio was calculated using the following equation. |
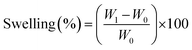 | (2) |
where W0 and W1 are the initial and the final weights of the film, respectively.33
Tensile strength measurement
All the scaffolds were cut into dumb-bell shaped specimens (100 × 16 mm2), and the load–elongation measurement was measured using a universal testing machine (INSTRON model 1405) according to Vogel at an extension rate of 5 mm min−1.30
In vitro biocompatibility, cell adhesion and proliferation studies
The cell viability of the nanofibrous scaffolds was determined using a MTT assay. The NIH 3T3 fibroblast and human keratinocytes (HaCaT) cell lines were grown on the PG-CPE-COL nanofibrous scaffold placed in 24-well plates (Corning, NY) and maintained in DMEM with 10% fetal calf serum supplemented with penicillin (120 units per mL), streptomycin (75 mg mL−1), gentamycin (160 mg mL−1), and amphotericin B (3 mg mL−1) at 37 °C at a density of 5 × 104 cells per mL and then incubated over a time interval for 24 h, 72 h and 7 days in a humidified atmosphere containing 5% CO2. Cells cultured in blank wells were used as the control.11 After 24 h, 72 h and 7 days the culture medium was replaced with a serum-free medium containing 10 μL of 3-(4,5-dimethylthiazol-2-yl)-2,5-diphenyl tetrazolium bromide (MTT) and incubated at 37 °C for 4 h in a humidified atmosphere containing 5% CO2. The medium was aspirated and 500 μL per well of dimethylsulfoxide (DMSO) was then added to dissolve the formazan needles with slow agitation for 10 min to yield a bluish purple solution. The absorbance of the dissolved solution was measured at 570 nm using Universal Microplate Reader.33
The cell attachment and proliferation of both NIH 3T3 fibroblast and human keratinocytes (HaCaT) cell lines were quantified for the live and nuclei staining at regular time intervals (6, 12, 24 and 48 hours); furthermore, the medium was removed and the cells were fixed with 4% paraformaldehyde and washed several times with PBS. In addition, each cell was first stained with DAPI to visualize the cell nuclei. To achieve this aim, the cells were treated with a DAPI solution (1.0 mg mL−1) for 15 min at 37 °C and washed again with PBS several times. A Calcein AM solution (2 μM; 400 μl) was added and incubated for 30 min at 37 °C. The plates with the scaffold were washed several times with PBS and viewed under a fluorescence microscope (EVOS FLoid Cell Imaging Station, Thermo fisher SCIENTIFIC, USA).34,35
Bacterial culture and antimicrobial activity
In this study, two bacterial strains were used, including one Gram positive bacteria Staphylococcus aureus (ATCC 11632) and one Gram negative bacteria Escherichia coli (ATCC 10536). All bacterial cultures were subcultured and maintained aseptically. The antimicrobial activity of the scaffolds was evaluated using the modified agar well diffusion method. About 100 μL [105 CFU (colony forming units)] of each bacterial culture was spread over the agar surface (Muller-Hinton agars) using a sterile glass spreader. The plates were then incubated for 24 h at 37 °C. The antibacterial activity was evaluated by measuring the zone of inhibition against the test organism.35
Statistical analysis
All the experiments were conducted in triplicate. The results are presented as the mean ± S.D. (n = 3). ANOVA (analysis of variance) and a student's t-test were carried out to determine the significant differences among the groups. The observed differences were statistically significant when p < 0.05.
Results and discussion
Characterization
Fourier transform infrared spectroscopy (FTIR). The structural modification due to the blending of PG with CPE in electrospinning was confirmed by FTIR spectroscopy, as depicted in Fig. 1(a). No apparent peaks of the solvent used during the electrospinning process were observed. All the peaks found in the IR spectrum were corresponding to PG, PG-CPE and PG-CPE-COL. The entire scaffold showed characteristic amide I (1600–1660 cm−1), amide II (∼1550 cm−1) and amide III (1320–1220 cm−1) absorption bands of gelatin.36 The characteristic absorption band of PHB was seen in the range of 1720–1740 cm−1 and 1270–1280 cm−1, which corresponds mainly to the ester carbonyl group and –CH group.37 The PG-CPE-COL scaffold showed the characteristic absorption band of amide A (3100–3600 cm−1) and exhibited a broad band associated with the N–H stretching vibration of hydrogen-bonded amide groups. Amide I (1600–1660 cm−1) corresponds to the carbonyl group, which is present within the triple helix structure in the secondary structure of the protein. The N–H bending and N–H stretching vibration were assigned to amide II (∼1550 cm−1) and amide III (1320–1220 cm−1), respectively.33 The presence of a phenolic deformation and carbonyl group at 1414 cm−1 and 1740–1630 cm−1 corresponds to the alkaloids present in the both CPE blended nanofibrous scaffold.38 All scaffolds exhibited C–H stretching absorption bands at 2987 cm−1 and 2933 cm−1, corresponding to the CH2 and CH3 in the PHB and gelatin scaffolds.14 The IR spectral data exhibit all the characteristic peaks corresponding to the entire nanofibrous scaffolds.
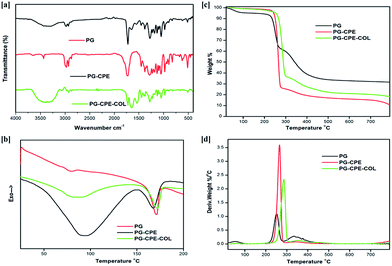 |
| Fig. 1 (a) FTIR spectra, (b) DSC, (c) TGA and (d) DTA of nanofibrous scaffold. | |
Thermal analysis. Thermogravimetric analysis (TGA) and differential thermal analysis (DTA) data of the nanofibrous scaffolds are shown in Fig. 1(c) and (d). The scaffold can be utilized for tissue engineering and biomedical application because it reveals satisfactory thermal stability. A single-step weight loss was observed between 250 and 300 °C, which was apparent from the Tmax and T−5% values presented in Table 1. The characteristic change in the endothermic peaks of the nanofibrous scaffold is presented in Fig. 1(b). DSC reveals the denaturation temperature of collagen in the PG-CPE-COL nanofibrous scaffold at 81.5 °C. The PG, PG-CPE and PG-CPE-COL scaffolds showed endothermic peaks at 173.13 °C, 167.97 °C and 171.95 °C, respectively, due to the degradation of the scaffold. The collagen-coated PG-CPE scaffold showed a significant interaction with both CPE and PG with increasing denaturation temperature.33,39
Table 1 Thermal properties of the electrospun nanofibrous scaffolds
Sample name |
T−5% (°C) |
Tmax1 (°C) |
Tmax2 (°C) |
Td (°C) |
Tm (°C) |
ΔHm (J g−1) |
ΔHd (J g−1) |
PG |
197.3 |
265.6 |
350.6 |
77.7 |
173.13 |
161.53 |
−1.47 |
PG-CPE |
198.1 |
252.8 |
334.7 |
93.30 |
167.97 |
−11.53 |
−116.3 |
PG-CPE-COL |
200.2 |
287.1 |
353.2 |
81.5 |
171.95 |
−30.98 |
−89.33 |
Scanning electron microscopy (SEM). Fig. 2(a–c) and (d–f) depict the SEM image and digital images of the nanofibrous scaffold PG, PG-CPE and PG-CPE-COL. The mean diameters of the fibers (Fig. 2(g–i)) were approximately 240 ± 30 nm. Incomplete evaporation of the solvent was observed in the collagen-coated PG-CPE scaffold. Fig. 2 shows that collagen is comprised of highly interconnected small micro fibrils onto the fibers of the PG-CPE scaffold as a collagen matrix embedded nanofibrous scaffold. The coalescence of the nanofibrous scaffolds clearly showed visible fibers at the junctions, making a greater contribution to the enhancement of the tensile strength of the nanofibrous scaffold. Overall, the fibrous nature of the scaffold will improve the cell attachment and permeability of oxygen.14,40
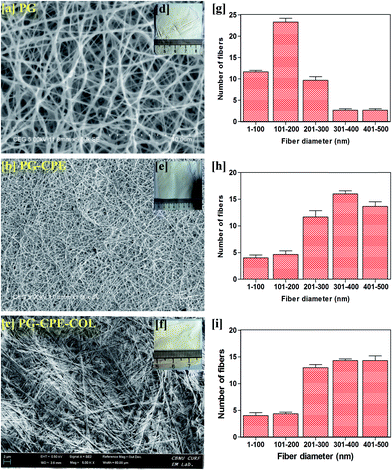 |
| Fig. 2 (a–c) SEM micrographs of the nanofibrous scaffold with their fiber diameters (g–i) and (d–f) insight showing the digital images of the nanofibrous scaffold. | |
Porosity
The PG and PG-CPE nanofibrous scaffold showed about 72% porosity with a decreasing rate compared to the collagen-coated scaffold (Fig. 3). This was due to the strong adherence of the collagen matrix between the nanofiber matrix.
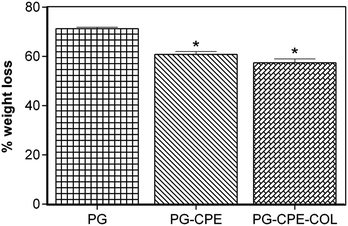 |
| Fig. 3 Porosity measurements of the nanofibrous scaffold (*p < 0.05; data presented are mean ± SD, n = 3). | |
Therefore, PG-CPE-COL exhibited only 64.3% porosity. The material used for the wound dressing should accomplish the porous nature for oxygen and nutrient exchange. In soft tissue engineering, scaffold materials should have a porous nature for nutrient and gas exchange.31,32
In vitro enzymatic degradation
The in vitro enzymatic stability of the nanofibrous scaffold was assessed to measure the degradation rate and biological stability against the collagenase enzyme. Fig. 4 shows that the PG and PG-CPE nanofibrous scaffold exhibited 71.29% and 60.8% weight loss, respectively, whereas the PG-CPE-COL nanofibrous scaffold showed 57.4% weight loss. The existence of a collagen matrix causes an increased reduction in the biodegradability of the PG-CPE-COL nanofibrous scaffold. Thus, the presence of collagen decreases the biostability of the scaffold, but this property will aid in cell proliferation and attachment when it will act as a dressing material.41
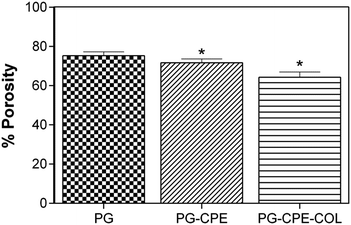 |
| Fig. 4 In vitro enzymatic degradation of the nanofibrous scaffold (*p < 0.05; data presented are mean ± SD, n = 3). | |
Swelling behavior
It is important to fabricate a nanofibrous scaffold with good swelling behavior to absorb the wound exudates and to keep the wound region dry and free from infection. The swelling behavior of the PG-CPE-COL nanofibrous matrix (Fig. 5) showed better swelling behavior, which reached saturation after 24 h. Only the collagen-coated nanofibrous scaffold showed an increase in swelling due to the surface binding of the collagen to the nanofibrous matrix. However, the CPE binds with gelatin during the electrospinning process and forms a PG-CPE nanofibrous scaffold with increased swelling index compared to the PG scaffold. Fig. 5 presents the swelling behavior of the nanofibrous scaffold. The initial swelling of the collagen increases the porosity of the nanofibrous scaffold and enhances the oxygen permeability with increasing porosity of the scaffold, thereby improving the wound healing efficiency.33,42
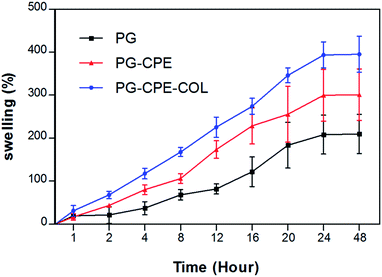 |
| Fig. 5 Swelling behavior of the nanofibrous scaffold. | |
Tensile strength measurement
The nanofibrous scaffold needs to be robust enough with prominent mechanical property for both handling and sterilization, before it has to be applied to the wound surface. The strength of the fibrous scaffold was mainly due to the property of the material used in the fabrication. The mechanical property based on the uniaxial tensile strength was conducted and the results are listed in Table 2. The PG and PG-CPE scaffold exhibited relatively similar Young's modulus of 2.84 ± 0.07 MPa and 2.83 ± 0.32 MPa, respectively. The collagen coated scaffold showed significantly high tensile strength with a Young's modulus of 2.99 ± 0.16 MPa. The increase in Young's modulus of the PG-CPE-COL was due to the enhanced entanglement of the collagen over the PG-CPE scaffold used as the tissue engineering nanofibrous scaffold.43
Table 2 Tensile properties of the nanofibrous scaffoldsa
Sample name |
Mean tensile strength (MPa) |
Mean elongation at break (%) |
Mean extension at the maximum load (mm) |
Mean Young's modulus (MPa) |
(*p < 0.05; data presented are the mean ± SD, n = 3). |
PG |
13.7 ± 0.7 |
15.8 ± 0.6 |
4.8 ± 0.2 |
2.84 ± 0.07* |
PG-CPE |
13.1 ± 0.6 |
15.3 ± 0.5 |
4.6 ± 0.3 |
2.83 ± 0.32* |
PG-CPE-COL |
14.8 ± 0.4 |
16.9 ± 0.5 |
4.9 ± 0.3 |
2.99 ± 0.16* |
In vitro biocompatibility, cell adhesion and proliferation studies
The tissue engineering applications of the nanofibrous scaffolds with better biocompatibility was determined using a MTT assay. The percentage cell viability of the scaffolds with NIH 3T3 fibroblasts and human keratinocytes (HaCaT) cells after 1, 3 and 7 days is shown in Fig. 6 and 7. The PG-CPE-COL showed a significant increase in the cell viability of the scaffold. The presence of collagen in the PG-CPE scaffold enhanced the biocompatibility of the nanofibrous scaffold.43,44
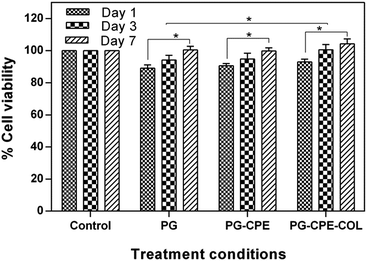 |
| Fig. 6 In vitro biocompatibility of the NIH 3T3 fibroblast cell line over 1, 3 and 7 days using a MTT assay scaffold after 7 and 14 days. The data are represented as the mean ± standard deviation; n = 3 (*p < 0.05). | |
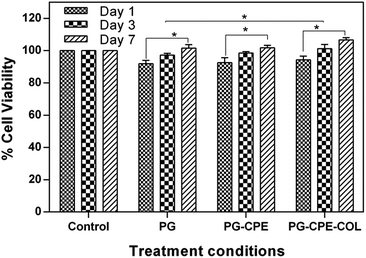 |
| Fig. 7 In vitro biocompatibility of the human (HaCaT) keratinocytes cell line over 1, 3 and 7 days using a MTT assay. The data are represented as the mean ± standard deviation; n = 3 (*p < 0.05). | |
The growth of fibroblasts provides uniform spreading of the cells with an increase in cell adhesion and proliferation in the nanofibrous matrix. The researchers found that unique property of the collagen matrix has attracted a certain type of cell types, mainly fibroblasts. The collagen-coated nanofibrous scaffold affects the fibroblast interaction with the collagen matrix. For example, Asaga et al.45 proposed an indirect interaction via cellular fibronectin between fibroblasts and collagen. However, the interaction depends not only on the collagen matrix and cells, but also on the interaction between the groups of cells and the surrounding matrix. This indicates that collagen emerges as biomimetic in nature to emphasize the function of the extracellular matrix.46 The high biocompatibility of the scaffold with a high degree of cell attachment in the scaffold was mainly due to the presence of fibroblast interaction with the collagen matrix.47,48 The cell proliferation and cell adhesion behavior of the NIH 3T3 fibroblast and keratinocytes cells on the surface of the nanofibrous matrix were assessed based on the four major steps: cell–material contact, cell–material interaction, cell attachment and spreading, which will precede better biocompatibility.49
In Fig. 8 and 9, both the cells were specifically studied using the Calcein AM-DAPI fluorescence staining. Furthermore, Calcein AM was associated with the live cell imaging, whereas DAPI was used to visualize the cells via cell nuclei staining over the nanofiber matrix. Both NIH 3T3 fibroblast and human keratinocytes (HacaT) cell lines showed good cell adhesion with a significant increase in cell amount compared to that of control cells. Both the cells exhibited an increase in cell growth onto the PG-CPE-COL nanofibrous scaffold. Moreover, the collagen coating on the nanofibrous matrix was beneficial and helps sustain cell adhesion with a major role in the cellular process. However, PG nanofiber matrix frames help achieve cell adhesion to the material surface via intracellular focal adhesion, spreading, proliferation and differentiation by mimicking the function of the extracellular matrix. The fluorescence microscopy analysis and cell viability investigation were congruent with the cell attachment and proliferation results.50 Ultimately, both cells were well spread and anchored throughout the nanofibrous matrix, exhibiting a stretched morphology with an increase in cell number. It is evident that increase in cell attachment and proliferation occurred in both the cells with the nanofibrous scaffold when the incubation period was between 12 h and 48 h, which allowed strong attachment with soft nanofibrous membrane.
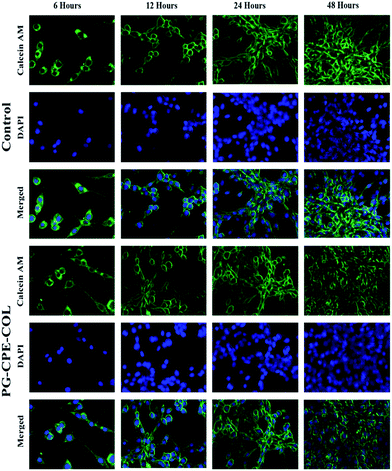 |
| Fig. 8 Calcein AM-DAPI fluorescence staining images of NIH 3T3 fibroblast cell adherence and proliferation onto the PG-CPE-COL nanofibrous scaffold in comparison to the control at 6, 12, 24 and 48 hours. The scale bar is 100 μm. | |
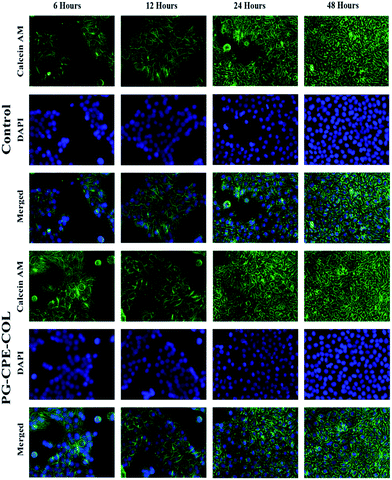 |
| Fig. 9 Calcein AM-DAPI fluorescence staining images of the human (HaCaT) keratinocytes cell adherence and proliferation onto the PG-CPE-COL nanofibrous scaffold in comparison to the control at 6, 12, 24 and 48 hours. The scale bar is 100 μm. | |
Antimicrobial activity of the scaffolds
The antimicrobial activity of the nanofibrous scaffold was evaluated against two bacterial strains. The CPE in the scaffold was found to undergo rapid neutralization of the plant compound in the wounded region and thus will not make a significant difference on the wound surface. Therefore, the scaffold without CPE did not form any zone compared with the PG-CPE-COL scaffold, as depicted in Fig. 10(a) and (b).
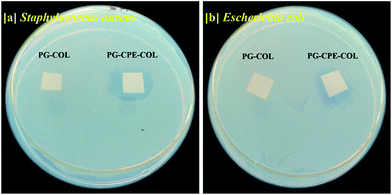 |
| Fig. 10 Antimicrobial activity of the nanofibrous scaffold: (a) Staphylococcus aureus and (b) Escherichia coli. | |
The PG-CPE-COL scaffold with a 0.3 mg/10 × 10 mm2 concentration of the CPE in the scaffold showed a clear zone of inhibition against both Staphylococcus aureus and Escherichia coli. The formation of a clear zone implies that CPE has active inhibition to prevent infection at the wound site. This makes the PG-CPE-COL nanofibrous scaffold a suitable dressing material in tissue engineering applications.31,34
Conclusion
In this study, the successful fabrication of PG-CPE-COL nanofibrous scaffold was achieved by electrospinning. The nanofibrous scaffold was found to have good physiochemical property and fiber morphology. The swelling behavior and porosity of the scaffolds was found to be suitable for a wound dressing material with better mechanical properties. The antibacterial property of the scaffold against both Gram positive and Gram negative bacteria was enhanced by the presence of CPE as compared with the scaffold without CPE. In vitro fluorescence staining clearly exhibits the cell adhesion and proliferation of the cells onto the nanofibrous scaffold. The collagen support helps in achieving better cell attachment over the nanofibrous matrix. Moreover, the excellent attachment of fibroblasts and keratinocytes over the nanofibrous scaffold highlights its potential application as a substrate in skin tissue engineering applications.
Acknowledgements
R. Giriprasath thanks the Council of Scientific and Industrial Research (CSIR) (award number 31/6(395)/2014-EMR-I), New Delhi, India, for providing the funds to carry out this study.
References
- C. He, W. Nie and W. Feng, J. Mater. Chem. B, 2014, 2, 7828 RSC
. - N. Goonoo, A. Bhaw-Luximon and D. Jhurry, RSC Adv., 2014, 4, 31618 RSC
. - R. Rajesh and Y. Dominic Ravichandran, RSC Adv., 2015, 5, 41135 RSC
. - P. Zahedi, I. Rezaeian, S.-O. Ranaei-Siadat, S.-H. Jafari and P. Supaphol, Polym. Adv. Technol., 2010, 21, 77–95 CAS
. - I.-S. Yeo, J.-E. Oh, L. Jeong, T. Seung Lee, S. Jin Lee, W. Ho Park and B.-M. Min, Biomacromolecules, 2008, 9, 1106 CrossRef CAS
. - E. Song, So Y. Kim, T. Chun, H.-J. Byun and Y. M. Lee, Biomaterials, 2006, 27, 2951 CrossRef CAS
. - E. Reichenberger and B. R. Olsen, Cell Dev. Biol., 1996, 7, 631–638 CrossRef CAS
. - V. Ottani, D. Martini, M. Franchi, A. Ruggeri and M. Raspanti, Micron, 2002, 33, 587–596 CrossRef CAS
. - J. M. Pachence, J. Biomed. Mater. Res., 1996, 33, 35–40 CrossRef CAS
. - M. Biondi, F. Ungaro, F. Quaglia and P. A. Netti, Adv. Drug Delivery Rev., 2008, 60, 229–242 CrossRef CAS
. - Y. W. Koo, H. J. Lee, S. Kim, N.-J. Song, J.-M. Ku, J. H. Lee, C. H. Choi, K. W. Park and G. H. Kim, RSC Adv., 2015, 5, 44943 RSC
. - A. M. Piras, F. Chiellini, E. Chiellini, L. Nikkola and N. Ashammakhi, J. Bioact. Compat. Polym., 2008, 23, 423 CrossRef CAS
. - T. Frier, C. Kunze, C. Nischan, S. Kramer, K. Sternberg, M. Sab, U. T. Hopt and K. H. Schmitz, Biomaterials, 2002, 23(13), 2649 CrossRef
. - N. Naveen, K. Ramadhar, S. Balaji, T. S. Uma, T. S. Natarajan and K. S. Praveen, Adv. Eng. Mater., 2010, 12, B380 CrossRef
. - D. I. Zeugolis, S. T. Khew, E. S. Y. Yew, A. K. Ekaputra, Y. W. Tong, L.-Y. L. Yung, D. W. Hutmacher, C. Sheppard and M. Raghunath, Biomaterials, 2008, 29, 2293 CrossRef CAS
. - C. S. Ki, D. H. Baek, K. D. Gang, Ki H. Lee, In C. Um and Y. H. Park, Polymer, 2005, 46, 5094 CrossRef CAS
. - A. P. Attanayake, K. A. P. W. Jayatilaka, C. Pathirana, L. Kumari and B. Mudduwa, Asian Pac. J. Trop. Dis., 2013, 3, 460 CrossRef
. - B. Deepti, K. Sasidhar, G. Sadhana, P. Srinivasa and S. Thaakur, Int. J. Res. Pharm. Sci., 2012, 3, 470 Search PubMed
. - S. O. Gonen, M. E. Taygun and S. K. çukbayrak, Chem. Eng. Technol., 2015, 38, 844–850 CrossRef CAS
. - M. Mashhadikhan, M. Soleimani, K. Parivar and P. Yaghmaei, Avicenna J. Med. Biotechnol., 2015, 7, 32–38 Search PubMed
. - B. Feng, H. Duan, W. Fu, Y. Cao, W. J. Zhang and Y. Zhang, J. Biomed. Mater. Res., Part A, 2014, 103, 431–438 CrossRef
. - N. Thuy Ba Linha, Y. Ki Minb and B.-T. Lee, J. Biomater. Sci., 2013, 24, 520–538 CrossRef
. - N. T. Ba Linh, K.-H. Lee and B.-T. Lee, J. Biomed. Mater. Res., Part A, 2013, 101, 2412–2423 CrossRef
. - N. T. Ba Linh, Y. Ki Min, Ho-Y. Song and B.-T. Lee, J. Biomed. Mater. Res., Part B, 2010, 95, 184–191 CrossRef PubMed
. - S. Yan, L. Xiaoqiang, L. Shuiping, W. Hongsheng and H. Chuanglong, J. Appl. Polym. Sci., 2010, 117, 542–547 CrossRef
. - H. Hajiali, S. Shahgasempour, M. R. Naimi-Jamal and H. Peirovi, Int. J. Nanomed., 2011, 6, 2133–2141 CrossRef CAS
. - P. Kuppan, S. Sethuraman and U. M. Krishnan, J. Biomater. Sci., 2014, 25, 574–593 CrossRef CAS
. - G. Ramanathan, S. Singaravelu, M. D. Raja, S. S. L. Sobhana, U. T. Sivagnanam and J. Biomater, Tissue Eng., 2014, 4, 203–209 CAS
. - R. M. Devendiran, S. K. Chinnaiyan, R. K. Mohanty, G. Ramanathan, S. Singaravelu and S. S. L. Sobhan, J. Biomater. Tissue Eng., 2014, 4, 430 CrossRef CAS
. - S. Singaravelu, G. Ramanathan, M. D. Raja, B. Sagar and T. S. Uma, Mater. Lett., 2015, 152, 90 CrossRef CAS
. - R. Zhao, X. Li, B. Sun, Y. Tong, Z. Jiang and C. Wang, RSC Adv., 2015, 5, 16940 RSC
. - T. Muthukumar, P. Prabu, K. Ghosh and S. Thotapalli Parvathaleswar, Colloids Surf., B, 2014, 113, 207 CrossRef CAS
. - G. Ramanathan, S. Singaravelu, M. D. Raja and U. T. Sivagnanam, Micron, 2015, 78, 28 CrossRef CAS
. - C. Wang, Z. Fan and Y. Han, J. Mater. Chem. B, 2015, 3, 5442 RSC
. - S. Kandhasamy, G. Ramanathan, J. Kamalraja, R. Balaji, N. Mathivanan, U. T. Sivagnanam and P. T. Perumal, RSC Adv., 2015, 5, 55075 RSC
. - N. Natarajan, V. Shashirekha, S. E. Noorjahan, M. Rameshkumar, C. Rose and T. P. Sastry, J. Macromol. Sci., Part A: Pure Appl. Chem., 2005, 42, 945 CrossRef
. - M. Kansiz, H. Billman and D. Mcnaughton, Appl. Environ. Microbiol., 2000, 66, 3415 CrossRef CAS PubMed
. - R. Ashokkumar and M. Ramaswamy, Int. J. Curr. Microbiol. Appl. Sci., 2014, 3, 395 CAS
. - G. Kim, M. Michler, G. H. Henning, S. Radusch and H. J. Wutzler, J. Appl. Polym. Sci., 2007, 103, 1860 CrossRef CAS
. - J. J. Deng, Y. L. Wang, L. X. Zhou, M. Gou, N. Luo, H. F. Chen, A. Tong, C. You and G. Guo, RSC Adv., 2015, 5, 42943 RSC
. - M. Lia, M. Ogiso and N. Minoura, Biomaterials, 2003, 24, 357 CrossRef
. - T. Liu, X. Ding, D. Lai, Y. Chen, R. Zhang, J. Chen, X. Feng, X. Chen, X. Yang, R. Zhao, K. Chen and X. Kong, J. Mater. Chem. B, 2014, 2, 6293 RSC
. - X. Zhu, W. Cui, X. Li and Y. Jin, Biomacromolecules, 2008, 9, 1795 CrossRef CAS PubMed
. - N. Nagiah, G. Ramanathan, T. S. Uma, L. Madhavi, R. Anitha and T. S. Natarajan, Adv. Polym. Technol., 2013, 32, 1 CrossRef
. - H. Asaga, S. Klkuchl and K. Yoshizato, Exp. Cell Res., 1991, 193, 167–174 CrossRef CAS PubMed
. - F. Grinnel, Trends Cell Biol., 2003, 13, 5 CrossRef
. - K. Anselme, C. Bacques, G. Charriere, D. J. Hartmann, D. Herbage and R. Garrone, J. Biomed. Mater. Res., 1990, 24, 689 CrossRef CAS PubMed
. - A. E. Postlethwaite, J. M. Seyer and A. H. Kang, Proc. Natl. Acad. Sci. U. S. A., 1978, 75, 871 CrossRef CAS
. - M. Moffa, A. Polini, A. Giovanna Sciancalepore, L. Persano, E. Mele, L. Gioia Passione, G. Potente and D. Pisignano, Soft Matter, 2013, 9, 5529 RSC
. - G. Vitiello, A. Luchini, G. DErrico, R. Santamaria, A. Capuozzo, C. Irace, D. Montesarchio and L. Paduano, Cationic, J. Mater. Chem. B, 2015, 3, 3011 RSC
.
|
This journal is © The Royal Society of Chemistry 2016 |