DOI:
10.1039/D4RA00976B
(Review Article)
RSC Adv., 2024,
14, 11284-11310
Comprehensive assessment of carbon-, biomaterial- and inorganic-based adsorbents for the removal of the most hazardous heavy metal ions from wastewater
Received
7th February 2024
, Accepted 21st March 2024
First published on 9th April 2024
Abstract
Owing to the high cost of recycling waste, underdeveloped countries discharge industrial, agricultural, and anthropogenic effluents without pretreatment. As a result, pollutant-loaded waste enters water bodies. Among the diverse toxic contaminants, heavy metal ions are the most detrimental because of their chronic toxicity, non-degradability, prevalence, and bioaccumulation. The growing shortage of water resources demands the removal of heavy metal ions from wastewater. Three SDGs of the sustainability agenda of the United Nations appeal for clean water to protect life beneath water and on land depending on the water sources. Therefore, efficient environmentally friendly approaches for wastewater treatment are urgently required. In this regard, several methods have been developed for the removal of heavy metal ions from wastewater, including adsorption as the most widely used method owing to its eco-friendly, cost-effective, and sustainable nature. The present review discusses the progress in the preparation and application of various adsorbents based on carbon, micro-organisms, agricultural waste and inorganic materials for the extraction of toxic metal ions such as Pb2+, Cr6+, As3+, As5+, Hg2+ and Cd2+. Herein, we provide information on the role of the homogeneity and heterogeneity of adsorbents, kinetics of the adsorption of an adsorbate on the surface of an adsorbent, insights into adsorption reaction pathways, the mechanism of the sorption process, and the uptake of solutes from solution. The present review will be useful for researchers working on environmental protection and clean environment.
1. Introduction
The pervasive presence of toxic heavy metals in different ecosystems poses environmental challenges, which needs to be promptly addressed to safeguard both human health and ecological balance. It is a public obligation to develop methods for treating wastewater to protect water dwelling species and human life. Heavy metal ion water contamination is one of the most serious environmental issues. It is the result of inappropriate, unregulated industrial wastewater and agricultural and irrigation drainage schemes that dump sewage directly into water bodies. This irresponsible approach of waste disposal has led to the concentrations of water pollutants exceeding the recommended safety limit. For example, the steel sector releases lead ions. Lead is also released into the environment from acid batteries, the combustion of leaded gasoline, the illegal use of tetraethyl lead as an anti-knock agent in gasoline, volcanic eruptions and natural weathering of rocks.1 Cadmium enters the environment through mining activities, coal combustion and excessive use of chemical fertilizers.2 The eruption of volcanoes and weathering of rocks are natural sources introducing heavy metals into the environment. Anthropogenic sources include industrial waste, combustion, mining, casting, and the use of pesticides and fertilizers. Water contamination has serious consequences on marine life, public health, and food quality. Accordingly, if proper pretreatment approaches are not adopted, then the scarcity of clean water and toxicity of seafood will become a global challenge in the near future.
The advent of industrialization and urbanization is significantly beneficial to the society, but together with its numerous advantages, it also poses serious problems.3,4 Toxic metal ions not only contaminate the water present in seas, lakes, ponds and reservoirs but also reach the underground water. Heavy metals can be absorbed by living organisms due to their high solubility in the aquatic environment. Consequently, heavy metals enter the food chain, posing a serious threat to the ecological balance. Even minor exposure to heavy metals can lead to dermatological problems and respiratory diseases. Furthermore, when the uptake of these metals exceeds the permissible limits, they cause serious health disorders.5–7 Hence it is crucial to monitor and regulate heavy metal exposure to safeguard human well-being. The World Health Organization (WHO) and US Environmental Protection Agency (US EPA) have set permissible limits for heavy metals in drinking water. These guidelines are crucial for ensuring the safety of communities by minimizing exposure to harmful substances. Numerous elements fall into the category of heavy metals but in this review, we focus on the top five toxic metals including mercury, cadmium, lead, arsenic and chromium and understanding their impact, which is crucial for effective management and mitigation strategies.
Cadmium is present in the Earth's crust, but anthropogenic activities such as electroplating, mining, stabilizing plastics, alloy, cement, pigment, and battery manufacturing, fossil fuel combustion, use of high-phosphate fertilizers, and municipal and sewage sludge incineration yield a huge amount of cadmium. Specifically, 150–2600 tons of cadmium is released into the environment annually.8 Similarly, due to the volatile nature of mercury, it is mostly used in batteries, thermometers, barometers, and dental amalgams. Mercury can exist in three forms, i.e., elemental form (Hg), organic form (CH3Hg), and inorganic form (Hg2+). It was reported by the United Nations Environment Program (UNEP) that in 2015, around 2200 tons of mercury was emitted from anthropogenic sources, which included the iron and steel industry, gold industry, and direct mercury production industries. Forest fires and volcanos are natural sources of mercury.9,10 Arsenic is a naturally occurring metalloid having a germicidal nature and resistance to decay. Due to this property, it is used as an insecticide, herbicide, and also the preservation of wood.11 Similar to mercury, chromium also exists in three thermodynamic stable forms Cr(0), Cr(III), and Cr(VI). The metallic form of chromium is chiefly used in iron-based alloys. The chemical industry, production of dyes, wood preservation, leather tanning, chrome plating, and manufacturing of various alloys utilize chromium, and then discharge it without processing, leading to environmental pollution.12 Lead is widely applied in various industries such as production of acid batteries, production of lead additives for gasoline, ceramic and glass manufacturing, metal planting and finishing, printing, and tanning.13,14 This is the first report presenting the sources of metal-based water contaminants, their toxicity mechanisms and methods for their efficient removal by recoverable adsorbents to avoid any chances secondary pollution. The current review presents remediation technology based on environmental-friendly protocols and is expected to advance renewable and sustainable water treatment strategies. This review is expected to play a critical role in the implementation of solutions for controlling pollution levels, which is necessary to protect human health and ensure the quality standards of aquatic environment.
2. Harmful effects of heavy metal ions
Various routes facilitate the entry of heavy metals into the human body including dermal exposure, inhalation and ingestion via contaminated food or drinking water. Overall, the effects of heavy metal ions on different parts of human body are shown in Fig. 1.15 Once inside the body, these metals can react with chlorine or oxygen and exert toxic effects. Prolonged exposure to heavy metals may result in their substitution for vital elements within the body such as calcium substituting lead, zinc replacing cadmium and the majority of trace elements substituting aluminum. The accumulation of heavy metals causes an imbalance in antioxidants. Enzymatic function and hormonal activity are also influenced by exposure to heavy metals. Studies have reported that oxidative stress is produced due to the generation of reactive oxygen species irrespective of which molecular pathway is undertaken. Oxidative stress leads to kidney damage, cancer, neurological disorders and abnormalities related to the endocrine system.16 Besides the various benefits of heavy metals in plants and humans, these heavy metals are also responsible for the malfunctioning of cells by displacing the original metals from their natural binding sites in proteins, which ultimately leads to toxicity. The binding of heavy metals to the nuclear protein and DNA is the prime reason for the oxidative deterioration of biological macromolecules.17
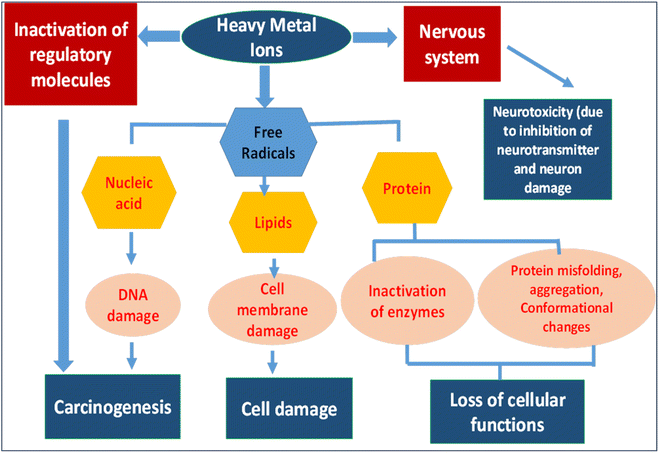 |
| Fig. 1 Harmful effects of heavy metal ions on human beings.15 | |
Cadmium (30 mg kg−1) has been reported to cause acute toxicity, which primarily includes abdominal discomfort, headaches, muscle cramps, fatigue, and even death. However, its dangerous effect on various organs in the body such as the lungs, liver, placenta, and endocrine tissues, kidney damage, skeletal and cardiovascular dysfunctions, and reproductive problems at higher concentration has also been reported. Cadmium replaces calcium in minerals due to their comparable ionic radius and chemical behavior, consequently reducing the uptake of Ca and affecting the bones.8 Cd also affects the biological activity of terrestrial and aquatic organisms. In plants, cadmium causes chlorosis and stunted growth. Moreover, it not only decreases the chlorophyll content and photosynthetic activity but also inhibits carbon fixation in plants. Osmotic stress in plants is also induced due to exposure to Cd in soil. This results in a decrease in the relative water content in leaves, stomatal conductance, and transpiration. Damage to plant membranes and destruction of cell biomolecules and organelles are consequences of the overproduction of reactive oxygen species (ROS) induced by the presence of cadmium.18 The oxidative stress may be attributed to an imbalance in the production of ROS and the ability of plants to detoxify them.
Mercury can exist in three forms, i.e., elemental form (Hg), organic form (CH3Hg), and inorganic form (Hg2+). Elemental mercury in vapor form can easily be absorbed by the lungs and causes damage to the body. Among the three different forms of mercury, the organic form (CH3Hg) causes neurological alterations in humans due to the increase in reactive oxygen species. Reactive oxygen species are also responsible for injuries in the central nervous system (CNS). The organization of microtubules, which is important in CNS development, is also inhibited by methylmercury. Inorganic mercury mainly causes cardiotoxicity.9,10 Heavy metals also affect the biological activity of terrestrial and aquatic organisms. For instance, Cd and Hg cause chlorosis and shunted growth in plants. The fixation of carbon is also inhibited due to presence of Cd, together with a decrease in chlorophyll content and photosynthetic activity. Osmotic stress in plants is also induced due to exposure to Cd in soil. Damage to plant membranes, destruction of cell biomolecules and organelles is also reported due to the excessive generation of reactive oxygen species. Boston fern (Nephrolepis exaltata) and Indian mustard (Brassica juncea) after exposure to Hg show loss of cell shape, decrease in intercellular spaces, and vascular abnormality in their leaves. The amount of chlorophyll is also reduced because the biosynthesis of chlorophyll depends on the activity of NADPH, where protochlorophyllide oxidoreductase (POR) is inhibited by Hg stress.19,20
Arsenic has the ability to attach to small particles in the air and remain suspended in the air for prolonged periods. It can change its forms and interaction with oxygen or other molecules present in the air, water, or soil, as well as with soil bacteria. The toxicity of arsenic depends on its oxidation state. The toxicity of arsenic has been reported to follow the order of AsH3 > As(III) > As(V) > organic As.11 Arsenic is classified as a class 1 human carcinogen by The International Agency for Research on Cancer. Drinking water containing arsenic causes skin cancer and lung, bladder, liver, and kidney damage. Non-insulin-dependent diabetes mellitus is also caused by increased exposure to arsenic.21 Peripheral neuropathy is the consequence of long-term exposure to inorganic arsenic. Changes in behaviors, confusion, disorientation, memory loss and cognitive impairment are other effects associated with arsenic.22
Chromium(VI) is carcinogenic and the most mobile form of chromium in the environment. The chemical form of this metal, its oxidation state, and the route of exposure determine the type of toxicity in humans. Cr6+ reacts spontaneously with intracellular reductants after entering the cell and generates Cr5+ or Cr4+, free radicals, and Cr3+ eventually. The limited membrane permeability of Cr3+ causes it to be retained inside the cell and interact with DNA. Reactive oxygen species are generated after the one-electron redox reaction of Cr5+, which react with DNA–protein multiplexes, triggering multiple possible apoptotic signaling pathways in various cell types. Also, Cr causes irritation and inflammation of the skin.23
Lead poisoning has common impacts, which include severe damage to the kidneys, liver, brain, reproductive system, and nervous system. Sterility, abortion, and neonatal death are caused by long-term exposure to lead. An elevated blood-lead level is the root cause of the rise in mortality and alteration of puberty in girls. In children, it is reported that exposure to lead causes impaired development, lower intelligent quotient, shortened attention span, hyperactivity and mental systolic blood pressure together with causing hearing impairment, intelligential impediments, and deterioration.14,24
When heavy metals enter the body through ingestion, upon reaching the stomach, they undergo conversion into their stable oxidation states (Zn2+, Pb2+, Cd2+, As2+, As3+, and Hg2+) due to the presence of acid in the stomach. These stable oxidation states react with the biomolecules in the body and form stable bonds. One of the most common bonding groups is the thiol group. This interaction plays a crucial role in the bioavailability and toxicity of heavy metals within the body.
Heavy metals have been reported to replace hydrogen or the methyl group in enzymes or proteins and inhibit their normal functioning.21 Heavy metal-bound enzymes are not utilized by the body. Consequently, these enzymes are inactivated and their persistence in the body causes various abnormalities and internal damage.25,26 The aggregation of proteins is also caused by heavy metals.25,26 Hence, to protect living organisms, it is imperative to find effective approaches for wastewater purification from lethal heavy metal ions. Herein, we present numerous adsorbents for addressing the challenges related to the removal of heavy metal ions, which are potential hazards for creatures living on land and in water bodies.
3. Techniques for the removal of heavy metals
To mitigate the effects of heavy metals, various methods have been employed for their removal. The methods used for the removal of contaminants include biological treatment, coagulation membrane, filtration, electrochemical treatment, electrodialysis, ion-exchange, photocatalysis, oxidation and adsorption, as shown in Fig. 2.27–29 Over the past few decades, intensive efforts have been devoted to the development of cheaper and more effective technologies and sorbent materials to improve the quality of treated effluents.6 Each method has its own advantages and disadvantages. Conventional methods have major disadvantages such as incomplete removal, high-energy requirements, and production of toxic sludge. Thus, the selecting of a suitable technique depends on the characteristics of the effluents, targeted specific heavy metal, scale of treatment, standards promulgated by government agencies and cost effectiveness. Fig. 2 presents the various treatment techniques for water purification.30
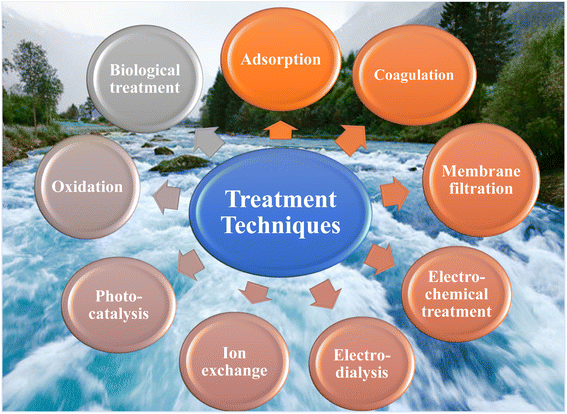 |
| Fig. 2 Treatment techniques for the removal of heavy metals from water. | |
This work reviews the adsorption method for the removal of heavy metals ions from wastewater. This water treatment approach has garnered significant attention in recent years. Adsorption treatment of heavy metals is preferred due to its significant metal removal efficiency, easy operation, low sludge production, and utilization of low-cost adsorbents.31,32 However, the adsorbents eventually become saturated with metal ions, and hence developing efficient regeneration methods is crucial. This is a comprehensive review on the removal of five hazardous heavy metals (Pb2+, Cr6+, As3+, As5+, Hg2+ and Cd2+) via synthetic and natural adsorbents.
4. Adsorption process
Adsorption is a surface phenomenon in which pollutants are adsorbed on the surface of a solid substance. The substance that becomes adsorbed on the solid surface is termed the adsorbate, while the solid surface itself is referred to as the adsorbent. Adsorption can be monolayer or multilayer depending on the applicable model (Langmuir or Freundlich). The Langmuir isotherm describes a monolayer adsorption process, in which the adsorbate is directly attached to the surface of the adsorbent. Alternatively, the Freundlich isotherm is a widely used model to describe the multilayer adsorption on heterogeneous surfaces. Typically, physical forces drive adsorption although weak chemical bonds may also play a role. Three major steps are involved in adsorption including diffusion of the adsorbate from solution towards the surface of the adsorbent, adsorption on the solid surface and diffusion inside the adsorbent particles.
Adsorption is broadly classified into two types, i.e., physico-sorption and chemisorption, as shown in Fig. 3.33 In the case of physico-sorption, the adsorbate adsorbs on the surface of the adsorbent through weak van der Waals forces. This type of adsorption is reversible but not specific. Alternatively, in chemisorption, chemical bonds (ionic or covalent bond) are formed between the adsorbate and adsorbent, which make them irreversible due to chemical reaction. In the adsorption process, different adsorbing materials are used such as synthetic and natural materials. The term bio-sorption is used for the type of adsorption in which the adsorbate adsorbs on natural materials (biological systems).34 Herein, we focus on carbon-based materials, biomaterials and inorganic materials for the removal of the five most hazardous heavy metals for wastewater treatment.
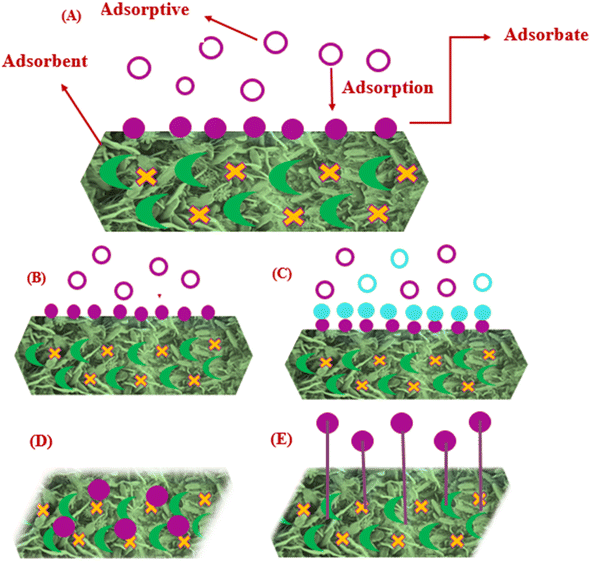 |
| Fig. 3 (A) General mechanism of adsorption, (B) monolayer adsorption, (C) multilayer adsorption, (D) physical adsorption, and (E) chemical adsorption. | |
4.1. Factors affecting the adsorption process
The adsorption process is influenced by several key parameters including temperature, nature of the adsorbate and adsorbent, pH, concentration of pollutants, contact time, synthesis route, and particle size.
4.1.1. Influence of pH. The adsorption capacity of heavy metals is affected by a change in pH. The pH value at which the charge on the adsorbent surface is zero is termed pHPZC (point of zero charge). When the pH is lower than pHPZC, the adsorbent surface becomes positively charged. As a result, it attracts anionic species from the solution. Alternatively, when the pH is greater than pHPZC, the adsorbent surface becomes negatively charged. This charge facilitates electrostatic interactions, particularly with cationic species such as heavy metals. Consequently, the adsorption capacity of metals is enhanced at higher pH compared to lower pH.35 Understanding this pH-dependent behavior is crucial for optimizing the adsorption process in water treatment and environmental remediation.
4.1.2. Influence of contact time. The removal of heavy metals is also affected by the contact time. Initially, the removal efficiency increases with an increase in contact time, but after reaching the maximum value, a further increase in contact time does not yield significant improvements. This phenomenon occurs because initially, the concentration gradient is high and the adsorption sites are vacant.36 However, as saturation occurs, additional contact time does not lead to a substantial enhancement in adsorption. During the adsorption of Pd(II) on a cryogel, it was observed that in the first 2 h, the adsorption capacity reached 140 mg g−1, while 360 min was the longest time to reach the point of equilibrium. The results of this test prove that during the initial stages, the adsorption capacity increases with an increase in contact time because of the greater availability of binding sites, and finally reaches equilibrium when the active sites are fully occupied.37
4.1.3. Influence of porosity of adsorbent. Various porous materials have been employed for the adsorption of pollutants from water bodies. Porosity is an important factor to be considered during the development of any adsorbent because large pores can facilitate the diffusion of pollutants, which is beneficial to reach the targeted functional groups.38
4.1.4. Influence of adsorbent dose. To obtain economical optimization in an adsorption system, the adsorbent dose plays a crucial role. Generally, the removal efficiency increases with higher adsorbent doses due to the enhanced availability of adsorption sites.39 However, at the same time, a decrease in metal adsorption capacity has also been reported at a very high adsorbent dose. For instance, the copper adsorption capacity decreased when the concentration of the adsorbent increased up to 40.5 mg g−1. This is due to the partial aggregation of the adsorbent particles.40
4.1.5. Influence of temperature. Temperature significantly influences adsorption and its effects vary based on whether the process is exothermic or endothermic. When the process is exothermic, an increase in temperature retards the adsorption capacity. Specifically, higher temperatures hinder the adsorption of substances. Conversely, in an endothermic process, an increase in temperature increases the adsorption capacity. At elevated temperatures, heavy metal ions exhibit mobility, and more active sites are available for adsorption.41
4.1.6. Influence of initial concentration of adsorbate. The initial concentration of heavy metals significantly influences the extent of adsorption and is considered a key parameter for evaluating the efficiency of an adsorbent. Generally, with an increase in the initial concentration of heavy metal ions, the removal percentage decreases. However, an increase in the initial concentration of heavy metal ions corresponds to an increased adsorbent capacity. This inverse relationship between removal percentage and initial concentration stems from the saturation of the adsorption sites on the adsorbent surface. The direct relation between the adsorbent capacity and initial concentration of heavy metal ions originates from the strong driving force for mass transfer.42
4.1.7. Influence of particle size. Particle size has a prominent effect on the adsorption performance. Particles with a smaller size have greater adsorption efficiency in comparison to larger particles. This foundation is based on surface area. Smaller particles have a greater surface area in comparison to larger particles. Therefore, smaller particles are always considered more suitable for adsorption in comparison to larger particles.43
4.1.8. Influence of synthesis route. The synthesis route is a prominent factor that affects the adsorption performance. This factor can be explained as follows: in the case of conventional polymers synthesis, higher or room temperature is used but the fabricated polymers have a condensed and packed structure, in which the diffusion of pollutants is very difficult to reach the targeted functional groups. Alternatively, cryogels (porous polymers) prepared at a negative temperature (temperature below the freezing point of the solvent) have the ability to facilitate the easy transport of pollutants to the targeted point. This observation confirms that the synthesis route has a prominent effect on the adsorption process.44
4.2 Equations related to isotherms, kinetics and thermodynamic study of adsorption
Isotherm studies explore the homogeneity and heterogeneity of adsorbents. An adsorption isotherm represents the relationship between the amount of pollutant adsorbed and its concentration in water at equilibrium.45 Specifically, the Langmuir adsorption isotherm assumes that the active sites are homogenously distributed. When a site is occupied, further adsorption ceases. This model is particularly applicable to monomolecular layers.46 The separation factor RL is used to access the favorability of adsorption based on the initial concentration of the adsorbate, which can be defined as follows:
where RL is a dimensionless constant. If RL is between 0 and 1, it means that the process is favorable, whereas if equal to zero, then adsorption is irreversible. Linear adsorption is indicated by RL equal to 1. RL greater than one shows that the process is unfavorable.47 Similarly, adsorption occurring on a heterogeneous surface is investigated using the Freundlich isotherm. According to Freundlich, the adsorption isotherm becomes linear, indicating favorable adsorption when n = 1, favorable when n < 1, and unfavorable when n > 1.48 The distribution of the binding energy can be explained by the Temkin model. This model considers the variation in the heat of adsorption across the surface. The temperature dependence is related to the Dubinin–Radushkevich isotherm. It also estimates the free energy of a heterogeneous surface and porosity of the adsorbent. The nature of biosorption can be determined, which refers to the adsorption of biological materials on surfaces. If the sorption energy value is in the range of 8 to 16 kJ mol−1, it suggests chemical or ion exchange, whereas for energies less than 8 kJ mol−1, physical adsorption is dominant.41 The value of the linear regression correlation coefficient R2 indicates which model is appropriate to give the best fit.
To understand the adsorption process, investigating the rate at which the adsorbate adsorbs on the surface of the adsorbent is crucial. The major kinetic models employed in adsorption studies include pseudo-first-order, pseudo-second-order, intraparticle model, and Elovich model. Additionally, thermodynamic studies estimate the changes in enthalpy, entropy and Gibbs free energy, which are essential for evaluating the feasibility of the process.49 The linear forms of different isotherms, kinetics models, and thermodynamic study used to determine the different parameters related to adsorption are presented in Table 1.
Table 1 Linear forms of different equations used in isotherm, kinetic, thermodynamic and batch studies
Equations used in adsorption study |
Isotherms |
Kinetic equations |
Batch study |
Thermodynamic equations |
Langmuir isotherm |
Pseudo-first-order |
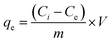 |
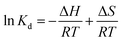 |
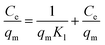 |
ln(qe − qt) = ln qe − K1t |
Freundlich isotherm |
Pseudo-second-order |
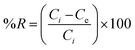 |
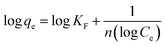 |
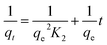 |
Temkin isotherm |
Elovich model |
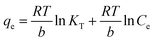 |
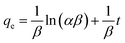 |
Dubinin Radushkevich isotherm |
Intraparticle model |
ln qe = ln qm − KDR ε2 |
 |
A variety of techniques such as UV-visible spectrophotometry, atomic absorption spectrophotometry, and inductive coupled plasma spectrometry is used for the measurement of the adsorbate concentration in the liquid phase. UV-visible spectrophotometry is based on the principle of the Beer–Lambert law and is the simplest and most cost-effective method for the detection of heavy metals. The common heavy metals quantified using this technique include Ni2+, Cu2+, Cr3+, Cr6+, Fe2+, and Fe3+.50 Atomic absorption spectrophotometry requires the sample to be in atomic gas form and can determine concentrations of over 65 elements. It exhibits high sensitivity, especially when measuring at parts per million (ppm). Another method employed for measuring the concentration of adsorbates in the liquid phase is inductively coupled plasma spectrometry. It is comprised of two further modifications, i.e., inductively coupled plasma mass spectrometry (ICP-MS) and inductively coupled plasma atomic emission spectrometry (ICP-AES). ICP-MS is 10–100 times superior to ICP-AES.43 The adsorbents utilized can originate from mineral, organic or biological sources, including zeolites, industrial by-products, agricultural waste, biomass, and polymeric materials. Adsorbents can be broadly classified into natural adsorbents, bio-adsorbents, commercially available adsorbents and adsorbents made from agriculture and industrial waste, as depicted in Fig. 4.51
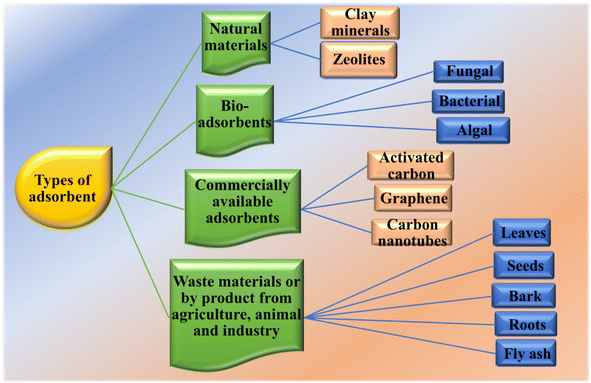 |
| Fig. 4 Types of adsorbent and their sources. | |
Commercially available adsorbents predominantly consist of carbon-based adsorbents such as graphene, carbon nanotubes, and activated carbon. Graphene has a two-dimensional (2D) structure and is available in various forms such as pristine graphene, graphene oxide and reduced graphene oxide. When rolled up, graphene sheets form carbon nanotubes, which may be single walled or multi-walled depending on the number of rolled-up graphene sheets. All these materials fall in the category of nanomaterials and can be employed as adsorbents for the removal of heavy metals due to their stable nature and high surface properties.
Inorganic-based adsorbents primarily include natural adsorbents such as clays, zeolites and silica. Mesoporous silica is characterized by a regular two-dimensional hexagonal array of channels. It has a highly ordered structure, which can be modified with functional groups such as carboxylic acid and sulfonic acid. Zeolites are employed as adsorbents due to their good ion exchange properties, high surface area and hydrophilic character, which make them suitable for the removal of metals, e.g. cadmium. It has been observed by researchers that modified zeolites show better efficiency compared to natural zeolites.52
Adsorbents made from agriculture waste are used as alternatives to conventional methods for the removal of pollutants from water bodies due to their cost effectiveness, highly efficient and environment-friendly nature, reduction of biological or chemical sludge, absence of need for extra nutrients, biosorbent regeneration, and potential for metal recovery. Ionizable functional groups such as carboxyl, amine, and hydroxyl create possible adsorption binding sites. The major components of agricultural waste biomass include hemicellulose, proteins, simple sugars, lignin, extractives, lipids, water hydrocarbons, and starch, which contains different functional groups that facilitate the formation of complexes with metals. To enhance the adsorption capacity of adsorbents, some researchers also modified the adsorbents by functionalizing them. Because coal-based AC is limited in its usage due to its high cost, multiple strategies have been used to create activated carbon from diverse agriculture-based sources. The selection of agriculture waste-based adsorbents is based on many factors such as local availability, easy desorption, high regeneration capability, high binding capacity and selectivity for heavy metals, cost-effectiveness, and the negligible release of unrequired compounds into aqueous solutions.53,54
Algae, fungi, bacteria and yeast can also be used as adsorbents, which are termed as bio-adsorbents. These microorganisms play a crucial role in sequestrating pollutants from water bodies. Their enzymatic activity enables them to transform or degrade pollutants. Non-living biomass needs simple and cost effective treatment. Whether free or immobilized, both forms can be used as microbial biomass. The resultant efficacy rates are influenced by environmental factors, physicochemical characteristics of the microbes used, and the target environments.55,56
Various other materials serve as adsorbents including biopolymers, biopolymeric nanocomposites, metal organic frameworks, covalent organic frameworks, and organic membranes. Biopolymers are more selective towards the adsorption of heavy metal ions with cellulose derived from the cell wall of various plants, being a common choice as a biopolymer. Chitosan, which is obtained by de-acetylating chitin, and sodium alginate, extracted from brown algae, are also notable adsorbents for heavy metal ions.57 Employing cheap and renewable materials such as chitosan-calcium carbonate nanocomposites (Cs/CaCO3) show significant adsorption capacity of 62.11 mg g−1 for Cr(III), 83.33 for Cd(II), and 98.03 for Pb(II) within short removal times.58
Metal organic frameworks (MOFs) are organic–inorganic hybrids composed of metal clusters and organic linkers. MOFs have variable porosity and high surface area, making them versatile for applications such as sensing, drug delivery, adsorption and water splitting. Shi-Wen Lv et al. synthesized MIL-101-NH2, which showed an adsorption capacity of 1.1 mM g−1 for Pb(II) ions.59,60 Similarly, UiO-66-NHC(S)NHMe exhibited a remarkable uptake capacity of 769 mg g−1 for Hg(II) ions.61 Nathan D. Rudd et al. synthesized luminescent metal–organic frameworks (LMOFs) for the detection and removal of the metal ions. The as-synthesized LMOF-263 was reported to demonstrate an adsorption capacity of 380 mg g−1 for Hg(II) ions.62 The rapid and selective removal of Hg(II) and Pb(II) ions were achieved using a metal–organic framework/polymer composite, i.e., Fe-BTC/PDA. This cos-effective and water-stable composite exhibited an uptake efficiency of 1634 mg g−1 for Hg(II) and 394 mg g−1 for Pb(II) ions.63 Similarly, covalent organic frameworks (COFs) typically consist of low molecular weight elements with robust covalent linkages. Their porous nature, tunable pore size, crystallinity, and easily tailored functional groups enable them to be used as adsorbents for heavy metal ions. In this regard, TPB-DMTP-COF-SH showed the highest adsorption capacity reported thus far for the removal of Hg(II) and Sn(II) ions at rates of 4395 and 4350 mg g−1, respectively.64
Membrane technology offers promising avenues for the separation of a wide variety of pollutants from wastewater due to their tailored pore size. However, many commercial membranes currently available are non-degradable, posing environmental concerns. Biodegradable plastics have gained attention owing to their ability to degrade under controlled conditions. Although biodegradable plastics have superior properties compared to conventional polymer membranes they have low mechanical, thermal and water stability. Thus, to overcome these limitations, novel approaches for membrane development are being explored, including blending of nanoparticles with polymers, copolymerization and cross-linking. Additionally, emerging biodegradable plastics other than cellulose and chitosan are gaining attention for their potential applications in wastewater treatment, including poly(butylene succinate)-based membranes, poly(ε-caprolactone)-based membranes, poly(lactic acid)-based membranes and poly(hydroxyalkonate)-based membranes.65 These advancements hold promise for the development of more sustainable and effective membrane technologies.
5. Carbon-based adsorbents
Carbonaceous materials such as activated carbon (AC), biochar, carbon nanotubes (CNTs), and graphene oxide (GO) are considered the most cost-effective and highly efficient materials for the removal of heavy metals from various environmental matrices. Their favorable surface properties, uniform adsorption, non-toxic nature, ease of synthesis, and high adsorption capacities have prompted researchers to investigate the use of carbon-based materials as adsorbents.66 CNTs exhibit a stable nature, large specific area and excellent mechanical and electrical properties. These attributes have attracted attention from researchers for utilizing them for the removal of heavy metals.67 Similarly, GO possesses a huge surface area and oxygenated functional groups. Researchers are keen on employing GO as an adsorbent for the removal of heavy metals. Coal-based AC is expensive, limiting its widespread use. Thus, concerted efforts have been employed to develop AC from various sources beyond coal. Any material with a high carbon content can potentially yield activated carbon.68 The adsorption of heavy metals on carbon-based adsorbents can take place via different mechanisms, which are influenced by the functional groups present on the surface of adsorbents. The major functional moieties include hydroxyl, amine and carboxyl groups (see Fig. 5). These carbon-based materials offer diverse options for efficient heavy metal removal, each with their unique advantages and applications. Adsorption can be physisorption or chemisorption depending on the type of interaction the between adsorbent and adsorbate. The large surface area and highly porous nature of carbon-based adsorbents are helpful for the formation of van der Waals interactions between heavy metal ions and adsorbents. Functional groups such as carboxyl, which bear the opposite charge to the adsorbate (heavy metal ions) are responsible for the electrostatic interaction between them. Sometimes the functional moieties present on the surface of adsorbents have exchangeable ions, for example, in the carboxyl group, hydrogen can be replaced by the neighboring metal ions, which is known as the ion exchange mechanism. To enhance the adsorption capacity of carbon-based adsorbents, metal binding agents are used to modify their surface. These modifications lead to the precipitation and redox mechanisms. If the thiol group is attached on the surface of adsorbent, it will facilitate the precipitation of lead ions. If the surface is modified by a reducing agent or oxidizing agent, then the metal ion will either oxidize or reduce itself, respectively.69
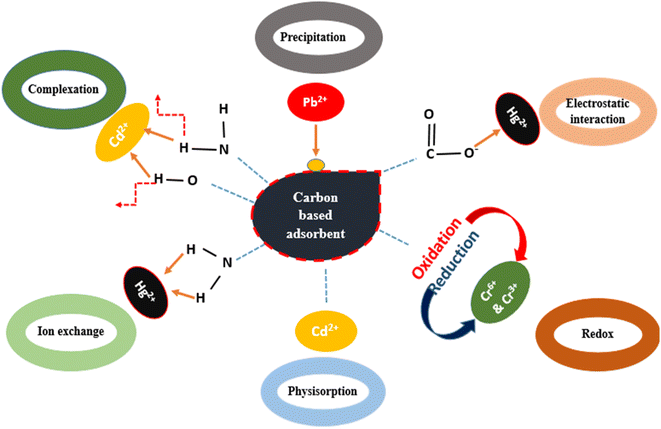 |
| Fig. 5 Mechanism of adsorption of heavy metals on carbon-based adsorbents. | |
Numerous researchers have implemented carbon-based materials for the removal of heavy metal ions for wastewater. Bandaru et al. synthesized thiol-derivatized single-walled carbon nanotubes (SWCNT-SH) and investigated their potential as an adsorbent for the removal of Hg(II) ions. Notably, the Langmuir parameter showed a maximum adsorption capacity of 131 mg g−1 for the functionalized SWCNTs, highlighting their effectiveness to treat mercury pollution. The enhanced adsorption capacity for Hg(II) ions is due the presence of thiol groups, which undergo the selective formation of a complex with Hg(II) ions via soft acid-soft base interaction.70 Another study highlighted the efficacy of calf/cow bone charcoal (CBC) as an adsorbent for removing Hg(II) ions from water. With a surface area of 112 m2 g−1, CBC demonstrated the maximum adsorption capacity of 38.08 mg g−1. The thermodynamic study showed the endothermic, spontaneous and reversible nature of adsorption.71 Similarly, many other carbonaceous materials demonstrate the ability to remove Hg(II) ions by adsorption including camel bone charcoal,72 sheep bone charcoal,73 and coconut shell-based granulated activated carbon.74
Janik et al. synthesized various amino silanes containing one, two, or three nitrogen atoms in the molecule for the modification of GO. Interestingly, despite having a different number of nitrogen atoms, the resulting GO derivatives exhibited similar maximum adsorption capacities for Cr(VI) in the range of 13.3–15.1 mg g−1. The detection limit was found to be 0.17 ng mL−1. The adsorption process followed a pseudo-second-order rate kinetic model, and the Langmuir and Freundlich isotherm data showed that adsorption is monolayer. Specifically, the anionic species of Cr(VI) form electrostatic interactions with the protonated amino groups on the surface of GO derivatives.75 Similarly, AC-functionalized multi-walled carbon nanotubes (AC/f-MWCNTs) have excellent potential for chromium resistance. The highest adsorption capacity achieved using AC/f-MWCNTs as an adsorbent was 113.29 mg g−1.76 Another study reported that GO prepared from the chemical exfoliation and oxidation of layered graphite showed the adsorption capacity of 240.3 mg g−1. The strong adsorptive interaction between the GO surface and Cr(VI) is confirmed by the adsorption energy (290.32 kcal mol−1) for Cr(VI).77 Similarly, graphene oxide functionalized with alpha cyclodextrin and modified with polypyrrole (GO-αCD-PPY NC) showed the maximum adsorption capacity of 666.67 mg g−1 at 45 °C for the removal of Cr(VI) ions.78
Šolić et al. reported that ox-MWCNTs act as an effective adsorbent for the removal of Cd(II). The duration of the functionalization processing impacted the capacity of the adsorbent. Thus, ox-MWCNTs functionalized for 6 h showed the maximum adsorption capacity of 13.5 mg g−1 for Cd(II).79 In another study, MWCNTs used as an adsorbent demonstrated an impressive adsorption capacity of 181.81 mg g−1 for the removal of Cd(II) ions.80 Alsaadi and team synthesized novel adsorbents for the removal of As3+ from water. This study used a deep eutectic solvent (DES) as the functionalization agent for CNTs. KM-CNTs (CNTs functionalized with KMnO4 and MTPB-based DES) showed the maximum adsorption capacity of 23.4 mg g−1.81 Besides this, microwave-assisted economic MWCNTs having a surface area of 9.1 m2 g−1 showed 92% and 91% removal of arsenate and arsenite, respectively.82 Additionally, many other carbonaceous materials have been employed to adsorb As ions including iron oxide nanoparticle-coated SWCNTs,83 chitosan magnetic graphene oxide (CMGO),84 iron oxide–graphene oxide nanocomposite,85 and graphene oxide/copper ferrite foam (GCFF).83
Momčilović et al. used AC prepared from the cones of European black pine as an adsorbent for the removal of lead(II) ions, which showed the maximum adsorption capacity of 27.53 mg g−1.13 Another study investigated hybrid nanofibers based on ZnO-loaded activated carbon nanofibers (ZnO-ACNFs). These activated NFs exhibited an adsorption capacity of 92.59 mg of Pb(II)/g within a contact time of 45 min.86 Moreover, GO prepared from waste dry cell batteries showed an impressive performance for the removal of Pb(II) ions, achieving a 98.87% removal efficiency. The oxygenated groups present on the surface of GO were responsible for the adsorption of Pb ions.87 Similarly, diethylenetriamine-modified MCNTs(d-MWCNT),88 CNT modified with four generations of poly-amidoamine dendrimer (PAMAM/CNT),89 graphene oxide–MnFe2O4 magnetic nanohybrids (GO–MnFe2O4 nanohybrids)90 were reported as carbon-based adsorbents for the removal of Pb(II) ions from water. The above-mentioned literature survey confirmed that carbon-based materials are excellent sorbents for the removal of heavy metals from water bodies due to the presence of various functionalities in their network. These studies underscore the importance of optimizing the functionalization process to enhance the adsorption efficiency. Carbon-based materials and the different factors affecting their performance such as pH, adsorption capacity, applicable isotherms and kinetic models are shown in Table 2.
Table 2 Comparison of different carbon-based adsorbents for the removal of heavy metals
Adsorbate |
Adsorbent |
pH |
Maximum adsorption capacity (mg g−1) |
Applicable isotherm models |
Applicable kinetic models |
Ref. |
Hg(II) |
(SWCNT-SH) |
5 |
131 |
Langmuir |
A first-order rate model |
70 |
(CBC) |
4 |
38.08 |
Langmuir isotherm |
Pseudo-second-order kinetic |
71 |
Camel bone charcoal |
2 |
28.24 |
Langmuir |
|
72 |
Sheep bone charcoal |
2 |
12.550 |
Freundlich isotherm model |
|
73 |
Coconut shell-based granulated activated carbon |
4.5 |
15.19 |
Langmuir |
|
74 |
Cr(VI) |
AC/f-MWCNTs |
2.2 |
113.29 |
Langmuir equation |
|
76 |
GO |
|
240.3 |
|
|
77 |
GO-αcd-PPY NC |
|
666.67 |
Langmuir models |
|
78 |
Cd(II) |
Oxidized multiwalled carbon nanotubes |
8 |
13.5 |
Freundlich |
|
79 |
MWCNTs |
7 |
181.81 |
|
|
80 |
Graphene magnetic composite nanoparticles (Fe3O4-GS) |
6–7 |
163.6 |
Freundlich equation |
Pseudo-second-order model |
91 |
(Fe3O4 NPs/HPC) |
|
34.22 |
|
|
92 |
As(III) |
KM-CNTs |
3.0 |
23.40 |
Freundlich |
Pseudo-second-order kinetics |
81 |
As(V) and As(III) |
Microwave assisted MWCNTs |
6 |
92.0% and 91.0% removal efficiency |
Temkin, Dubinin-Radushkevich, Freundlich, and Langmuir models |
Pseudo-first order |
82 |
As(V) |
Iron oxide nanoparticle-coated SWCNT |
8 |
49.65 |
Freundlich model |
Pseudo-second order |
83 |
As(III) |
CMGO |
7.5 |
45 |
Langmuir isotherm |
Pseudo-second-order kinetic model |
84 |
As(III) and As(V) |
Iron oxide–graphene oxide nanocomposite |
|
147 for As(III) and 113 for As(V) |
|
|
85 |
As(III) and As(V) |
Graphene oxide/copper ferrite foam (GCFF) |
7 |
As(III) and As(V) are 51.64 and 124.69 |
Langmuir model |
|
83 |
Pb(II) |
Activated carbon obtained from cones |
6.7 |
27.53 |
Langmuir model |
Pseudo-second-order kinetic model |
13 |
ZnO-ACNFs |
pH 7 |
92.59 |
Langmuir model |
Pseudo-second-order kinetic model |
86 |
GO prepared from waste dry cell battery |
4 |
55.80 |
Langmuir model |
Pseudo-second-order kinetic model |
87 |
D-MWCNT |
6.2 |
58.26 |
Langmuir model |
Pseudo-second-order kinetic model |
88 |
PAMAM/CNT |
7 |
4870 |
Langmuir |
Pseudo-second-order kinetic model |
89 |
GO–MnFe2O4 nanohybrids |
|
673 |
Langmuir |
Pseudo-second-order kinetic model |
90 |
6. Agriculture waste-based adsorbents
Different parts of plants such as their bark, stem, leaves, root, flower, fruit biomass, husk, hull, skin, shell, bran and stone fall under the category of agriculture waste, as shown in Fig. 6.93 These types of adsorbents have the ability to remove organic and inorganic pollutants through different interaction modes owing to their various functional moieties. Adsorbents derived from agriculture waste exhibit abundant availability of binding groups, demonstrating a strong affinity and selective capability to attach heavy metals. These adsorbents contain structural polysaccharides and various functional groups such as carboxyl, phenolic, amido, amino, and sulphydryl carboxyl groups. These groups have the ability to effectively bind heavy metals either by substituting hydrogen with metal ions or by donating electron pairs to form complexes.94 Agriculture waste-based adsorbents can be used directly as well as modified with acids, such as hydrochloric and phosphoric, or with alkaline solutions, such as sodium hydroxide, and potassium hydroxide, or cross-linked with other materials. Due to the high selectivity, porosity, and surface area of activated carbon, biochar, and charcoal produced from agricultural solid residuals, they have become emerging adsorbents for the removal of heavy metals.95
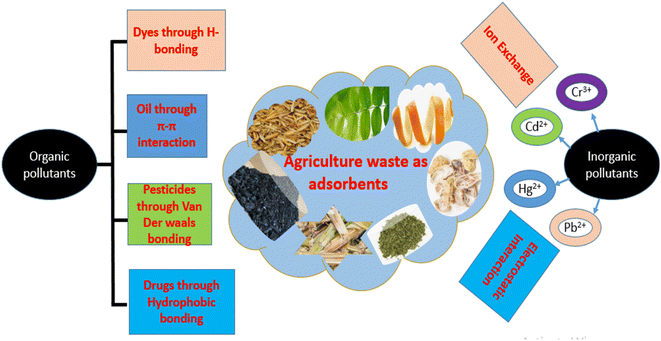 |
| Fig. 6 Agriculture waste-based adsorbents and their interaction with organic and inorganic pollutants.93 | |
Jeyaseelan et al. utilized green tea leaves (Camellia sinensis) as a natural adsorbent for the removal of Cr(VI) from water, achieving an impressive 99% removal efficiency under the optimized conditions including pH of 2, contact time of 180 min, and adsorbent dosage of 0.8 g L−1.96 Similarly, Eucalyptus bark (Eucalyptus globulus tree species) showed more than 99% removal efficiency at a concentration of 200 ppm. Furthermore, at a Cr(VI) concentration of 250 mg L−1 in the effluent, the adsorption capacity was reported to be 45 mg g−1 of adsorbent.97
In another study, Jatropha oil cake demonstrated a remarkable removal efficiency of 97% at pH 2. The adsorption capacity was reported to be 4.76 mg g−1 at an adsorbent dose of 2.5 g L−1. Additionally, FTIR analysis conducted before and after the adsorption showed a significant shift in the position and shape of the –OH group, indicating that Cr(VI) binding primarily occurs with –OH groups.98 Other agriculture waste bio-adsorbents include Caesalpinia bonduc plant leaves and their ashes,99 magnetic biochar derived from peanut hull,100 and leaves and stems of Eclipta prostrata,101 all of which have the ability to remove Cr(VI) ions. In the case of magnetic biochar derived from peanut hull, it was observed that there was no change in the crystal lattice of γ-Fe2O3 and no new shape crystals were formed. This observation clearly suggests that the maghemite layer attracts Cr(VI), leading to the formation of a monolayer.
Dubey et al. reported that Portulaca plant biomass (PPBM), obtained from its leaves and stems, has significant potential to remove Cd(II) ions from water, achieving 72% maximum adsorption efficiency at pH 6. The RL value of 0.429 indicates the favorable adsorption of Cd(II) ions.102 Similarly, another study revealed that the sawdust of Pinus sylvestris also has the capacity to bind Cd(II) ions. At pH 5.5, the maximum adsorption efficiency of 96% was observed. The adsorption process followed pseudo-second-order kinetics.103 Agriculture waste such as orange residues contains different functional components including cellulose, pectins, hemicellulose, chlorophyll pigments and other low-molecular weight compounds, including limonene. It has been observed that the carboxylic groups in pectins and the alcoholic hydroxyl groups in cellulose act as active binding sites for metals. The maximum adsorption capacity of orange waste was found to be 0.43 mmol g−1 at pH 6 for Cd(II) ions.104 Additionally, other adsorbents derived from agriculture waste including sugarcane bagasse (SCB), maize corncob (MCC) and Jatropha oil cake (JOC),105 bagasse fly ash,106 cashew nut shell,107 urea-modified wheat straw (MWS)108 show enhanced adsorption towards Cd(II) ions. In their investigation, Akbar and colleagues (Fig. 7) studied the use of natural flax fiber tows (FFT) as an adsorbent for removing Cu2+, Pb2+ and Zn2+ ions from water. The dominant mechanism for the adsorption of heavy metals in natural materials is the ion exchange mechanism. In flax fibers, which are lignocellulosic materials, the bound atoms (Na+ and Mg2+) are exchanged with the heavy metal ions present in wastewater and responsible for the good adsorption capacity of flax fibers tows. It was observed that the adsorption rate for these ions increased rapidly and the optimal removal efficiency was achieved within 60 min. The Langmuir model well-described the adsorption isotherm and a significant reduction in metal levels (97.4% for Pb, 79% for Cu and 73.28% for Zn) after FTT treatment was reported. The economic viability and potential for urban runoff water treatment make FTT a promising bio-based material.
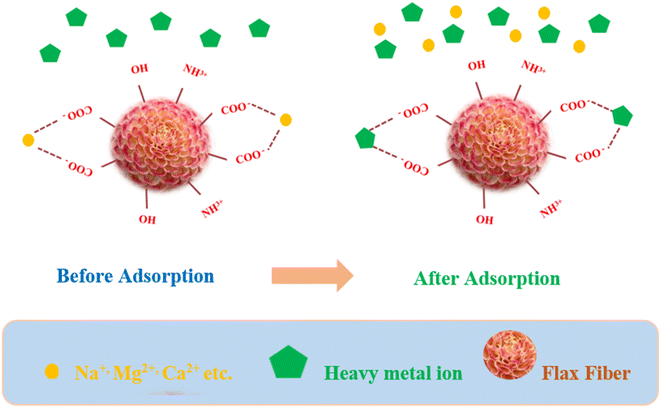 |
| Fig. 7 Schematic illustration of proposed mechanisms for heavy metal adsorption on flax fiber tows.109 | |
Recent studies highlighted the potential of raw and alkali metal ion-free banana peel as an effective adsorbent for the removal of Hg2+ ions, showing a maximum loading capacity of 46.8 and 52.2 mg g−1, respectively. This study emphasized that the protonation step enhances the removal efficiency of Hg2+ ions from aqueous solution.110 Giraldo et al. explored calcinated sugarcane bagasse (CSB) as an alternative adsorbent. CSB showed the maximum adsorption efficiency of 13.6 mg of Hg(II)/g. The kinetic studies suggested that the adsorption process follows chemisorption, confirming the strong interactions due to complexation through coordinated bonds.111 Moreover, magnetic carbon composites (MCC) prepared from pinewood sawdust showed an adsorption capacity of 167.22 mg g−1 for Hg2+ ions.112 Palm leaves,113 Eucalyptus leaves,114 and adsorbents derived from date pits115 serve as an effective sorbents for the removal of Hg(II) ions.
Rao et al. synthesized activated carbon prepared from Ceiba pentandra hulls and used them as a sorbent for the removal of lead, exhibiting a maximum removal efficiency of 99.5%.116 Another study focused on sunflower residue, which showed an adsorption capacity of 182 mg g−1 for Pb ions.117 Recent investigations have shown that banana stem scutcher shows a remarkable adsorption capacity of 179.9 mg g−1 for Pb(II) ions. FTIR and XPS analysis highlighted the significance of functional groups (C–OH, C–O–C) as major binding groups.118 Sugarcane bagasse,119 Musa paradisiaca peels,120 tea waste and peanut shells,121 maize cob,122 orange and banana peel123 are some other reported agriculture-based adsorbents for the removal of Pb(II) ions.
Javanshir and colleagues synthesized iron nanoparticles using a mixed extract of two plant species, namely Prangos ferulacea and Teucrium polium. These green nanoparticles showed an arsenic removal efficiency of 93.8% with a maximum adsorption of 61.7 mg g−1.124 In another study, magnetite nanoparticles were synthesized from onion peel (MNp-OP) and corn silk extract (MNp-CS), having a specific surface areas of 243 m2 g−1 and 261 m2 g−1, respectively, which demonstrated the ability to effectively remove As from water. The Langmuir isotherm model showed the maximum adsorption capacities of 1.86 mg g−1 for MNp-OP and 2.79 mg g−1 for MNp-CS.125 Other agriculture-based sorbents include iron nanoparticles synthesized from black tea leaves (Camellia sinensis), oak tree leaves (Quercus virginiana), green tea leaves (C. sinensis), pomegranate leaves (Punica granatum), and eucalyptus leaves (Eucalyptus globulus).126 Additionally, iron nanoparticles (BB-Fe NPs) produced using blueberries extract,127 modified green tea waste,128 and nanoparticles synthesized from mint leaves129 demonstrated the capacity for arsenic removal. Table 3 lists the various reported agriculture waste-based adsorbents together with their capacities for removing different metal ions and relevant parameters.
Table 3 Comparison of different agriculture waste-based adsorbents for the removal of heavy metals
Adsorbate |
Adsorbent |
pH |
Adsorption capacity (mg g−1) |
Isotherm |
Kinetics |
Ref. |
Cr(VI) |
Eucalyptus bark |
2 |
45 |
Freundlich isotherm |
First order |
97 |
Caesalpinia bonduc |
|
19.6 with ash |
|
|
99 |
12.7 with leaves |
Magnetic biochar derived from peanut hull |
|
77.54 |
Elovich model |
|
100 |
Jatropha curcas seed cake |
2.0 |
4.76 |
Freundlich isotherm |
|
98 |
Cd(II) |
Coffee grounds |
5 |
16.2 |
Langmuir and Freundlich models |
Pseudo-second-order |
130 |
Wheat straw |
5 |
31.6 |
Langmuir and Freundlich models |
Pseudo-second-order |
130 |
Cashew nut shell |
5 |
22.11 |
Freundlich |
Pseudo-second order |
107 |
Urea-modified wheat straw |
6 |
39.22 |
Langmuir model |
Pseudo-second-order kinetic model |
108 |
Orange peel |
7 |
35.71 |
Langmuir model |
Pseudo-second-order kinetic model |
131 |
Orange peel and Fe2O3 nanoparticles |
7 |
71.43 |
Langmuir model |
Pseudo-second-order kinetic model |
131 |
Cashew nut shell |
5 |
436.7 |
Langmuir isotherm |
Pseudo-second-order |
132 |
Reed plant (Phragmites australis) root |
10 |
168.6 |
|
|
133 |
Seaweed (Ascophyllum nodosum) |
10 |
106.3 |
|
|
133 |
Esterified spent grain from brewery |
5 |
473.93 |
Langmuir isotherm |
Pseudo-second order |
134 |
Raw spent grain from brewery |
5 |
335.57 |
Langmuir isotherm |
Pseudo-second order |
134 |
Hg(II) |
SDS-modified bamboo leaf powder |
8 |
31.05 |
Langmuir isotherm |
Pseudo-second-order |
135 |
SDS-modified bamboo leaf powder(BLP) |
8 |
27.1 |
Both Freundlich and Temkin isotherms |
Pseudo-second-order |
135 |
Triton X-100-modified BLP |
8 |
28.1 |
Both Freundlich and Temkin isotherms |
Pseudo-second-order and Elovich |
135 |
Alkali metal ion-free banana peel |
|
52.2 |
Langmuir |
Pseudo-second-order |
110 |
CSB |
6 |
13.6 |
Langmuir |
Pseudo-second-order and intraparticle diffusion |
111 |
Pinewood sawdust (MCC) |
|
167.22 |
Langmuir |
Pseudo-second-order |
112 |
Pb(II) |
Ceiba pentandra hull AC |
6.0 |
25.5 |
Freundlich isotherm |
Second-order rate |
116 |
Sunflower residue |
5 |
182 |
Modified two-site Langmuir |
|
117 |
Banana stem scutcher |
|
179.9 |
Langmuir model |
Pseudo-second-order kinetic |
118 |
Sugarcane bagasse |
6.0 |
1.61 mg g−1 with 89.31% removal efficiency |
Freundlich isotherm |
Pseudo-second-order kinetic models |
119 |
Maize cob |
5 |
95% removal efficiency |
Freundlich model |
Lagergren first-order kinetics |
122 |
As(III) |
Green-synthesised iron nanoparticles using Prangos ferulacea and Teucrium polium extract |
6 |
61.7 |
Redlich-Peterson isotherm |
Pseudo-second-order kinetic model |
124 |
(MNp-OP) and (MNp-CS) |
8.1 |
1.86 and 2.79 |
Freundlich model |
Pseudo-second-order model |
125 |
As(V) |
Eucalyptus leaf nanoparticles |
|
39.84 |
Langmuir model |
Pseudo-second kinetic model |
126 |
As(V) |
Oak tree leaf nanoparticles |
|
32.05 |
Langmuir model |
Pseudo-second kinetic model |
126 |
BB-Fe NPs |
|
50.40 |
Langmuir-Freundlich model |
Pseudo-second kinetic model |
127 |
7. Micro-organism-based adsorbents
Many microbial species such as bacteria, fungi, yeast and algae are known to be capable of adsorbing heavy metals on their surface. These microbial species have special surface properties such as adhesion and flocculation abilities. The number of effective adsorption binding sites increases with the modification of functional groups on the cell surface. Various functional groups such as carboxylic, hydroxyl, amines, and phenolic groups endow microorganisms with a negatively charged cell surface, enabling them to bind various cationic species such as heavy metals. Microbial cells have several protection mechanisms against heavy metal toxicity, such as active efflux of metal ions and reduction of metal ions. Ambient factors, sorbing materials, and metals to be removed are factors affecting the biosorption efficiency of microorganism-based adsorbents.136,137
Umer Shafique and team utilized Pleurotus ostreatus as a bioadsorbent for the removal of chromium(VI) owing to its fast metal removal rate, remarkable biosorption capacity and high regeneration ability. The maximum adsorption of Cr(VI) takes place at pH 2.5, while the maximum bio-sorption capacity of fungus was 10.75 mg g−1. FTIR analysis revealed that amine and carboxylic acid groups play a major role in the adsorption process.138 In another study, Staphylococcus saprophyticus bacteria were found to be proficient for the removal of chromium, showing the maximum adsorption of 88.66 mg for Cr(VI) at pH 2, temperature of 27 °C, and an initial ion concentration 193.66 mg L−1.139 Dadrasnia et al. used Bacillus salmalaya as a biosorbent, showing a maximum sorption capacity of 20.35 mg g−1 for Cr(VI). The adsorption process followed a pseudo-second-order mechanism and the Langmuir isotherm model provided the best fit to the data. The thermodynamic parameters showed that it is an endothermic process.140 Similarly, Chlorella vulgaris was used as an adsorbent for the removal of chromium(VI) ions, showing a removal efficiency of 99.75% under the optimum conditions. The value of RL confirmed the favorable adsorption process.141 Moreover, Spirulina platensis possesses enriched functional groups, which aid in the adsorption of metal ions. Sulfate, phosphate, hydroxyl, carbonyl and other charged groups present in algae contribute to heavy metal binding. Waste algal biomass of Spirulina platensis, obtained after biodiesel production, was successfully employed as an adsorbent for the removal of chromium(VI) ions with an adsorption capacity of 45.5 mg g−1. This approach demonstrated the potential for reusing algal biomass waste as an adsorbent.142
Mahmood et al. reported the adsorption potential of dead biomass of Sargassum sp., a brown marine alga, for the removal of heavy metals, specifically Cd(II). Remarkably the removal efficiency reached 95.3%.143 Additionally, Bacillus subtilis coated with maghemite nanoparticles was used as a biosorbent for the removal of Cd(II) ions. It showed a maximum removal efficiency of 84% and a high recovery percentage (76.4%), making it a sustainable method. The adsorption data correlated well with the Langmuir and Freundlich isotherm models and the pseudo-second-order equation provided a good correlation with the obtained adsorption data.144
Three different mechanisms are observed for the removal of heavy metals by algae.145 The most common mechanism is the biosorption of heavy metals. The cell wall of algae contains different functional groups such as carboxyl, hydroxyl, sulfate and amino groups, which are responsible for ion exchange, electrostatic interaction and complexation of heavy metals. Cyanobacterial extracellular polymers contain a variety of polymers such as nucleic acid, polysaccharides, and proteins, which play a vital role in biosorption. Polysaccharides help to bind heavy metals to the surfaces, lipids, and proteins of algae. The transport of metal ions across the cell wall is another mechanism for adsorption on algae, which is known as bioaccumulation. Energy is required to accumulate intracellular heavy metals. In this process, as microorganisms grow, they accumulate heavy metals intracellularly and allow metals to concentrate, often exceeding the levels in the surrounding environment. Another type of mechanism used for the detoxification of heavy metals in the cells of algae is biotransformation, which is related to the enzymatic or biochemical transformation of heavy metals. In the case of enzymatic transformation, the enzymes present inside the cells of algae convert the non-degradable heavy metals into less hazardous inorganic complexes. A schematic representation of these mechanisms is shown in Scheme 1.
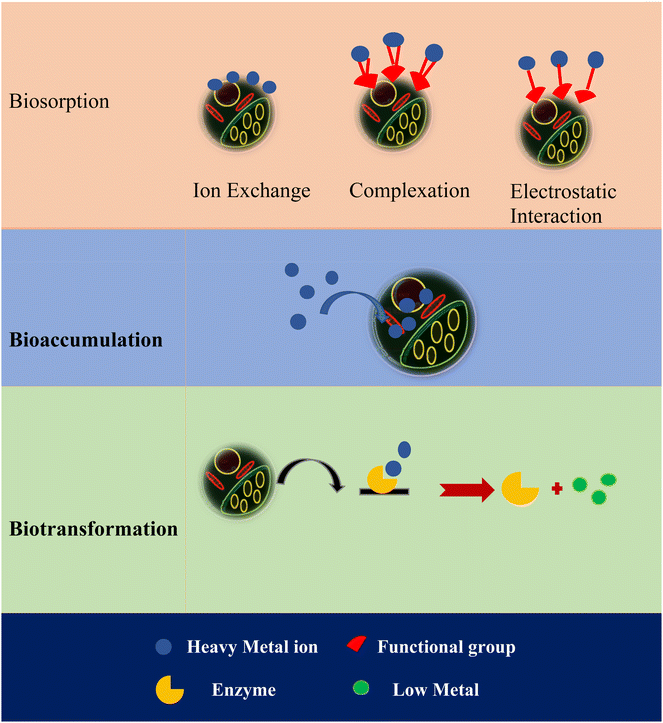 |
| Scheme 1 Mechanism for the removal of heavy metals by algae.34 | |
In a separate study, Pseudomonas aeruginosa B237 bacteria exhibited a maximum Cd2+ adsorption capacity (qmax) of 16.48 mg g−1. The Langmuir isotherm model effectively described the Cd2+ ion adsorption by this bacterial biomass with its favorable process indicated by the low RL value of 0.002.146 Aspergillus niger (A. niger)147 and Spirulina platensis also have the capability to serve as sorbents for the removal of Cd(II) ions. Wen et al. synthesized a novel adsorbent by immobilizing the endogenous bacterium Bacillus licheniformis with magnetic polyvinyl alcohol (PVA) and sodium alginate for the removal of lead ions, showing a maximum adsorption capacity of up to 113.84 mg g−1.148 Furthermore, the fungus Mucor indicus was found to have the ability to remove lead with a maximum biosorption capacity of 22.1 mg g−1.149 Similarly, the red marine algae Jania rubens pretreated with formaldehyde showed the highest adsorption capacity of 774 mg g−1 for Pb(II) ions and that for Jania pretreated with CaCl2 reached 1089.6 mg g−1.150 Other microorganism-based biosorbents including Aspergillus niger and Aspergillus terreus fungus isolates on luffa sponge,151 Cystoseira compressa, Sargassum vulgare, Turbinaria, Agaricus campestris,152 as well as the green alga Ulva lactuca153 have been used for removal of lead ions.
Ahmet Sarı and coworkers investigated the efficiency of dead green algae (Mougeotia genuflexa) biomass for the removal of As(III) from water. The Langmuir model showed a maximum monolayer biosorption capacity of 57.48 mg g−1. Additionally, the mean free energy calculated from the D–R model (10.2 kJ mol−1) indicated that the adsorption occurred through chemical ion exchange.154 A mixture of green (Chlorophyta) and blue-green (Cyanobacteria) algae showed the maximum sorption capacity of 35 mg g−1 for As(III). FTIR confirmed the presence of hydroxyl and carboxyl groups on the surface of the adsorbent, thus playing a key role in capturing metals.155 Similarly, some bacterial stains such as Yersinia sp. strain SOM-12D3 showed the adsorption capacity of 159 mg g−1 towards As(III).156 Other microbial adsorbents including Bacillus thuringiensis strain WS3,157 mixed dried biomass of Bacillus thuringiensis strain, Pseudomonas stutzeri strain and Micrococcus yunnanensis strain,158 and Saccharomyces cerevisiae61 exhibited enhanced ability to remediate As(III) ions.
Xia et al. reported that extracellular polymeric substances (EPS) extracted from Klebsiella sp. NT8 and Bacillus sp. NT10 (EPS-K and EPS-B) have the maximum sorption capacity of 2597.62 and 2617.23 mg g−1, respectively, for Hg(II) ions. The adsorption followed pseudo-second-order kinetics and fit the Langmuir isotherm model.159 Another study showed that Brevundimonas species IITISM22 has the potential to remove Hg ions, achieving a maximum adsorption capacity of 666.6 mg g−1 at pH 6.5.160 Additionally, yeast Yarrowia spp.,161 Sargassum glaucescens (brown algae),162 Sargassum bevanom,163 Chlorella vulgaris,164 etc. show promise as effective sorbents for Hg(II) ions. Table 4 provides a comparison of various microorganism-based adsorbents used for the removal of heavy metal ions together with their important parameters.
Table 4 Comparison of different microorganism-based adsorbents for heavy metals
Adsorbate |
Type |
Adsorbent |
pH |
Adsorption capacity (mg g−1) |
Ref. |
Cr(VI) |
Fungi |
Pleurotus ostreatus |
|
10.75 |
138 |
Bacteria |
Bacillus salmalaya |
|
20.35 |
140 |
Chemically modified algae/waste algal biomass |
Biodiesel-extracted Spirulina platensis |
|
45.5 |
142 |
Rhizoclonium hookeri (0.1 M HCl) |
|
67.3 |
142 |
Sargassum siliquosum |
|
66.4 |
142 |
Sargassum sp. |
|
58.2 |
142 |
Bacteria |
Bacillus megaterium |
|
30.7 |
165 |
Yeast |
Yeast |
|
86.95 |
166 |
Cd(II) |
Bacteria |
Bacillus subtilis coated with maghemite nanoparticles |
4 |
71.4 |
144 |
|
Pseudomonas aeruginosa |
6 |
16.48 |
146 |
Fungi |
Aspergillus niger (A. niger) |
4 |
15.50 |
147 |
Algae-based bioadsorbents |
Red algae Galaxaura oblongata |
5 |
85.5 |
167 |
|
Red algae Chondracanthus chamissoi |
4 |
85.4 |
168 |
|
Red algae Hypnea valentiae |
6 |
28.6 |
169 |
|
Alginate-PEI modified with Fucus vesiculosus (brown algae) |
3.5 |
97.8 |
170 |
Pb(II) |
Bacterium |
Bacillus licheniformis-based adsorbent |
6 |
113.8 |
148 |
Fungus |
Mucor indicus |
5.5 |
22.1 |
149 |
Algae |
Jania rubens pretreated with formaldehyde |
|
774 |
150 |
Algae |
Jania pretreated with CaCl2 |
|
1089.6 |
150 |
As(III) |
Algae |
Mougeotia genuflexa |
6 |
57.48 |
154 |
Algae |
Mixture of green (Chlorophyta) and blue-green (Cyanobacteria) algae |
4–5 |
35 |
155 |
Bacteria |
Yersinia sp. strain SOM-12D3 |
7 |
159 |
156 |
Bacteria |
Bacillus thuringiensis strain WS3 |
7 |
95.238 |
157 |
Fungus |
Saccharomyces cerevisiae |
|
113.9 |
61 |
Hg(II) |
|
EPS-K and EPS-B |
|
2597.62 and 2617.23 |
159 |
Bacteria |
Brevundimonas species IITISM22 |
6.5 |
666.6 |
160 |
Yeast |
Yarrowia spp. |
|
32.2 |
161 |
Algae |
Sargassum glaucescens |
5,7 |
147.05 |
162 |
Algae |
Chlorella vulgaris |
6 |
42.0 |
164 |
8. Inorganic-based adsorbents
Inorganic-based adsorbents are ideal and effective adsorbents for the removal of heavy metal ions, which include clay, soil, minerals and zeolites. Their high affinity for heavy metal ions stems from their properties such as solubility (Ksp), charge density, electronegativity, and hydrolysis constant (pKH). Minerals and soil readily precipitate metal carbonates and hydroxides due to these properties.171 Ahmadi et al. investigated bentonite clay (BC) and bentonite clay@MnFe2O4 composite (BCMFC) for the removal of Cr(III) and Cr(VI). The adsorption followed the Freundlich isotherm model and quasi-second-order kinetic model and demonstrated physical, spontaneous, and exothermic nature of adsorption.172 Similarly, red mud,173 natural clay and clay/Fe–Mn composite,174 and iron oxide-coated acid-treated activated red mud175 have been proven to be effective for the removal of heavy metals ions.
Natural and modified zeolites also contribute to the remediation of heavy metals. Their ion exchange properties and molecular sieve-type structures make them suitable candidates for the removal of heavy metals. Further, they offer advantages such as selectivity, low sludge production and compliance with strict discharge standards. Modification of natural zeolites enhances their surface properties.176 Thus, Neolaka et al. reported the use of an activated natural zeolite-magnetic composite (ANZ–Fe3O4) as an adsorbent material with a Cr(VI) adsorption capacity of 2.850 mg g−1.177 Recent studies showed that a zeolite-Ag2S nanohybrid material exhibited an extraordinary adsorption capacity of 390 mg g−1 towards Hg(II) ions in wastewater with higher removal rates than pure zeolite.178 It was found that the zeolite component of the nanohybrid material provided a porous structure with high surface area, while the Ag2S NPs enhanced its adsorption capacity, as shown in Fig. 8. Thus, Ag2S interacted with Hg(II) ions, forming stable complexes via –OH− and –COO− groups on the nanohybrid surface. The sulphur atom can act as a ligand and attach the Hg(II) ion. Electrostatic interactions are responsible for the stabilization of metal ions on the surface of nanohybrids. Zeolites are aluminosilicates and the interaction of Hg(II) with the oxygen atom present in zeolites or with the sulphur atom present in metal sulfides represents physisorption, as shown in pathway 1 and 2. In pathway 3, the hydrogen atom from one hydroxyl group is removed and metal ions form a bond between two hydroxyl atoms, which represent chemisorption.
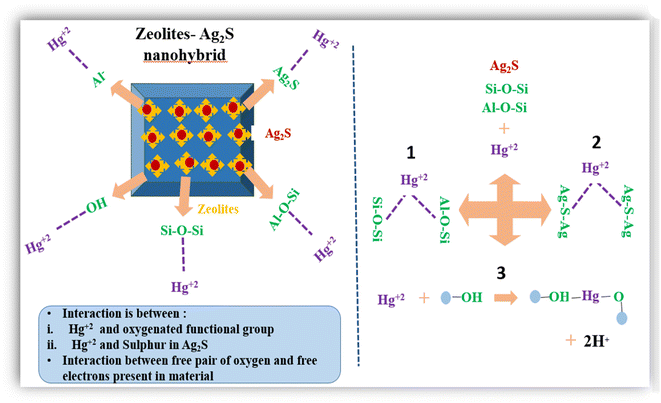 |
| Fig. 8 Major processes of mercury adsorption in zeolite-Ag2S nanohybrid materials from wastewater. The proposed chemical reaction mechanism: (1) and (2) represent physisorption and (3) represents chemisorption. Reproduced with permission from ref. 178. Copyright [2023], Elsevier. All rights are reserved. | |
Due to chemisorption, strong chemical bonds are formed between Hg(II) ions and the functional groups on the nanohybrid surface. The simple synthesis, high effectiveness and stability of functionalized nanohybrids make them excellent candidates for wastewater remediation.
Similarly, an Na–Y zeolite demonstrated the maximum adsorption capacity of 0.81 mmol g−1 for Cd(II) ions. The adsorption process is favorable, as indicated by the separation factor. Physical sorption mechanisms were responsible for the process, as supported by the mean free energy value of less than 8 kJ mol−1.179 Other zeolite-based adsorbents including zeolites/MgAl-LDHs,180 magnetic zeolite,181 and dithizone-immobilized natural zeolite (DIZ)182 have been employed for the removal of heavy metals via the adsorption process.
Additionally, silica-based materials such as mesoporous silica as adsorbents offer advantages due to their porosities, large surface areas, and reasonable mechanical thermal stabilities.183 Snoussi and coworkers employed polyethylenimine-functionalized mesocellular silica foam as an adsorbent, showing more than 90% removal efficiency towards Cd(II) ions.184 Another study showed that a silica gel material modified with nitrilotriacetic acid (NTA-silica gel) exhibited the maximum adsorption capacity of 76.22 mg g−1 for Pb(II) ions. Equilibrium and kinetic studies confirmed that the adsorption followed the Freundlich and Langmuir isotherm models together with a pseudo-second-order kinetic model. The positive ΔH° values and negative ΔG° values show that the adsorption of lead is an endothermic and spontaneous process, respectively.185 Bao et al. reported the use of mercaptoamine-functionalised silica-coated magnetic nanoparticles (MAF-SCMNPs) as an adsorbent for the removal of Pb(II) ions. The mechanism involved chelation through the amine group and ion exchange between heavy metal ions and the thiol functional groups on the nanoadsorbent surface. The maximum adsorption capacity of 292 mg g−1 for Pb(II) ion showed that silica-based adsorbents can play a role in removing inorganic pollutants such as heavy metals from aqueous medium.186
Fig. 9 (ref. 187) shows the mechanism of adsorption and desorption of Pb(II) on mesoporous silica. The understanding of adsorption and regeneration is vital for the synthesis of new adsorbents in the future. Electrostatic interaction is responsible for the adsorption of positively charged lead ions and negatively charged silica. The pHzpc of mesoporous silica is 3.2, above which, it is negatively charged and the concentration of H+ decreases. The maximum adsorption of Pb(II) takes place at pH 6 through electrostatic interactions. When the pH of the solution is lower than the pHzpc value, the process of desorption from lead-loaded mesoporous silica is dominant.
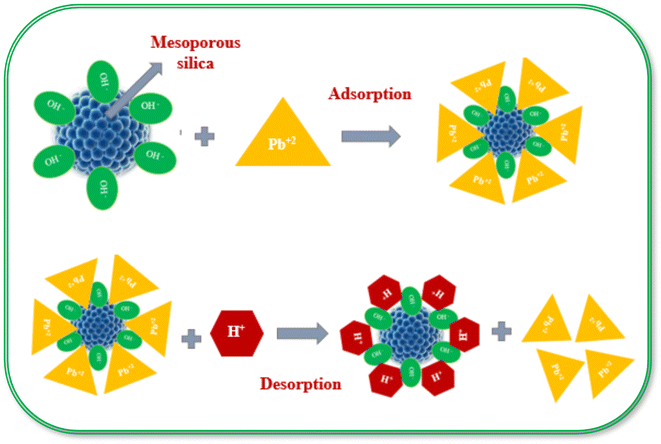 |
| Fig. 9 Mechanism of the adsorption and regeneration of Pb(II) on mesoporous silica. Reprinted with permission from ref. 187. Copyright [2015], Elsevier. All rights are reserved. | |
The other reported silica-based adsorbents include mesoporous silica modified by iron-manganese binary oxide (FeMnOx/SBA-15) with 76.5% FeMnOx mass fraction,188 silica-based hybrid organic–inorganic adsorbent (MNPs@SiO2-TSD-TEOS),189 diatom silica microparticles functionalized with n-(2-aminoethyl)-3-aminopropyl-trimethoxysilane (AEAPTMS),190 and functionalized mesoporous silica/poly(m-aminothiophenol) nanocomposite191 for the removal of heavy metal ions. Table 5 summarizes the different inorganic-based adsorbents for the removal of heavy metals, together with their important adsorption parameters.
Table 5 Comparison of various inorganic-based adsorbents for the removal of heavy metals
Adsorbate |
Adsorbent |
pH |
Adsorption capacity |
Ref. |
Cr(VI) |
Bentonite clay (BC) |
3 |
95.74% |
172 |
161.3 mg g−1 |
Bentonite clay@MnFe2O4 composite (BCMFC) |
3 |
98.65% |
172 |
178.6 mg g−1 |
Activated natural zeolite-magnetic composite (ANZ-Fe3O4) |
2 |
2.850 mg g−1 |
177 |
Zeolites/MgAl-LDHs |
|
121.85 mg kg−1 |
180 |
Zeolites/ZnAl-LDHs |
|
272.10 mg kg−1 |
180 |
Cd(II) |
MgO-ATP |
5 |
25.3 mg g−1 |
192 |
Magnetic zeolite |
|
204.2 mg g−1 |
181 |
Na–Y zeolite |
5–6 |
0.81 mmol g−1 |
179 |
Polyethylenimine-functionalized mesocellular silica foam |
5 |
90% |
184 |
As(III) and As(V) |
Mesoporous silica modified by iron-manganese binary oxide (FeMnOx/SBA-15) with 76.5% FeMnOx mass fractions |
Below 9 |
90% of As 32.89 mg g−1 As(III) |
188 |
35.71 mg g−1 As(V) |
Red mud |
7.25 for As(V) |
96.52% for As(V) |
173 |
Natural clay and clay/Fe–Mn composite |
3–4 |
86.86 mg g−1 and 120.70 mg g−1 for As(V) |
174 |
Zeolitic imidazolate framework-8 (ZIF-8) |
7 |
As(III) and As(V) were 49.49 and 60.03 mg g−1, respectively |
185 |
Pb(II) |
Iron oxide-coated acid-treated activated red mud |
6 |
27.02 mg g−1 |
175 |
Mercaptoamine-functionalised silica-coated magnetic nanoparticles |
6–7 |
292 mg g−1 |
186 |
NTA-silica gel |
|
76.22 mg g−1 |
185 |
(MNPs@SiO2-TSD-TEOS) |
5 |
417 mg g−1 |
189 |
Hg(II) |
Diatom silica microparticles functionalized with n-(2-aminoethyl)-3-aminopropyl-trimethoxysilane (AEAPTMS) |
|
169.5 mg g−1 |
190 |
Functionalized mesoporous silica/poly(m-aminothiophenol) nanocomposite |
|
242.42 mg g−1 |
191 |
Dithizone-immobilized natural zeolite (DIZ) |
5 |
13.1 μmol g−1 |
182 |
Zeolite-Ag2S nanohybrid |
|
390 mg g−1 |
178 |
9. Advantages and disadvantages of different adsorbents
• The possibility of regeneration and recovery of metal ions are major benefits associated with biological adsorbents. These adsorbents are eco-friendly, easily accessible, and depending on the biomass source and pretreatment techniques, their adsorption capacities may vary, impacting their selectivity. Mechanical instability and challenges in separating biomass after the removal process are the major disadvantages associated with using microorganisms. Thus, to eliminate these disadvantages, the method of immobilization of microorganisms on a carrier is used which, increases the productivity, improved mechanical strength, and increased chemical resistance.
• Nanomaterial-based adsorbents have a high surface area and enhanced reactivity. However, modified carbonaceous materials face challenges related to complexity, their modification and the need to balance their high adsorption capacity with other desirable properties. Difficulty in the recovery of nanoparticles after adsorption is another limitation associated with nanomaterials. However, magnetic nanoparticles allow easy separation when a magnetic field is applied. Biopolymers such as chitosan are valuable for wastewater treatment due to their hydroxyl and amino groups, facilitating the adsorption of contaminants from wastewater. However, they suffer from drawbacks such as instability, low selectivity, solubility in acidic media, poor mechanical properties and challenges in controlling their pore size. Thus, to address these limitations and capitalize on the strengths of both nanomaterials and biopolymers, bio-nanocomposites have emerged. Examples include cyclodextrin-polycaprolactone/TiO2-NPs, chitosan/clay, and cellulose nanocrystal/ZnO-NPs, which offer combined properties for effective wastewater treatment.
• Agriculture-based adsorbents such as vegetable and fruit peels are easily available, cost effective, and have abundant functional groups and high metal adsorption capacity, which make them popular adsorbents nowadays. Moreover, they are easily processed, applied, and recovered without causing any harmful effect to the environment. Chemical modification of agricultural waste-based adsorbents improves their adsorption capacity by exposing functional groups. This process involves removing lower molecular weight lignin, waxes and natural fats from the adsorbent, while also creating surface roughness. However, it is important to note that modifying agriculture-based adsorbents can sometimes lead to environmental toxicity. Also, it is worth noting that employing green technology for the treatment of wastewater must not compromise agricultural production, which can potentially exacerbate the food shortage. Thus, the adsorbent from green resources should be carefully selected to ensure they have no value as food sources.
• The abundant clays and clay-based composites have natural physicochemical properties, high specific surface area, extraordinary cation exchange capacity (CEC), surface hydrophilicity, surface electronegativity and cation exchange selectivity, due to which they have gained attention for the remediation of pollutants from water bodies. However, despite the easy availability, low cost and high surface area of clay-based adsorbents, the regeneration of these adsorbents through desorption techniques is not feasible. One more disadvantages is controlling the pH of clay.34,58,193,194
• MOFs have a high surface area, highly ordered pore size and shape, which can be tuned by changing the linkage and the type of linkers used. However, despite their remarkable efficacy and selectivity in adsorbing heavy metal ions, many MOFs suffer from poor water and chemical stability.61
• Naturally available inexpensive resources for biopolymers have multiple active sites for adsorption but the efficiency of the adsorption process is hindered by their low mechanical strength, low surface areas, and poor stability.57 Also, biopolymers may undergo degradation under harsh treatment conditions, compromising their effectiveness. Although biopolymers offer sustainability benefits, their drawbacks must be addressed to ensure efficient contaminant removal in wastewater treatment.
10. Conclusion, future prospective and challenges
Among the variety of wastewater treatment methods, the adsorption process is the preferred choice owing to the adsorption potential of different adsorbents for the removal of heavy metals. Among the various carbon-based adsorbents, CNTs modified with four generations of poly-amidoamine dendrimer demonstrated the highest adsorption capacity (4870 mg g−1) towards Pb2+ ions. It was found that adsorbents based on micro-organisms such as extracellular polymeric substances (EPS) extracted from Klebsiella sp. NT8 and Bacillus sp. NT10 (EPS-K and EPS-B) exhibit the maximum sorption capacity of 2597.62 and 2617.23 mg g−1 for Hg(II) ions, respectively. This review concludes that efficient adsorption depends strongly on the interaction between the adsorbate and the adsorbent, which is mostly influenced by various factors such as pH, initial adsorbate concentration, adsorbent mass and temperature. The majority of the adsorption process data best fit the pseudo-second-order kinetic model, which follows either the Langmuir or Freundlich isotherms. By utilizing low-cost adsorbents such as waste materials and agriculture residues, the environmental hazards associated with heavy metal ion removal can be addressed to meet the WHO standards for drinking water quality. Waste materials can serve as an alternative to replace activated carbon for application in water purification due to their easy availability and low cost. It is expected that low-cost adsorbents will become important for the removal of heavy water from wastewater in the near future. Overall, this review offers guidelines to new researchers on how to develop useful adsorbents with a better adsorption capacity for heavy metal ions in wastewater.
Due to the escalating water contamination crisis, a range of adsorbents is under extensive investigation for treating metal-contaminated waters owing to their extraordinary properties, as outlined in this review. However, despite the advancements, further studies are necessary to optimize the performance of adsorbents. In the future, potential directions for improving adsorbent development and utilizing the adsorption process for industrial applications include:
• A comprehensive investigation into the mechanisms behind the selective and specific adsorption of metal ions is imperative. This endeavor will facilitate the design of targeted removal strategies for the treatment of wastewater, thereby enhancing the efficiency in addressing water contamination issues.
• Although researchers primarily emphasize the exceptional adsorption capacity of various adsorbents, they often overlook their potential environmental impacts. Thus, to address this oversight, it is recommended that the biodegradability of these materials, together with the conditions for their degradation and proper disposal post-use be thoroughly investigated. This holistic approach will contribute to the development of environmentally sustainable adsorption technologies.
• Current studies show that modified adsorbents are more effective compared to unmodified adsorbents. However, the high cost of modification and the use of toxic additives impose limitations on their widespread usage. Therefore, future studies should prioritize the development of alternative modification methods that are both cost effective and environmentally benign.
• The complexity of the adsorption process compounded by the presence of mixed pollutants poses a significant challenge to practical applications. Currently, research efforts focus on single pollutants, thereby limiting the applicability of findings. However, industries are now actively pursuing low-cost adsorbents capable of simultaneously removing multiple coexisting pollutants. This area of research is currently highly active and aims to address the pressing need for comprehensive pollution mitigation solutions.
Conflicts of interest
Authors declare no conflict of interest.
Acknowledgements
Afzal Shah acknowledges the support of Quaid-i-Azam University and the Higher Education Commission of Pakistan.
References
- N. Rascio and F. Navari-Izzo, Heavy metal hyperaccumulating plants: how and why do they do it? And what makes them so interesting?, Plant Sci., 2011, 180, 169–181 CrossRef CAS PubMed.
- M. Zaynab, R. Al-Yahyai, A. Ameen, Y. Sharif, L. Ali, M. Fatima, K. A. Khan and S. Li, Health and environmental effects of heavy metals, J. King Saud Univ., Sci., 2022, 34, 101653 CrossRef.
- R. Singh, N. Gautam, A. Mishra and R. Gupta, Heavy metals and living systems: an overview, Indian J. Pharmacol., 2011, 43, 246 CrossRef CAS PubMed.
- H. A. Hegazi, Removal of heavy metals from wastewater using agricultural and industrial wastes as adsorbents, HBRC J., 2013, 9, 276–282 CrossRef.
- Y. Zhang, K. Yan, F. Ji and L. Zhang, Enhanced removal of toxic heavy metals using swarming biohybrid adsorbents, Adv. Funct. Mater., 2018, 28, 1806340 CrossRef.
- M. Barakat, New trends in removing heavy metals from industrial wastewater, Arabian J. Chem., 2011, 4, 361–377 CrossRef CAS.
- H. N. M. E. Mahmud, A. O. Huq and R. binti Yahya, The removal of heavy metal ions from wastewater/aqueous solution using polypyrrole-based adsorbents: a review, RSC Adv., 2016, 6, 14778–14791 RSC.
- Z. Khan, A. Elahi, D. A. Bukhari and A. Rehman, Cadmium sources, toxicity, resistance and removal by microorganisms-A potential strategy for cadmium eradication, J. Saudi Chem. Soc., 2022, 26, 101569 CrossRef CAS.
- K. G. Pavithra, P. SundarRajan, P. S. Kumar and G. Rangasamy, Mercury sources, contaminations, mercury cycle, detection and treatment techniques: a review, Chemosphere, 2022, 312, 137314 CrossRef PubMed.
- Q. Wang, D. Kim, D. D. Dionysiou, G. A. Sorial and D. Timberlake, Sources and remediation for mercury contamination in aquatic systems—a literature review, Environ. Pollut., 2004, 131, 323–336 CrossRef CAS PubMed.
- J.-Y. Chung, S.-D. Yu and Y.-S. Hong, Environmental source of arsenic exposure, J. Prev. Med. Public Health, 2014, 47, 253 CrossRef PubMed.
- A. Zhitkovich, Chromium in drinking water: sources, metabolism, and cancer risks, Chem. Res. Toxicol., 2011, 24, 1617–1629 Search PubMed.
- M. Momčilović, M. Purenović, A. Bojić, A. Zarubica and M. Ranđelović, Removal of lead (II) ions from aqueous solutions by adsorption onto pine cone activated carbon, Desalination, 2011, 276, 53–59 CrossRef.
- J. Goel, K. Kadirvelu, C. Rajagopal and V. K. Garg, Removal of lead (II) by adsorption using treated granular activated carbon: batch and column studies, J. Hazard. Mater., 2005, 125, 211–220 CrossRef CAS PubMed.
- S. Mitra, A. J. Chakraborty, A. M. Tareq, T. B. Emran, F. Nainu, A. Khusro, A. M. Idris, M. U. Khandaker, H. Osman and F. A. Alhumaydhi, Impact of heavy metals on the environment and human health: novel therapeutic insights to counter the toxicity, J. King Saud Univ., Sci., 2022, 34, 101865 CrossRef.
- K. Rehman, F. Fatima, I. Waheed and M. S. H. Akash, Prevalence of exposure of heavy metals and their impact on health consequences, J. Cell. Biochem., 2018, 119, 157–184 CrossRef CAS PubMed.
- M. Jaishankar, T. Tseten, N. Anbalagan, B. B. Mathew and K. N. Beeregowda, Toxicity, mechanism and health effects of some heavy metals, Interdiscip. Toxicol., 2014, 7, 60 CrossRef PubMed.
- F. U. Haider, C. Liqun, J. A. Coulter, S. A. Cheema, J. Wu, R. Zhang, M. Wenjun and M. Farooq, Cadmium toxicity in plants: impacts and remediation strategies, Ecotoxicol. Environ. Saf., 2021, 211, 111887 CrossRef CAS PubMed.
- F. U. Haider, C. Liqun, J. A. Coulter, S. A. Cheema, J. Wu, R. Zhang, M. Wenjun and M. Farooq, Cadmium toxicity in plants: impacts and remediation strategies, Ecotoxicol. Environ. Saf., 2021, 211, 111887 CrossRef CAS PubMed.
- J. Chen and Z. M. Yang, Mercury toxicity, molecular response and tolerance in higher plants, BioMetals, 2012, 25, 847–857 CrossRef CAS PubMed.
- M. Argos, H. Ahsan and J. H. Graziano, Arsenic and human health: epidemiologic progress and public health implications, Rev. Environ. Health, 2012, 27, 191–195 CAS.
- N. Singh, D. Kumar and A. P. Sahu, Arsenic in the environment: effects on human health and possible prevention, J. Environ. Biol., 2007, 28, 359 CAS.
- A. Monga, A. B. Fulke and D. Dasgupta, Recent developments in essentiality of trivalent chromium and toxicity of hexavalent chromium: implications on human health and remediation strategies, J. Hazard. Mater. Adv., 2022, 7, 100113 CrossRef CAS.
- J. Bayuo, K. B. Pelig-Ba and M. A. Abukari, Optimization of adsorption parameters for effective removal of lead (II) from aqueous solution, Phys. Chem.: Indian J., 2019, 14, 1–25 CrossRef PubMed.
- J. O. Duruibe, M. O. C. Ogwuegbu and J. N. Egwurugwu, Heavy metal pollution and human biotoxic effects, Int. J. Phys. Sci., 2007, 2, 112–118 Search PubMed.
- G. A. Engwa, P. U. Ferdinand, F. N. Nwalo and M. N. Unachukwu, Mechanism and health effects of heavy metal toxicity in humans, Poisoning in the Modern World-New Tricks for An Old Dog, 2019, vol. 10, pp. 70–90 Search PubMed.
- A. Haleem, A. Shafiq, S.-Q. Chen and M. A. Nazar, Comprehensive Review on Adsorption, Photocatalytic and Chemical Degradation of Dyes and Nitro-Compounds over Different Kinds of Porous and Composite Materials, Molecules, 2023, 28, 1081 CrossRef CAS PubMed.
- A. Haleem, F. Wu, M. Ullah, T. Saeed, H. Li and J. Pan, Fast and effective palladium adsorption from electronic waste using a highly macroporous monolith synthesized via rapid UV-irradiation, Sep. Purif. Technol., 2024, 331, 125500 CrossRef CAS.
- F. Wu, H. Li, J. Tang, A. Haleem and J. Pan, Surface Wettability Guiding In-situ Cultivation Engineering of Hollow Polymer Nanospheres for Persistent Efficient Uranium Extraction, J. Mater. Chem. A, 2023, 11, 26661–26671 RSC.
- A. Verma, K. Rejendra, K. Singh and S. Shukla, Use of low cost adsorbents for the remediation of heavy metals from waste water, IJLTEMAS, 2017, 6, 2278–2540 Search PubMed.
- Renu, M. Agarwal and K. Singh, Methodologies for removal of heavy metal ions from wastewater: an overview, Interdiscip. Environ. Rev., 2017, 18, 124–142 CrossRef.
- P. S. Kumar and R. Gayathri, Adsorption of Pb2+ ions from aqueous solutions onto bael tree leaf powder: isotherms, kinetics and thermodynamics study, J. Eng. Sci. Technol., 2009, 4, 381–399 Search PubMed.
- A. Gupta, V. Sharma, K. Sharma, V. Kumar, S. Choudhary, P. Mankotia, B. Kumar, H. Mishra, A. Moulick and A. Ekielski, A review of adsorbents for heavy metal decontamination: growing approach to wastewater treatment, Materials, 2021, 14, 4702 CrossRef CAS PubMed.
- Z. Raji, A. Karim, A. Karam and S. Khalloufi, Adsorption of heavy metals: mechanisms, kinetics, and applications of various adsorbents in wastewater remediation—a review, in Proceedings of the Waste, 2023, pp. 775–805 Search PubMed.
- A. Stafiej and K. Pyrzynska, Adsorption of heavy metal ions with carbon nanotubes, Sep. Purif. Technol., 2007, 58, 49–52 CrossRef CAS.
- A. Rahmani, H. Z. Mousavi and M. Fazli, Effect of nanostructure alumina on adsorption of heavy metals, Desalination, 2010, 253, 94–100 CrossRef CAS.
- A. Haleem, F. Wu, M. Ullah, T. Saeed, H. Li and J. Pan, Chitosan functionalization with vinyl monomers via ultraviolet illumination under cryogenic conditions for efficient palladium recovery from waste electronic materials, Sep. Purif. Technol., 2024, 329, 125213 CrossRef CAS.
- A. Haleem, J.-M. Pan, A. Shah, H. Hussain and W.-D. He, A systematic review on new advancement and assessment of emerging polymeric cryogels for environmental sustainability and energy production, Sep. Purif. Technol., 2023, 316, 123678 CrossRef CAS.
- M. Ajmal, R. A. Rao, R. Ahmad, J. Ahmad and L. A. Rao, Removal and recovery of heavy metals from electroplating wastewater by using Kyanite as an adsorbent, J. Hazard. Mater., 2001, 87, 127–137 CrossRef CAS PubMed.
- G. Sibi, Factors influencing heavy metal removal by microalgae—A review, J. Crit. Rev., 2019, 6, 29–32 Search PubMed.
- M. Matouq, N. Jildeh, M. Qtaishat, M. Hindiyeh and M. Q. Al Syouf, The adsorption kinetics and modeling for heavy metals removal from wastewater by Moringa pods, J. Environ. Chem. Eng., 2015, 3, 775–784 CrossRef CAS.
- H. Bhandari, S. Garg and R. Gaba, Advanced nanocomposites for removal of heavy metals from wastewater, in Proceedings of the Macromol. Symp., 2021, p. 2000337 Search PubMed.
- S. Srivastava, S. Agrawal and M. Mondal, A review on progress of heavy metal removal using adsorbents of microbial and plant origin, Environ. Sci. Pollut. Res., 2015, 22, 15386–15415 CrossRef PubMed.
- A. Haleem, J. Chen, X.-X. Guo, J.-Y. Wang, H.-J. Li, P.-Y. Li, S.-Q. Chen and W.-D. He, Hybrid cryogels composed of P (NIPAM-co-AMPS) and metal nanoparticles for rapid reduction of p-nitrophenol, Polymer, 2020, 193, 122352 CrossRef CAS.
- I. Ali, New generation adsorbents for water treatment, Chem. Rev., 2012, 112, 5073–5091 CrossRef CAS PubMed.
- S. Chen, Q. Yue, B. Gao and X. Xu, Equilibrium and kinetic adsorption study of the adsorptive removal of Cr (VI) using modified wheat residue, J. Colloid Interface Sci., 2010, 349, 256–264 CrossRef CAS PubMed.
- A. Bhattacharya, T. Naiya, S. Mandal and S. Das, Adsorption, kinetics and equilibrium studies on removal of Cr (VI) from aqueous solutions using different low-cost adsorbents, Chem. Eng. J., 2008, 137, 529–541 CAS.
- S. Afroze and T. K. Sen, A review on heavy metal ions and dye adsorption from water by agricultural solid waste adsorbents, Water, Air, Soil Pollut., 2018, 229, 1–50 CrossRef CAS.
- A. Raees, H. N. Bhatti, S. Alshehri, F. Aslam, F. F. Al-Fawzan, S. A. Alissa, M. Iqbal and A. Nazir, Adsorption Potential of Schizophyllum commune White Rot Fungus for Degradation of Reactive Dye and Condition Optimization: A Thermodynamic and Kinetic Study, Adsorpt. Sci. Technol., 2023, 2023, 4725710 CrossRef.
- A. Ahmed, A. Singh, B. Padha, A. K. Sundramoorthy, A. Tomar and S. Arya, UV–vis spectroscopic method for detection and removal of heavy metal ions in water using Ag doped ZnO nanoparticles, Chemosphere, 2022, 303, 135208 CrossRef CAS PubMed.
- T. A. Aragaw and F. M. Bogale, Biomass-based adsorbents for removal of dyes from wastewater: a review, Front. Environ. Sci., 2021, 9, 558 Search PubMed.
- Renu, M. Agarwal and K. Singh, Heavy metal removal from wastewater using various adsorbents: a review, J. Water Reuse Desalin., 2017, 7, 387–419 CrossRef.
- L. Y. Yee, N. M. Asrul, F. F. Kamarulzaman, T. G. Ali, N. S. E. Zulkifli, A. F. Kamaruddin, N. Attan, M. A. M. Huri and A. S. A. Keyon, Recent advances in agricultural waste-based adsorbents for the removal of pollutants in water (2017-2020), Malays. J. Anal. Sci., 2021, 25, 415–431 Search PubMed.
- D. Sud, G. Mahajan and M. Kaur, Agricultural waste material as potential adsorbent for sequestering heavy metal ions from aqueous solutions–A review, Bioresour. Technol., 2008, 99, 6017–6027 CrossRef CAS PubMed.
- A. C. Blaga, C. Zaharia and D. Suteu, Polysaccharides as support for microbial biomass-based adsorbents with applications in removal of heavy metals and dyes, Polymers, 2021, 13, 2893 CrossRef CAS PubMed.
- J. Nyika and M. O. Dinka, The application of microorganism-derived biosorbents in the removal of heavy metals and dyes, in Adsorption Applications for Environmental Sustainability, IOP Publishing, Bristol, UK, 2023, pp. 1–22 Search PubMed.
- A. N. Doyo, R. Kumar and M. Barakat, Recent advances in cellulose, chitosan, and alginate based biopolymeric composites for adsorption of heavy metals from wastewater, J. Taiwan Inst. Chem. Eng., 2023, 151, 105095 CrossRef CAS.
- S. S. Hassan, M. Abd El-Aziz, A. E.-S. Fayez, A. H. Kamel and A. Youssef, Synthesis and characterization of bio-nanocomposite based on chitosan and CaCO3 nanoparticles for heavy metals removal, Int. J. Biol. Macromol., 2024, 255, 128007 CrossRef CAS PubMed.
- S.-W. Lv, J.-M. Liu, C.-Y. Li, N. Zhao, Z.-H. Wang and S. Wang, A novel and universal metal-organic frameworks sensing platform for selective detection and efficient removal of heavy metal ions, Chem. Eng. J., 2019, 375, 122111 CrossRef CAS.
- A. Chakraborty, S. Bhattacharyya, A. Hazra, A. C. Ghosh and T. K. Maji, Post-synthetic metalation in an anionic MOF for efficient catalytic activity and removal of heavy metal ions from aqueous solution, Chem. Commun., 2016, 52, 2831–2834 RSC.
- J. Kim, H.-J. Yang, G. Ha, S.-J. Shin, S.-J. Jeong and D.-Y. Jeong, Biosorption of arsenite (As [III]) using Saccharomyces cerevisiae SRCM 501804 isolated from Korean turbid rice wine, Prep. Biochem. Biotechnol., 2022, 52, 913–923 CrossRef CAS PubMed.
- N. D. Rudd, H. Wang, E. M. Fuentes-Fernandez, S. J. Teat, F. Chen, G. Hall, Y. J. Chabal and J. Li, Highly efficient luminescent metal–organic framework for the simultaneous detection and removal of heavy metals from water, ACS Appl. Mater. Interfaces, 2016, 8, 30294–30303 CrossRef CAS PubMed.
- D. T. Sun, L. Peng, W. S. Reeder, S. M. Moosavi, D. Tiana, D. K. Britt, E. Oveisi and W. L. Queen, Rapid, selective heavy metal removal from water by a metal–organic framework/polydopamine composite, ACS Cent. Sci., 2018, 4, 349–356 CrossRef CAS PubMed.
- E. A. Gendy, J. Ifthikar, J. Ali, D. T. Oyekunle, Z. Elkhlifia, I. I. Shahib, A. I. Khodair and Z. Chen, Removal of heavy metals by covalent organic frameworks (COFs): a review on its mechanism and adsorption properties, J. Environ. Chem. Eng., 2021, 9, 105687 CrossRef CAS.
- R. Sen Gupta, P. K. Samantaray and S. Bose, Going beyond Cellulose and Chitosan: Synthetic Biodegradable Membranes for Drinking Water, Wastewater, and Oil–Water Remediation, ACS Omega, 2023, 8, 24695–24717 CrossRef CAS PubMed.
- N. Kaya, F. Arslan and Z. Yildiz Uzun, Production and characterization of carbon-based adsorbents from waste lignocellulosic biomass: their effectiveness in heavy metal removal, Fullerenes, Nanotubes Carbon Nanostruct., 2020, 28, 769–780 CrossRef CAS.
- F. A. Al-Khaldi, B. Abusharkh, M. Khaled, M. A. Atieh, M. Nasser, T. A. Saleh, S. Agarwal, I. Tyagi and V. K. Gupta, Adsorptive removal of cadmium (II) ions from liquid phase using acid modified carbon-based adsorbents, J. Mol. Liq., 2015, 204, 255–263 CrossRef.
- T. Anirudhan and S. Sreekumari, Adsorptive removal of heavy metal ions from industrial effluents using activated carbon derived from waste coconut buttons, J. Environ. Sci., 2011, 23, 1989–1998 CrossRef CAS PubMed.
- Y. Fei and Y. H. Hu, Design, synthesis, and performance of adsorbents for heavy metal removal from wastewater: a review, J. Mater. Chem. A, 2022, 10, 1047–1085 RSC.
- N. M. Bandaru, N. Reta, H. Dalal, A. V. Ellis, J. Shapter and N. H. Voelcker, Enhanced adsorption of mercury ions on thiol derivatized single wall carbon nanotubes, J. Hazard. Mater., 2013, 261, 534–541 CrossRef CAS PubMed.
- S. P. Moussavi, A. Kadier, R. Singh, R. Ashoori, M. Shirinkar, J. Lu, N. S. Zaidi and F. Sher, Superior removal of humic acid from aqueous stream using novel calf bones charcoal nanoadsorbent in a reversible process, Chemosphere, 2022, 301, 134673 CrossRef CAS PubMed.
- S. S. Hassan, N. S. Awwad and A. H. Aboterika, Removal of mercury (II) from wastewater using camel bone charcoal, J. Hazard. Mater., 2008, 154, 992–997 CrossRef CAS PubMed.
- A. Dawlet, D. Talip and H. Y. Mi, Removal of mercury from aqueous solution using sheep bone charcoal, Procedia Environ. Sci., 2013, 18, 800–808 CrossRef CAS.
- J. Goel, K. Kadirvelu and C. Rajagopal, Competitive sorption of Cu (II), Pb (II) and Hg (II) ions from aqueous solution using coconut shell-based activated carbon, Adsorpt. Sci. Technol., 2004, 22, 257–273 CrossRef CAS.
- S. Parlayici, V. Eskizeybek, A. Avcı and E. Pehlivan, Removal of chromium (VI) using activated carbon-supported-functionalized carbon nanotubes, J. Nanostruct. Chem., 2015, 5, 255–263 CrossRef CAS.
- P. Janik, B. Zawisza, E. Talik and R. Sitko, Selective adsorption and determination of hexavalent chromium ions using graphene oxide modified with amino silanes, Microchim. Acta, 2018, 185, 1–8 CrossRef CAS PubMed.
- A. Alija, D. Gashi, R. Plakaj, A. Omaj, V. Thaçi, A. Reka, S. Avdiaj and A. Berisha, A theoretical and experimental study of the adsorptive removal of hexavalent chromium ions using graphene oxide as an adsorbent, Open Chem., 2020, 18, 936–942 CAS.
- V. P. Chauke, A. Maity and A. Chetty, High-performance towards removal of toxic hexavalent chromium from aqueous solution using graphene oxide-alpha cyclodextrin-polypyrrole nanocomposites, J. Mol. Liq., 2015, 211, 71–77 CrossRef CAS.
- M. Šolić, S. Maletić, M. K. Isakovski, J. Nikić, M. Watson, Z. Kónya and S. Rončević, Removing low levels of Cd (II) and Pb (II) by adsorption on two types of oxidized multiwalled carbon nanotubes, J. Environ. Chem. Eng., 2021, 9, 105402 CrossRef.
- G. Bhanjana, N. Dilbaghi, K.-H. Kim and S. Kumar, Carbon nanotubes as sorbent material for removal of cadmium, J. Mol. Liq., 2017, 242, 966–970 CrossRef CAS.
- M. K. AlOmar, M. A. Alsaadi, M. Hayyan, S. Akib and M. A. Hashim, Functionalization of CNTs surface with phosphonuim based deep eutectic solvents for arsenic removal from water, Appl. Surf. Sci., 2016, 389, 216–226 CrossRef CAS.
- I. Ali, Microwave assisted economic synthesis of multi walled carbon nanotubes for arsenic species removal in water: batch and column operations, J. Mol. Liq., 2018, 271, 677–685 CrossRef CAS.
- L.-K. Wu, H. Wu, H.-B. Zhang, H.-Z. Cao, G.-Y. Hou, Y.-P. Tang and G.-Q. Zheng, Graphene oxide/CuFe2O4 foam as an efficient absorbent for arsenic removal from water, Chem. Eng. J., 2018, 334, 1808–1819 CrossRef CAS.
- A. Sherlala, A. Raman, M. M. Bello and A. Buthiyappan, Adsorption of arsenic using chitosan magnetic graphene oxide nanocomposite, J. Environ. Manage., 2019, 246, 547–556 CrossRef CAS PubMed.
- H. Su, Z. Ye and N. Hmidi, High-performance iron oxide–graphene oxide nanocomposite adsorbents for arsenic removal, Colloids Surf., A, 2017, 522, 161–172 CrossRef CAS.
- A. S. Ibupoto, U. A. Qureshi, M. Arain, F. Ahmed, Z. Khatri, R. Z. Brohi, I. S. Kim and Z. Ibupoto, Zno/Carbon nanofibers for efficient adsorption of lead from aqueous solutions, Environ. Technol., 2019, 41, 2731–2741 CrossRef PubMed.
- M. G. Azam, M. H. Kabir, M. A. A. Shaikh, S. Ahmed, M. Mahmud and S. Yasmin, A rapid and efficient adsorptive removal of lead from water using graphene oxide prepared from waste dry cell battery, J. Water Process Eng., 2022, 46, 102597 CrossRef.
- G. D. Vuković, A. D. Marinković, S. D. Škapin, M. Đ. Ristić, R. Aleksić, A. A. Perić-Grujić and P. S. Uskoković, Removal of lead from water by amino modified multi-walled carbon nanotubes, Chem. Eng. J., 2011, 173, 855–865 CrossRef.
- B. Hayati, A. Maleki, F. Najafi, H. Daraei, F. Gharibi and G. McKay, Super high removal capacities of heavy metals (Pb2+ and Cu2+) using CNT dendrimer, J. Hazard. Mater., 2017, 336, 146–157 CrossRef CAS PubMed.
- S. Kumar, R. R. Nair, P. B. Pillai, S. N. Gupta, M. Iyengar and A. K. Sood, Graphene oxide–MnFe2O4 magnetic nanohybrids for efficient removal of lead and arsenic from water, ACS Appl. Mater. Interfaces, 2014, 6, 17426–17436 CrossRef CAS PubMed.
- X. Guo, B. Du, Q. Wei, J. Yang, L. Hu, L. Yan and W. Xu, Synthesis of amino functionalized magnetic graphenes composite material and its application to remove Cr (VI), Pb (II), Hg (II), Cd (II) and Ni (II) from contaminated water, J. Hazard. Mater., 2014, 278, 211–220 CrossRef CAS PubMed.
- N. Wang, M. Wang, H. Quan, S. Wang and D. Chen, Waste Camellia oleifera shell-derived hierarchically porous carbon modified by Fe3O4 nanoparticles for capacitive removal of heavy metal ions, Sep. Purif. Technol., 2024, 329, 125184 CrossRef CAS.
- Y. Dai, Q. Sun, W. Wang, L. Lu, M. Liu, J. Li, S. Yang, Y. Sun, K. Zhang and J. Xu, Utilizations of agricultural waste as adsorbent for the removal of contaminants: a review, Chemosphere, 2018, 211, 235–253 CrossRef CAS PubMed.
- T. Nguyen, H. Ngo, W. Guo, J. Zhang, S. Liang, Q. Yue, Q. Li and T. Nguyen, Applicability of agricultural waste and by-products for adsorptive removal of heavy metals from wastewater, Bioresour. Technol., 2013, 148, 574–585 CrossRef CAS PubMed.
- T. K. Sen, Agricultural Solid Wastes Based Adsorbent Materials in the Remediation of Heavy Metal Ions from Water and Wastewater by Adsorption: A Review, Molecules, 2023, 28, 5575 CrossRef CAS PubMed.
- C. Jeyaseelan and A. Gupta, Green tea leaves as a natural adsorbent for the removal of Cr (VI) from aqueous solutions, Air, Soil Water Res., 2016, 9, S35227 CrossRef.
- V. Sarin and K. K. Pant, Removal of chromium from industrial waste by using eucalyptus bark, Bioresour. Technol., 2006, 97, 15–20 CrossRef CAS PubMed.
- U. K. Garg, M. Kaur, V. Garg and D. Sud, Removal of hexavalent chromium from aqueous solution by agricultural waste biomass, J. Hazard. Mater., 2007, 140, 60–68 CrossRef CAS PubMed.
- Y. H. Rao and K. Ravindhranath, REMOVAL OF CHROMIUM (VI) FROM WATER USING BIO-ADSORBENTS DERIVED FROM LEAVES OF Salvadora persico AND Caesalpinia bonduc PLANTS, Rasayan J. Chem., 2017, 10, 1104–1113 CAS.
- Y. Han, X. Cao, X. Ouyang, S. P. Sohi and J. Chen, Adsorption kinetics of magnetic biochar derived from peanut hull on removal of Cr (VI) from aqueous solution: effects of production conditions and particle size, Chemosphere, 2016, 145, 336–341 CrossRef CAS PubMed.
- A. N. Babu, G. K. Mohan and K. Ravindhranath, Removal of Chromium (VI) from Polluted waters using Adsorbents derived from Chenopodium album and Eclipta prostrate Plant Materials, Int. J. ChemTech Res., 2016, 9, 506–516 CAS.
- A. Dubey, A. Mishra and S. Singhal, Application of dried plant biomass as novel low-cost adsorbent for removal of cadmium from aqueous solution, Int. J. Environ. Sci. Technol., 2014, 11, 1043–1050 CrossRef CAS.
- V. C. Taty-Costodes, H. Fauduet, C. Porte and A. Delacroix, Removal of Cd (II) and Pb (II) ions, from aqueous solutions, by adsorption onto sawdust of Pinus sylvestris, J. Hazard. Mater., 2003, 105, 121–142 CrossRef CAS PubMed.
- A. Pérez-Marín, V. M. Zapata, J. Ortuno, M. Aguilar, J. Sáez and M. Lloréns, Removal of cadmium from aqueous solutions by adsorption onto orange waste, J. Hazard. Mater., 2007, 139, 122–131 CrossRef PubMed.
- U. Garg, M. Kaur, G. Jawa, D. Sud and V. Garg, Removal of cadmium (II) from aqueous solutions by adsorption on agricultural waste biomass, J. Hazard. Mater., 2008, 154, 1149–1157 CrossRef CAS PubMed.
- V. K. Gupta, C. K. Jain, I. Ali, M. Sharma and V. Saini, Removal of cadmium and nickel from wastewater using bagasse fly ash—a sugar industry waste, Water Res., 2003, 37, 4038–4044 CrossRef CAS PubMed.
- P. S. Kumar, S. Ramalingam, V. Sathyaselvabala, S. D. Kirupha, A. Murugesan and S. Sivanesan, Removal of cadmium (II) from aqueous solution by agricultural waste cashew nut shell, Korean J. Chem. Eng., 2012, 29, 756–768 CrossRef CAS.
- U. Farooq, M. A. Khan, M. Athar and J. A. Kozinski, Effect of modification of environmentally friendly biosorbent wheat (Triticum aestivum) on the biosorptive removal of cadmium (II) ions from aqueous solution, Chem. Eng. J., 2011, 171, 400–410 CrossRef CAS.
- B. Abbar, A. Alem, S. Marcotte, A. Pantet, N.-D. Ahfir, L. Bizet and D. Duriatti, Experimental investigation on removal of heavy metals (Cu2+, Pb2+, and Zn2+) from aqueous solution by flax fibres, Process Saf. Environ. Prot., 2017, 109, 639–647 CrossRef CAS.
- K. Lavanya, J. A. K. Florence, B. Vivekanandan and R. Lakshmipathy, Comparative investigations of raw and alkali metal free banana peel as adsorbent for the removal of Hg2+ ions, Mater. Today: Proc., 2022, 55, 321–326 CAS.
- S. Giraldo, I. Robles, A. Ramirez, E. Flórez and N. Acelas, Mercury removal from wastewater using agroindustrial waste adsorbents, SN Appl. Sci., 2020, 2, 1–17 Search PubMed.
- H. Wang, Y. Liu, J. Ifthikar, L. Shi, A. Khan, Z. Chen and Z. Chen, Towards a better understanding on mercury adsorption by magnetic bio-adsorbents with γ-Fe2O3 from pinewood sawdust derived hydrochar: influence of atmosphere in heat treatment, Bioresour. Technol., 2018, 256, 269–276 CrossRef CAS PubMed.
- M. Mohammadi, K. Shamsi, A. Dargahi and P. Sekhavat, Mercury removal from aqueous solutions by palm leaves adsorbent, J. Adv. Environ. Health Res., 2017, 5, 101–107 CAS.
- M. Alimohammadi, Z. Saeedi, B. Akbarpour, H. Rasoulzadeh, K. Yetilmezsoy, M. A. Al-Ghouti, M. Khraisheh and G. McKay, Adsorptive removal of arsenic and mercury from aqueous solutions by eucalyptus leaves, Water, Air, Soil Pollut., 2017, 228, 1–27 CrossRef CAS.
- M. A. Al-Ghouti, D. Da'ana, M. Abu-Dieyeh and M. Khraisheh, Adsorptive removal of mercury from water by adsorbents derived from date pits, Sci. Rep., 2019, 9, 15327 CrossRef PubMed.
- M. M. Rao, G. C. Rao, K. Seshaiah, N. Choudary and M. Wang, Activated carbon from Ceiba pentandra hulls, an agricultural waste, as an adsorbent in the removal of lead and zinc from aqueous solutions, Waste Manage., 2008, 28, 849–858 CrossRef CAS PubMed.
- M. Jalali and F. Aboulghazi, Sunflower stalk, an agricultural waste, as an adsorbent for the removal of lead and cadmium from aqueous solutions, J. Mater. Cycles Waste Manage., 2013, 15, 548–555 CrossRef CAS.
- S. Kokate, K. Parasuraman and H. Prakash, Adsorptive removal of lead ion from water using banana stem scutcher generated in fiber extraction process, Results Eng., 2022, 14, 100439 CrossRef CAS.
- B. A. Ezeonuegbu, D. A. Machido, C. M. Whong, W. S. Japhet, A. Alexiou, S. T. Elazab, N. Qusty, C. A. Yaro and G. E.-S. Batiha, Agricultural waste of sugarcane bagasse as efficient adsorbent for lead and nickel removal from untreated wastewater: biosorption, equilibrium isotherms, kinetics and desorption studies, Biotechnol. Rep., 2021, 30, e00614 CrossRef CAS PubMed.
- N. E. Ibisi and C. A. Asoluka, Use of agro-waste (Musa paradisiaca peels) as a sustainable biosorbent for toxic metal ions removal from contaminated water, Chem. Int., 2018, 4, 52 CAS.
- S. F. S. Draman, N. Mohd, N. H. I. Wahab, N. S. Zulkfli and N. Bakar, Adsorption of lead (II) ions in aqueous solution using selected agro-waste, ARPN J. Eng. Appl. Sci., 2015, 10, 297–300 Search PubMed.
- P. Muthusamy and S. Murugan, Removal of lead ion using maize cob as a bioadsorbent, Int. J. Eng. Res. Ind. Appl., 2016, 6, 05–10 Search PubMed.
- A. Adesanmi, A. Evuti, Y. Aladeitan and A. Abba, Utilization of waste in solving environmental problem: application of banana and orange peels for the removal of lead (II) ions from aqueous solution of lead nitrate, Nigeria J. Eng. Sci. Technol. Res., 2020, 6, 18–33 Search PubMed.
- P. Karimi, S. Javanshir, M. H. Sayadi and H. Arabyarmohammadi, Arsenic removal from mining effluents using plant-mediated, green-synthesized iron nanoparticles, Processes, 2019, 7, 759 CrossRef CAS.
- J. Nikić, A. Tubić, M. Watson, S. Maletić, M. Šolić, T. Majkić and J. Agbaba, Arsenic removal from water by green synthesized magnetic nanoparticles, Water, 2019, 11, 2520 CrossRef.
- V. Kamath, P. Chandra and G. P. Jeppu, Comparative study of using five different leaf extracts in the green synthesis of iron oxide nanoparticles for removal of arsenic from water, Int. J. Phytorem., 2020, 22, 1278–1294 CrossRef CAS PubMed.
- K. Manquián-Cerda, E. Cruces, M. A. Rubio, C. Reyes and N. Arancibia-Miranda, Preparation of nanoscale iron (oxide, oxyhydroxides and zero-valent) particles derived from blueberries: reactivity, characterization and removal mechanism of arsenate, Ecotoxicol. Environ. Saf., 2017, 145, 69–77 CrossRef PubMed.
- S. Yang, Y. Wu, A. Aierken, M. Zhang, P. Fang, Y. Fan and Z. Ming, Mono/competitive adsorption of Arsenic (III) and Nickel (II) using modified green tea waste, J. Taiwan Inst. Chem. Eng., 2016, 60, 213–221 CrossRef CAS.
- K. S. Prasad, P. Gandhi and K. Selvaraj, Synthesis of green nano iron particles (GnIP) and their application in adsorptive removal of As (III) and As (V) from aqueous solution, Appl. Surf. Sci., 2014, 317, 1052–1059 CrossRef CAS.
- A. Dutta, Y. Diao, R. Jain, E. R. Rene and S. Dutta, Adsorption of cadmium from aqueous solutions onto coffee grounds and wheat straw: equilibrium and kinetic study, J. Environ. Eng., 2016, 142, C4015014 CrossRef.
- V. Gupta and A. Nayak, Cadmium removal and recovery from aqueous solutions by novel adsorbents prepared from orange peel and Fe2O3 nanoparticles, Chem. Eng. J., 2012, 180, 81–90 CrossRef CAS.
- P. Senthil Kumar, S. Ramalingam, R. V. Abhinaya, S. D. Kirupha, A. Murugesan and S. Sivanesan, Adsorption of metal ions onto the chemically modified agricultural waste, Clean: Soil, Air, Water, 2012, 40, 188–197 Search PubMed.
- N. Akunwa, M. Muhammad and J. C. Akunna, Treatment of metal-contaminated wastewater: a comparison of low-cost biosorbents, J. Environ. Manage., 2014, 146, 517–523 CrossRef CAS PubMed.
- Q. Li, L. Chai and W. Qin, Cadmium (II) adsorption on esterified spent grain: equilibrium modeling and possible mechanisms, Chem. Eng. J., 2012, 197, 173–180 CrossRef CAS.
- D. K. Mondal, B. K. Nandi and M. Purkait, Removal of mercury (II) from aqueous solution using bamboo leaf powder: equilibrium, thermodynamic and kinetic studies, J. Environ. Chem. Eng., 2013, 1, 891–898 CrossRef CAS.
- Z. Cheng, K. Feng, Y. Su, J. Ye, D. Chen, S. Zhang, X. Zhang and D. D. Dionysiou, Novel biosorbents synthesized from fungal and bacterial biomass and their applications in the adsorption of volatile organic compounds, Bioresour. Technol., 2020, 300, 122705 CrossRef CAS PubMed.
- A. Priya, L. Gnanasekaran, K. Dutta, S. Rajendran, D. Balakrishnan and M. Soto-Moscoso, Biosorption of heavy metals by microorganisms: evaluation of different underlying mechanisms, Chemosphere, 2022, 307, 135957 CrossRef CAS PubMed.
- A. Javaid, R. Bajwa, U. Shafique and J. Anwar, Removal of heavy metals by adsorption on Pleurotus ostreatus, Biomass Bioenergy, 2011, 35, 1675–1682 CrossRef CAS.
- S. Ilhan, M. N. Nourbakhsh, S. Kiliçarslan and H. Ozdag, Removal of chromium, lead and copper ions from industrial waste waters by Staphylococcus saprophyticus, Turk. Electron. J. Biotechnol., 2004, 2, 50–57 Search PubMed.
- A. Dadrasnia, K. S. Chuan Wei, N. Shahsavari, M. S. Azirun and S. Ismail, Biosorption potential of Bacillus salmalaya strain 139SI for removal of Cr (VI) from aqueous solution, Int. J. Environ. Res. Public Health, 2015, 12, 15321–15338 CrossRef CAS PubMed.
- P. Indhumathi, P. Syed Shabudeen, U. Shoba and C. Saraswathy, The removal of chromium from aqueous solution by using green micro algae, J. Chem. Pharm. Res., 2014, 6, 799–808 CAS.
- K. Nithya, A. Sathish, K. Pradeep and S. K. Baalaji, Algal biomass waste residues of Spirulina platensis for chromium adsorption and modeling studies, J. Environ. Chem. Eng., 2019, 7, 103273 CrossRef CAS.
- Z. Mahmood, S. Zahra, M. Iqbal, M. A. Raza and S. Nasir, Comparative study of natural and modified biomass of Sargassum sp. for removal of Cd 2+ and Zn 2+ from wastewater, Appl. Water Sci., 2017, 7, 3469–3481 CrossRef CAS.
- C. Devatha and S. Shivani, Novel application of maghemite nanoparticles coated bacteria for the removal of cadmium from aqueous solution, J. Environ. Manage., 2020, 258, 110038 CrossRef CAS PubMed.
- Z. Chen, A. I. Osman, D. W. Rooney, W.-D. Oh and P.-S. Yap, Remediation of heavy metals in polluted water by immobilized algae: current applications and future perspectives, Sustainability, 2023, 15, 5128 CrossRef CAS.
- T. Limcharoensuk, N. Sooksawat, A. Sumarnrote, T. Awutpet, M. Kruatrachue, P. Pokethitiyook and C. Auesukaree, Bioaccumulation and biosorption of Cd2+ and Zn2+ by bacteria isolated from a zinc mine in Thailand, Ecotoxicol. Environ. Saf., 2015, 122, 322–330 CrossRef CAS PubMed.
- Y.-G. Liu, F. Ting, G.-m. Zeng, L. Xin, T. Qing, Y. Fei, Z. Ming, W.-h. Xu and Y.-e. Huang, Removal of cadmium and zinc ions from aqueous solution by living Aspergillus niger, Trans. Nonferrous Met. Soc. China, 2006, 16, 681–686 CrossRef CAS.
- X. Wen, C. Du, G. Zeng, D. Huang, J. Zhang, L. Yin, S. Tan, L. Huang, H. Chen and G. Yu, A novel biosorbent prepared by immobilized Bacillus licheniformis for lead removal from wastewater, Chemosphere, 2018, 200, 173–179 CrossRef CAS PubMed.
- V. Javanbakht, H. Zilouei and K. Karimi, Lead biosorption by different morphologies of fungus Mucor indicus, Int. Biodeterior. Biodegrad., 2011, 65, 294–300 CrossRef CAS.
- M. Hanbali, H. Holail and H. Hammud, Remediation of lead by pretreated red algae: adsorption isotherm, kinetic, column modeling and simulation studies, Green Chem. Lett. Rev., 2014, 7, 342–358 CrossRef.
- D. Sriharsha, L. Kumar and J. Savitha, Immobilized fungi on Luffa cylindrica: an effective biosorbent for the removal of lead, J. Taiwan Inst. Chem. Eng., 2017, 80, 589–595 CrossRef.
- N. A. Negm, M. G. Abd El Wahed, A. R. A. Hassan and M. T. Abou Kana, Feasibility of metal adsorption using brown algae and fungi: effect of biosorbents structure on adsorption isotherm and kinetics, J. Mol. Liq., 2018, 264, 292–305 CrossRef CAS.
- M. M. Areco, S. Hanela, J. Duran and M. dos Santos Afonso, Biosorption of Cu (II), Zn (II), Cd (II) and Pb (II) by dead biomasses of green alga Ulva lactuca and the development of a sustainable matrix for adsorption implementation, J. Hazard. Mater., 2012, 213, 123–132 CrossRef PubMed.
- A. Sarı, Ö. D. Uluozlü and M. Tüzen, Equilibrium, thermodynamic and kinetic investigations on biosorption of arsenic from aqueous solution by algae (Maugeotia genuflexa) biomass, Chem. Eng. J., 2011, 167, 155–161 CrossRef.
- A. H. Sulaymon, A. Mohammed and T. Al-Musawi, Column biosorption of lead, cadmium, copper, and arsenic ions onto algae, J. Bioprocess. Biotech., 2013, 3, 1–7 Search PubMed.
- S. Asadi Haris, W. A. H. Altowayti, Z. Ibrahim and S. Shahir, Arsenic biosorption using pretreated biomass of psychrotolerant Yersinia sp. strain SOM-12D3 isolated from Svalbard, Arctic, Environ. Sci. Pollut. Res., 2018, 25, 27959–27970 CrossRef CAS PubMed.
- W. A. H. Altowayti, H. A. Algaifi, S. A. Bakar and S. Shahir, The adsorptive removal of As (III) using biomass of arsenic resistant Bacillus thuringiensis strain WS3: characteristics and modelling studies, Ecotoxicol. Environ. Saf., 2019, 172, 176–185 CrossRef CAS PubMed.
- W. A. H. Altowayti, S. A. Haris, H. Almoalemi, S. Shahir, Z. Zakaria and S. Ibrahim, The removal of arsenic species from aqueous solution by indigenous microbes: batch bioadsorption and artificial neural network model, Environ. Technol. Innovation, 2020, 19, 100830 CrossRef.
- L. Xia, J. Tan, P. Wu, Q. He, S. Song and Y. Li, Biopolymers extracted from Klebsiella sp. and Bacillus sp. in wastewater sludge as superb adsorbents for aqueous Hg (II) removal from water, Chem. Phys. Lett., 2020, 754, 137689 CrossRef CAS.
- S. Singh, V. Kumar, P. Gupta, M. Ray and A. Kumar, The synergy of mercury biosorption through Brevundimonas sp. IITISM22: kinetics, isotherm, and thermodynamic modeling, J. Hazard. Mater., 2021, 415, 125653 CrossRef CAS PubMed.
- G. O. Oyetibo, K. Miyauchi, H. Suzuki, S. Ishikawa and G. Endo, Extracellular mercury sequestration by exopolymeric substances produced by Yarrowia spp.: thermodynamics, equilibria, and kinetics studies, J. Biosci. Bioeng., 2016, 122, 701–707 CrossRef CAS PubMed.
- A. Esmaeili, B. Saremnia and M. Kalantari, Removal of mercury (II) from aqueous solutions by biosorption on the biomass of Sargassum glaucescens and Gracilaria corticata, Arabian J. Chem., 2015, 8, 506–511 CrossRef CAS.
- H. Esfandian, M. Parvini, B. Khoshandam and A. Samadi-Maybodi, Artificial neural network (ANN) technique for modeling the mercury adsorption from aqueous solution using Sargassum Bevanom algae, Desalin. Water Treat., 2016, 57, 17206–17219 CrossRef CAS.
- M. Kumar, A. K. Singh and M. Sikandar, Biosorption of Hg (II) from aqueous solution using algal biomass: kinetics and isotherm studies, Heliyon, 2020, 6, e03321 CrossRef PubMed.
- T. Srinath, T. Verma, P. Ramteke and S. Garg, Chromium (VI) biosorption and bioaccumulation by chromate resistant bacteria, Chemosphere, 2002, 48, 427–435 CrossRef CAS PubMed.
- N. Lokeshwari and K. Joshi, Biosorption of heavy metal (chromium) using biomass, Global J. Environ. Res., 2009, 3, 29–35 CAS.
- W. M. Ibrahim, Biosorption of heavy metal ions from aqueous solution by red macroalgae, J. Hazard. Mater., 2011, 192, 1827–1835 CrossRef CAS PubMed.
- A. Yipmantin, H. J. Maldonado, M. Ly, J. M. Taulemesse and E. Guibal, Pb (II) and Cd (II) biosorption on Chondracanthus chamissoi (a red alga), J. Hazard. Mater., 2011, 185, 922–929 CrossRef CAS PubMed.
- A. Rathinam, B. Maharshi, S. K. Janardhanan, R. R. Jonnalagadda and B. U. Nair, Biosorption of cadmium metal ion from simulated wastewaters using Hypnea valentiae biomass: a kinetic and thermodynamic study, Bioresour. Technol., 2010, 101, 1466–1470 CrossRef CAS PubMed.
- H. Demey, T. Vincent and E. Guibal, A novel algal-based sorbent for heavy metal removal, Chem. Eng. J., 2018, 332, 582–595 CrossRef CAS.
- L. Joseph, B.-M. Jun, J. R. Flora, C. M. Park and Y. Yoon, Removal of heavy metals from water sources in the developing world using low-cost materials: a review, Chemosphere, 2019, 229, 142–159 CrossRef CAS PubMed.
- A. Ahmadi, R. Foroutan, H. Esmaeili and S. Tamjidi, The role of bentonite clay and bentonite clay@ MnFe2O4 composite and their physico-chemical properties on the removal of Cr (III) and Cr (VI) from aqueous media, Environ.
Sci. Pollut. Res., 2020, 27, 14044–14057 CrossRef CAS PubMed.
- H. S. Altundoğan, S. Altundoğan, F. Tümen and M. Bildik, Arsenic adsorption from aqueous solutions by activated red mud, Waste Manage., 2002, 22, 357–363 CrossRef PubMed.
- R. Foroutan, R. Mohammadi, A. S. Adeleye, S. Farjadfard, Z. Esvandi, H. Arfaeinia, G. A. Sorial, B. Ramavandi and S. Sahebi, Efficient arsenic (V) removal from contaminated water using natural clay and clay composite adsorbents, Environ. Sci. Pollut. Res., 2019, 26, 29748–29762 CrossRef CAS PubMed.
- S. Lakshmi Narayanan, G. Venkatesan and I. Vetha Potheher, Equilibrium studies on removal of lead (II) ions from aqueous solution by adsorption using modified red mud, Int. J. Environ. Sci. Technol., 2018, 15, 1687–1698 CrossRef CAS.
- M. Irannajad and H. Kamran Haghighi, Removal of heavy metals from polluted solutions by zeolitic adsorbents: a review, Environ. Processes, 2021, 8, 7–35 CrossRef CAS.
- Y. A. Neolaka, Y. Lawa, J. Naat, A. A. Riwu, A. W. Mango, H. Darmokoesoemo, B. A. Widyaningrum, M. Iqbal and H. S. Kusuma, Efficiency of activated natural zeolite-based magnetic composite (ANZ-Fe3O4) as a novel adsorbent for removal of Cr (VI) from wastewater, J. Mater. Res. Technol., 2022, 18, 2896–2909 CrossRef CAS.
- K. K. Jena, K. S. K. Reddy, G. N. Karanikolos and D. S. Choi, l-Cysteine and silver nitrate based metal sulfide and Zeolite-Y nano adsorbent for efficient removal of mercury (II) ion from wastewater, Appl. Surf. Sci., 2023, 611, 155777 CrossRef CAS.
- M. Araissi, E. Elaloui and Y. Moussaoui, The removal of cadmium, cobalt, and nickel by adsorption with Na-Y zeolite, Iran. J. Chem. Chem. Eng., 2020, 39, 169–179 CAS.
- X. Zhang, Z. Song, Y. Dou, Y. Xue, Y. Ji, Y. Tang and M. Hu, Removal difference of Cr (VI) by modified zeolites coated with MgAl and ZnAl-layered double hydroxides: efficiency, factors and mechanism, Colloids Surf., A, 2021, 621, 126583 CrossRef CAS.
- Z.-d. Peng, X.-m. Lin, Y.-l. Zhang, Z. Hu, X.-j. Yang, C.-y. Chen, H.-y. Chen, Y.-t. Li and J.-j. Wang, Removal of cadmium from wastewater by magnetic zeolite synthesized from natural, low-grade molybdenum, Sci. Total Environ., 2021, 772, 145355 CrossRef CAS PubMed.
- M. Mudasir, K. Karelius, N. H. Aprilita and E. T. Wahyuni, Adsorption of mercury (II) on dithizone-immobilized natural zeolite, J. Environ. Chem. Eng., 2016, 4, 1839–1849 CrossRef CAS.
- S. Zeb, N. Ali, Z. Ali, M. Bilal, B. Adalat, S. Hussain, S. Gul, F. Ali, R. Ahmad and H. M. Iqbal, Silica-based nanomaterials as designer adsorbents to mitigate emerging organic contaminants from water matrices, J. Water Process Eng., 2020, 38, 101675 CrossRef.
- Y. Snoussi, M. Abderrabba and A. Sayari, Removal of cadmium from aqueous solutions by adsorption onto polyethylenimine-functionalized mesocellular silica foam: equilibrium properties, J. Taiwan Inst. Chem. Eng., 2016, 66, 372–378 CrossRef CAS.
- Y. Li, J. He, K. Zhang, T. Liu, Y. Hu, X. Chen, C. Wang, X. Huang, L. Kong and J. Liu, Super rapid removal of copper, cadmium and lead ions from water by NTA-silica gel, RSC Adv., 2019, 9, 397–407 RSC.
- S. Bao, K. Li, P. Ning, J. Peng, X. Jin and L. Tang, Highly effective removal of mercury and lead ions from wastewater by mercaptoamine-functionalised silica-coated magnetic nano-adsorbents: behaviours and mechanisms, Appl. Surf. Sci., 2017, 393, 457–466 CrossRef CAS.
- B. Ekka, L. Rout, M. K. S. A. Kumar, R. K. Patel and P. Dash, Removal efficiency of Pb (II) from aqueous solution
by 1-alkyl-3-methylimidazolium bromide ionic liquid mediated mesoporous silica, J. Environ. Chem. Eng., 2015, 3, 1356–1364 CrossRef CAS.
- J. Zhou, X. Zhou, K. Yang, Z. Cao, Z. Wang, C. Zhou, S. A. Baig and X. Xu, Adsorption behavior and mechanism of arsenic on mesoporous silica modified by iron-manganese binary oxide (FeMnOx/SBA-15) from aqueous systems, J. Hazard. Mater., 2020, 384, 121229 CrossRef CAS PubMed.
- N. Ahmad, H. Sereshti, M. Mousazadeh, H. R. Nodeh, M. A. Kamboh and S. Mohamad, New magnetic silica-based hybrid organic-inorganic nanocomposite for the removal of lead (II) and nickel (II) ions from aqueous solutions, Mater. Chem. Phys., 2019, 226, 73–81 CrossRef CAS.
- Y. Yu, J. Addai-Mensah and D. Losic, Functionalized diatom silica microparticles for removal of mercury ions, Sci. Technol. Adv. Mater., 2012, 13, 5835–5842 CrossRef PubMed.
- Y. Fu, J. Jiang, Z. Chen, S. Ying, J. Wang and J. Hu, Rapid and selective removal of Hg (II) ions and high catalytic performance of the spent adsorbent based on functionalized mesoporous silica/poly (m-aminothiophenol) nanocomposite, J. Mol. Liq., 2019, 286, 110746 CrossRef CAS.
- R. Huang, Q. Lin, Q. Zhong, X. Zhang, X. Wen and H. Luo, Removal of Cd (II) and Pb (II) from aqueous solution by modified attapulgite clay, Arabian J. Chem., 2020, 13, 4994–5008 CrossRef CAS.
- P. Staroń and J. Chwastowski, Raphia-microorganism composite biosorbent for lead ion removal from aqueous solutions, Materials, 2021, 14, 7482 CrossRef PubMed.
- T. Zhang, W. Wang, Y. Zhao, H. Bai, T. Wen, S. Kang, G. Song, S. Song and S. Komarneni, Removal of heavy metals and dyes by clay-based adsorbents: from natural clays to 1D and 2D nano-composites, Chem. Eng. J., 2021, 420, 127574 CrossRef CAS.
|
This journal is © The Royal Society of Chemistry 2024 |