DOI:
10.1039/D3BM02008H
(Minireview)
Biomater. Sci., 2024,
12, 1943-1949
Liquid–liquid phase separation-inspired design of biomaterials
Received
8th December 2023
, Accepted 23rd February 2024
First published on 26th February 2024
Abstract
Liquid–liquid phase separation (LLPS) is a crucial biological process that governs biomolecular condensation, assembly, and functionality within phase-separated aqueous environments. This phenomenon serves as a source of inspiration for the creation of artificial designs in both structured and functional biomaterials, presenting novel strategies for manipulating the structures of functional protein aggregates in a wide range of biomedical applications. This mini review summarizes my past research endeavors, offering a panoramic overview of LLPS-inspired biomaterials utilized in the design of structured materials, the development of cell mimetics, and the advancement of intelligent biomaterials.
 Yang Song | Dr Yang Song is working as an associate professor in the School of Materials Science & Engineering, Shanghai Jiao Tong University. His current research interest is focused on understanding the supramolecular assembly, phase-transition, and functionalization of peptide-based coacervates in phase-separated all-aqueous environments. The new findings should inspire a biomimetic strategy towards programmed design of smart biomaterials, to be used for disease modeling, theranostics, immunomodulation and tissue engineering. |
Introduction
Liquid–liquid phase separation (LLPS) is a biological process that drives the condensation of biomolecules within molecularly crowded aqueous solutions, ultimately resulting in the formation of two immiscible aqueous phases.1 Pioneered by Prof. C. P. Brangwynne, LLPS has been revealed as a key organizational principle for a variety of intracellular organelles,2–4 including P granules, stress granules, the nucleolus, and nuclear bodies, which manifest as liquid condensates. The genesis of these liquid condensates involves the intermolecular aggregation of disordered proteins or RNAs, mediated by multivalent and non-covalent interactions encompassing electrostatic forces, cation–π interactions, hydrophobic effects, hydrogen bonding, and van der Waals forces.5 It is these nuanced interactions that orchestrate the supramolecular assembly of biomolecules, furnishing them with dynamic phase behaviors responsive to environing biochemical cues.6 Biological condensates can undergo transformations among various phase states, such as single-phase solution, coacervate, bi-continuous phase, liquid crystal, and microgel states.7 Such dynamic phase behavior confers a spatial and temporal regulatory mechanism on biomolecules,8 impacting essential cellular processes such as cytoplasmic compartmentalization,9 metabolic regulation,10 signal transduction,11 and gene expression.12
Grounded in these biophysical insights, the design and engineering of biomaterials have been revolutionized. The properties of LLPS extend the framework for developing biomaterials endowed with complex aqueous structures and responsive functionalities, emblematic of the dynamic nature of biological systems.13 Advancements in synthetic biology of LLPS are not only enlarging the horizons of biophysics, but are also unlocking potent applications in the realms of biomaterial science and biomedical engineering.
Fabrication of all-aqueous structured biomaterials with LLPS
LLPS creates two distinct aqueous phases that serve as a means to modulate biomolecules/cells for assembly of biomaterials or bio-integrated structures. These all-aqueous emulsions are superior to traditional water/oil emulsions, as they adeptly maintain the native configurations of proteins and nucleic acids, and sustain cell viability. This offers a cytocompatible liquid scaffolding that is essential for the assembly of bioactive substances and complex biological entities. Segregated LLPS systems, also known as aqueous two-phase systems (ATPSs), have demonstrated their efficacy in enzyme purification14 and meticulous cell patterning.15 Nonetheless, the precise handling of all-aqueous structures, particularly with ultralow interfacial tensions, remains a significant challenge. Steijn16,17 and Shum18,19et al. have pioneered a microfluidic strategy to produce water-in-water emulsions, integrating mechanical disturbances to the inner phase, thereby expediting the uniform breakup of water/water jets (Fig. 1a). Building upon this foundational work, my research within Shum's Lab has adopted an all-aqueous electrospray technique to fabricate water-in-water emulsions (Fig. 1b).20,21 This method introduces an intermediary air phase, which cleverly elevates the surface tension and circumvents the constraints imposed by the slow breakup of jets in low tension systems. Furthermore, the all-aqueous electrospray can easily embed a multitude of gelling agents into the aqueous droplets, which undergo solidification in response to specific triggers such as light, heat, or chemical stimuli, culminating in the production of microgels. This technique provides a robust and adaptable platform for the efficient synthesis of hydrogel particles21 (Fig. 1c and d), capsules (Fig. 1e and f),22 colloidosomes23 as enzyme encapsulants, cell containers and bioreactors, significantly broadening the potential applications of all-aqueous structured biomaterials.
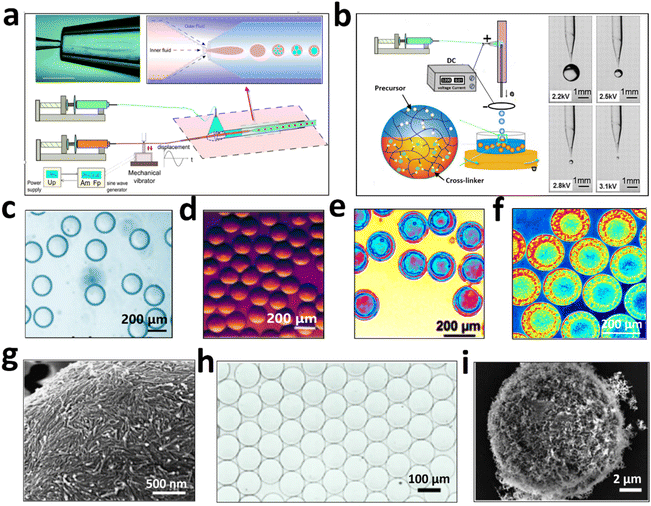 |
| Fig. 1 All-aqueous multiphase microfluidics for preparation of structured biomaterials. (a) Mechanical disturbance-assisted generation of all-aqueous droplets. (b) Electrospray-assisted formation of all-aqueous droplets. (c) Monodisperse W/W droplets; (d) alginate particles templated from W/W droplets; (e) monodisperse W/W/W double emulsion; (f) core–shell structured collagen capsules. (g) W/W emulsion stabilized by a monolayer of fibrils; (h) monodisperse protein colloidosomes; (i) multilayered fibrillosomes. Figures are redrawn and reprinted with permission from ref. 19, 21 and 23. Copyright © 2012, American Chemical Society. Copyright © 2015, American Chemical Society. Open Access © 2016, Nature Publishing Group. | |
Another challenge that needs to be addressed is the lack of stability in all-aqueous emulsion droplets, which hinders the preparation of stable and complex emulsion structures, such as the water/water/water double emulsion. The low interfacial tension between two immiscible aqueous phases often results in the dynamic interfacial adsorption and detachment of colloidal nanoparticles.24 This low energy barrier for colloidal detachment fails to effectively prevent the coalescence of the water/water (W/W) droplets. To overcome this limitation, I propose the use of protein nanofibrils as stabilizers for W/W droplets.23 Protein nanofibrils have a higher specific contact area than spherical nanoparticles, enabling them to better stabilize the W/W droplets. Additionally, protein nanofibrils can be introduced at the W/W interface to facilitate the growth of multilayered capsules through fibril–fibril interactions. The resulting protein colloidosomes, referred to as ‘fibrillosomes’ (Fig. 1g–i), exhibit ultra-thin shell thickness (<100 nm), selective permeability to nanoparticles of varying sizes (30 nm < d< 50 nm), mechanical robustness, and deformability for osmotic inflation. Fibrillosomes can be utilized for encapsulating live cells while preserving their viability. As evidence, we successfully developed mini-scaffolds of fibrillosomes for long-term culture of tumor spheroids (SGC-996) lasting over 30 days.24 The high permeability of oxygen and nutrients across fibrillosomes supports the growth of large tumor spheroids, with reduced hindrance to cell fusion compared to hydrogel particles. These unique features of fibrillosomes make them promising candidates for more efficient applications in anticancer drug screening and tissue engineering.
Modeling of protocell behaviors with the LLPS system
ATPS droplets are ideal for simulating protocells as their aqueous nature preserves biomolecule activity, their crowded environment reflects the viscoelastic cytoplasm conditions aiding the assembly of biomolecules, and their ultralow interfacial tension allows for mimicking the soft and dynamic protocell interface, essential for analyzing cellular deformation under environmental stress or cell–cell contact.25,26 For example, the division of protocells must overcome their surface tension and surmount the energy barrier resulting from an increase in the interfacial area. Such phenomena have been traditionally modeled using liposomes with asymmetric lipid bilayers27 or heterogeneous liquid phases.28 While these liposome-based models can replicate some dynamic features of the morphology of dividing cells, they fail to elucidate the potential influence of protein fibrils and the cytoskeleton network.
A simplistic model of cytoskeleton–cytoplasm interaction involves the loading of protein fibrils into W/W droplets, which mimics the nascent cytoskeletal architecture and the molecularly crowded cytoplasmic milieu of protocells.29 Synthetic protein fibrils, despite not capturing the full complexity of the cytoskeleton, offer a simplified model to study dynamic assembly and decomposition behaviors, resembling the periodical formation and degradation of the actin network observed in budding yeast cells.30 To trigger the contractile movements seen in the cytoskeleton, I also introduce a negative osmotic pressure to induce shrinkage of the droplets and the embedded fibril networks. Notably, upon exceeding the overlapping concentration of the fibrils, the contraction of the W/W droplets is non-uniform, reminiscent of budding phenomena observed in dividing yeast cells (Fig. 2a–c). The morphology of budding and complete fission behaviors of the W/W droplets can be modulated by controlling the degree of dehydration and fibril concentration (Fig. 2d–g). This observation is ascribed to the collective dewetting of fibrils from the W/W droplets. Given that fibrils exhibit higher wetting energy with the droplet phase than with the continuous phase, fibrils tend to translocate toward the continuous phase to reduce the fibril–liquid contact energy. Concurrently, the relocating fibrils are adsorbed and entrapped by the W/W interface, which reduces the interfacial energy of the W/W emulsion. In an effort to minimize the free energy of the entangled system, the fibril networks exert additional tension at the droplet interface, leading to the generation of new interfacial area and subsequent splitting. By augmenting the fibril payload within the droplets, we are able to increase the interfacial area, thereby enhancing support for droplet division. The simple demonstration, although insufficient to reproduce the complex motion of the cytoskeleton, could elucidate the effect of dynamic fibril aggregation and decomposition on modulating the dynamic behaviors of protocells.
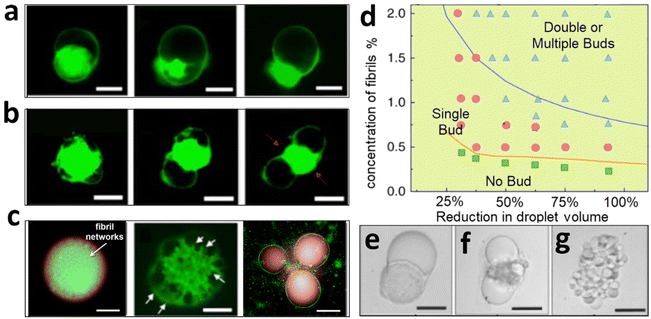 |
| Fig. 2 Modeling of protocell division using an entangled system of LLPS and fibril networks. (a) Dewetting behaviors of fibril networks from a W/W droplet; C(fibrils) = 0.5%. (b) Splitting of W/W droplets modulated with fibril networks; C(fibrils) = 1.0%. (c) Formation of multiple buds from W/W droplets preloaded with condensed fibril networks; C(fibrils) = 1.5%. (d) A phase diagram showing the budding morphology of W/W droplets modulated through the fibril concentration and the degree of dehydration. (e–g) Representative microscopy images of budding droplets. Scale bars, 200 μm. Figures are redrawn and reprinted from ref. 27. Open Access © 2018, Nature Publishing Group. | |
LLPS-inspired design of functional biomaterials
Aside from ATPS, the formation of most membraneless organelles and nuclear bodies is predominantly driven by associative liquid–liquid phase separation (LLPS), frequently referred to as the coacervate system.31 Coacervates are droplets that originate from the supramolecular assembly of intrinsically disordered proteins (IDPs), long non-coding RNAs, polysaccharides, or synthetic polymers. Biologically, coacervates are integral to the compartmentalization of biomolecules,32 catalysis of biochemical reactions,33 and activation of intracellular signaling pathways,34 displaying remarkable responsiveness to environmental stimuli. In the domain of biomimetic materials, coacervates are exploited extensively, with applications spanning underwater adhesives,35 RNA vaccine-delivery vehicles36 and fibrous scaffolds for tissue engineering.37 A growing trend in this area is to design functional coacervates by a strategic programming of peptide sequences for designing specific functional entities.38
The cutting-edge field of designing functional coacervates is now exploring the development of IDPs or RNAs for the diagnosis and treatment of diseases. Traditional coacervates, often constructed from disordered peptides, typically consist of homopolymers with redundant sequences of more than 50 amino acids.39 Such macromolecules are generally hampered by limited efficacy in penetrating cells, which constrains their utility as drug carriers and bioprobes for tissue invasion and disease intervention. However, the recent proposition of the sticker–spacer theory40 has opened the window for designing short peptides that exhibit LLPS characteristics. My laboratory is particularly focused on the creation of bioprobes utilizing these short, phase-separating peptides with the aim of enhancing cellular permeability and advancing therapeutic applications.
Our recent research interest focused on the development of coacervate-based intracellular probes,41 drawing inspiration from the sophisticated transmembrane signaling processes observed in immune cells. Specifically, transmembrane kinase receptors possess a remarkable ability to accumulate intracellular secondary messengers for the formation of coacervates.42 The genesis of these coacervates can subsequently amplify the cascade reactions and activate the signaling pathways. A parallel mechanism of signal amplification is frequently observed within the cellular nucleus. RNA-binding proteins (RNP granules) amalgamate with RNA to engender complex coacervate droplets, which accelerates biochemical reactions through synchronized sequestration of enzymes and reagents.43 Capitalizing on these biological principles, I am interested in connecting coacervation with traditional molecular bioprobes for localized detection of biomarkers. As a preliminary step, my lab has synthesized RNP-mimetic peptides conjugated with aggregation-induced emission fluorophores (AIEgens). We anticipate that upon association with RNA to constitute coacervates, the AIEgen–RNP will incite molecular aggregation of AIEgens, consequently amplifying the fluorescence emission.
The AIEgen-conjugated phase-separating peptides display RNA-concentration-dependent luminescence (Fig. 3a), attributable to the coacervate-induced molecular condensation of AIE fluorophores (CIE).41 At elevated RNA concentrations, a microgel phase emerges (Fig. 3b), beyond which no further enhancement in fluorescence is detected, owing to the saturation of AIE fluorophore aggregation in the solidified matrix. Integration of AIE fluorophores with phase-separating peptides brings additional advantages for the design of bioprobes. First of all, the critical concentration of peptide coacervation is lowered by more than an order of magnitude compared to peptides lacking AIEgens. Second, the phase stability of coacervates is markedly improved in physiologically relevant saline solution, displaying better resistance to salt-induced charge screening. Third, a potent cation–π intermolecular interaction is postulated to facilitate the formation of coacervates with a cationic surface, which may enhance their permeability across tissues and the cell membrane.
 |
| Fig. 3 LLPS-inspired bioprobes for illuminating intracellular RNA through coacervation induced emission. (a) AIEgen-conjugated phase-separating peptides (AIEgen–peptide) emit RNA-dose dependent fluorescence. (b) Phase diagram of AIEgen–peptide/RNA complexes. (c–e) AIEgen–peptide (blue color) can illuminate intracellular RNA and colocalize with a commercial RNA dye (green, SYTO 13). Scale bars, 50 μm. Figures are redrawn and reprinted with permission from ref. 38. Copyright © 2023, American Chemical Society. | |
Extending our research, we have co-cultured these luminogenic entities with a gallbladder cancer cell line, SGC-996, allowing for the intracellular RNA visualization via localized peptide/RNA coacervation (Fig. 3c). Validation of our probe's specific performance was achieved through colocalization with a commercial RNA-selective small-molecule dye (Fig. 3d and e). Notably, our probe exhibits high permeability across the cell membrane and sequence-dependent cytotoxicity, which could be potentially used for bioimaging and cancer therapeutics.
While our research provides an exciting proof-of-concept, there is a clear need for further studies to enhance the specificity of bioprobes targeting diseased RNA foci. Refinement is needed to ensure that these probes are appropriately tailored for the disease-relevant microenvironments found in pathological states. Additionally, the ability of biological coacervates to undergo multistate phase transitions in response to various biological stimuli and signals underscores their potential for use as self-adaptive biomaterials. Nonetheless, the fundamental principles that govern such biorecognition, the resulting phase change and cellular responses remain to be fully elucidated within synthetic systems. Future investigations are required to dissect the intricate relationships between the structure, phase behavior, and function of phase-separating peptides (or RNAs), as well as elucidating the cellular responses to functional coacervates. Deciphering these aspects is essential for advancing the development of bioprobes that could revolutionize clinical diagnostics and therapeutic interventions.
In summary, LLPS-derived biomaterials have several unique advantages for biomedical applications, such as good cytocompatibility due to their structural similarity to membraneless organelles, the ability to recruit and compartmentalize biomolecules from the environment, as well as environmental responsiveness and adaptiveness due to their capacity of phase transition. These merits allow coacervates to be used as cytocompatible adhesives for efficient tissue repair,44,45 smart self-healing hydrogels for tissue engineering,46 encapsulants for delivery of bioactive drugs,47 and functional probes for disease diagnosis and therapy.48
Conflicts of interest
The author declares no competing financial interest.
Acknowledgements
I′d like to thank Prof. Ho Cheung Shum of the University of Hong Kong and Prof. Shuichi Takayama of Georgia Institute of Technology (USA) for their leading roles in supervising these projects. Special thanks to my collaborators Prof. Tuomas PJ Knowles of the University of Cambridge, Prof. Qingming Ma of Qingdao University and many others involved in the work above.
This work is supported by the Natural Science Foundation of Shanghai Municipality (23ZR1433100) and Shanghai Jiao Tong University Medical and Industrial Cross Research Fund (YG2022QN026, YG2023QNB28 and YG2023QNA11).
References
- A. A. Hyman, C. A. Weber and F. Jülicher, Liquid-liquid phase separation in biology, Annu. Rev. Cell Dev. Biol., 2014, 30, 39–58 CrossRef CAS PubMed
.
- C. P. Brangwynne, C. R. Eckmann and D. S. Courson,
et al. Germline P granules are liquid droplets that localize by controlled dissolution/condensation, Science, 2009, 324(5935), 1729–1732 CrossRef CAS PubMed
.
- Y. Shin and C. P. Brangwynne, Liquid phase condensation in cell physiology and disease, Science, 2017, 357(6357), eaaf4382 CrossRef PubMed
.
- S. Mehta and J. Zhang, Liquid–liquid phase separation drives cellular function and dysfunction in cancer, Nat. Rev. Cancer, 2022, 22(4), 239–252 CrossRef CAS PubMed
.
- D. Bracha, M. T. Walls and C. P. Brangwynne, Probing and engineering liquid-phase organelles, Nat. Biotechnol., 2019, 37(12), 1435–1445 CrossRef CAS PubMed
.
- J. Berry, C. P. Brangwynne and M. Haataja, Physical principles of intracellular organization via active and passive phase transitions, Rep. Prog. Phys., 2018, 81(4), 046601 CrossRef PubMed
.
- T. P. Fraccia and G. Zanchetta, Liquid–liquid crystalline phase separation in biomolecular solutions, Curr. Opin. Colloid Interface Sci., 2021, 56, 101500 CrossRef CAS
.
- Q. Su, S. Mehta and J. Zhang, Liquid-liquid phase separation: Orchestrating cell signaling through time and space, Mol. Cell, 2021, 81(20), 4137–4146 CrossRef CAS PubMed
.
- W. M. Aumiller Jr. and C. D. Keating, Experimental models for dynamic compartmentalization of biomolecules in liquid organelles: Reversible formation and partitioning in aqueous biphasic systems, Adv. Colloid Interface Sci., 2017, 239, 75–87 CrossRef PubMed
.
- B. G. O'Flynn and T. Mittag, The role of liquid–liquid phase separation in regulating enzyme activity, Curr. Opin. Cell Biol., 2021, 69, 70–79 CrossRef PubMed
.
- P. A. Chong and J. D. Forman-Kay, Liquid–liquid phase separation in cellular signaling systems, Curr. Opin. Struct. Biol., 2016, 41, 180–186 CrossRef CAS PubMed
.
- S. Mehta and J. Zhang, Liquid–liquid phase separation drives cellular function and dysfunction in cancer, Nat. Rev. Cancer, 2022, 22(4), 239–252 CrossRef CAS PubMed
.
- Q. Ma, Y. Song, W. Sun, J. Cao, H. Yuan, X. Wang, Y. Sun and H. C. Shum, Cell-inspired all-aqueous microfluidics: from intracellular liquid–liquid phase separation toward advanced biomaterials, Adv. Sci., 2020, 7(7), 1903359 CrossRef CAS PubMed
.
- S. S. Nadar, R. G. Pawar and V. K. Rathod, Recent advances in enzyme extraction strategies: A comprehensive review, Int. J. Biol. Macromol., 2017, 101, 931–957 CrossRef CAS PubMed
.
- D. Petrak, E. Atefi, L. Yin, W. Chilian and H. Tavana, Automated, spatio–temporally controlled cell microprinting with polymeric aqueous biphasic system, Biotechnol. Bioeng., 2014, 111(2), 404–412 CrossRef CAS PubMed
.
- I. Ziemecka, V. van Steijn and G. J. M. Koper,
et al. Monodisperse hydrogel microspheres by forced droplet formation in aqueous two-phase systems, Lab Chip, 2011, 11(4), 620–624 RSC
.
- I. Ziemecka, V. Van Steijn and G. J. M. Koper,
et al. All-aqueous core-shell droplets produced in a microfluidic device, Soft Matter, 2011, 7(21), 9878–9880 RSC
.
- H. C. Shum, J. Varnell and D. A. Weitz, Microfluidic fabrication of water-in-water (w/w) jets and emulsions, Biomicrofluidics, 2012, 6(1), 12808 CrossRef PubMed
.
- Y. Song and H. C. Shum, Monodisperse w/w/w double emulsion induced by phase separation, Langmuir, 2012, 28(33), 12054–12059 CrossRef CAS PubMed
.
- Y. Song, A. Sauret and H. C. Shum, All-aqueous multiphase microfluidics, Biomicrofluidics, 2013, 7(6), 061301 CrossRef PubMed
.
- Y. Song, Y. K. Chan, Q. Ma, Z. Liu and H. C. Shum, All-aqueous electrosprayed emulsion for templated fabrication of cytocompatible microcapsules, ACS Appl.
Mater. Interfaces, 2015, 7(25), 13925–13933 CrossRef CAS PubMed
.
- M. Kim, S. J. Yeo, C. B. Highley, J. A. Burdick, P. J. Yoo, J. Doh and D. Lee., One-step generation of multifunctional polyelectrolyte microcapsules via nanoscale interfacial complexation in emulsion (NICE), ACS Nano, 2015, 9(8), 8269–8278 CrossRef CAS PubMed
.
- Y. Song, T. C. T. Michaels, Q. Ma, Z. Liu, H. Yuan, S. Takayama, T. P. J. Knowles and H. C. Shum, Budding-like division of all-aqueous emulsion droplets modulated by networks of protein nanofibrils, Nat. Commun., 2018, 9(1), 2110 CrossRef PubMed
.
-
Y. Wei, Z. Cai, T. Yang, T. Yang, S. Yang, X. Xu, H. Yu, R. Zan, H. Zhang, Z. Liu, C. Liu, T. Kong and Y. Song, All-aqueous Synthesis of Fibrillated Protein Microcapsules for Membrane-bounded Culture of Tumor Spheroids, ChemRxiv 2023, preprint, DOI:10.26434/chemrxiv-2023-101vb.
- J. Liu, L. Tian, Y. Qiao, S. Zhou, A. J. Patil, K. Wang, M. Li and S. Mann, Hydrogel-immobilized coacervate droplets as modular microreactor assemblies, Angew. Chem., Int. Ed., 2020, 59(17), 6853–6859 CrossRef CAS PubMed
.
- P. Gobbo, A. J. Patil, M. Li, R. Harniman, W. H. Briscoe and S. Mann, Programmed assembly of synthetic protocells into thermoresponsive prototissues, Nat. Mater., 2018, 17(12), 1145–1153 CrossRef CAS PubMed
.
- Y. S. Ryu, I.-H. Lee, J.-H. Suh, S. C. Park, S. Oh, L. R. Jordan, N. J. Wittenberg, S.-H. Oh, N. L. Jeon, B. Lee, A. N. Parikh and S.-D. Lee, Reconstituting ring-rafts in bud-mimicking topography of model membranes, Nat. Commun., 2014, 5(1), 4507 CrossRef CAS PubMed
.
- Y. Li, R. Lipowsky and R. Dimova, Membrane nanotubes induced by aqueous phase separation and stabilized by spontaneous curvature, Proc. Natl. Acad. Sci. U. S. A., 2011, 108(12), 4731–4736 CrossRef CAS PubMed
.
- K. A. Ganar, L. W. Honaker and S. Deshpande, Shaping synthetic cells through cytoskeleton-condensate-membrane interactions, Curr. Opin. Colloid Interface Sci., 2021, 54, 101459 CrossRef CAS
.
- R. Li and A. W. Murray, Feedback control of mitosis in budding yeast, Cell, 1991, 66(3), 519–531 CrossRef CAS PubMed
.
- W. Mu, L. Jia, M. Zhou, J. Wu, Y. Lin, S. Mann and Y. Qiao, Superstructural ordering in self-sorting coacervate-based protocell networks, Nat. Chem., 2023, 1–10 Search PubMed
.
- G. A. Mountain and C. D. Keating, Formation of multiphase complex coacervates and partitioning of biomolecules within them, Biomacromolecules, 2019, 21(2), 630–640 CrossRef PubMed
.
- H. Seo and H. Lee, Recent developments in microfluidic synthesis of artificial cell-like polymersomes and liposomes for functional bioreactors, Biomicrofluidics, 2021, 15(2), 021301 CrossRef CAS PubMed
.
- J. A. Riback, L. Zhu, M. C. Ferrolino, M. Tolbert, D. M. Mitrea, D. W. Sanders, M. Wei, R. W. Kriwacki and C. P. Brangwynne, Composition-dependent thermodynamics of intracellular phase separation, Nature, 2020, 581(7807), 209–214 CrossRef CAS PubMed
.
- R. J. Stewart, C. S. Wang and H. Shao, Complex coacervates as a foundation for synthetic underwater adhesives, Adv. Colloid Interface Sci., 2011, 167(1–2), 85–93 CrossRef CAS PubMed
.
- Y. Sun, S. Y. Lau, Z. W. Lim, S. C. Chang, F. Ghadessy, A. Partridge and A. Miserez, Phase-separating
peptides for direct cytosolic delivery and redox-activated release of macromolecular therapeutics, Nat. Chem., 2022, 14(3), 274–283 CrossRef CAS PubMed
.
- A. D. Malay, T. Suzuki, T. Katashima, N. Kono, K. Arakawa and K. Numata, Spider silk self-assembly via modular liquid-liquid phase separation and nanofibrillation, Sci. Adv., 2020, 6(45), eabb6030 CrossRef CAS PubMed
.
- R. Balu, N. K. Dutta, A. K. Dutta and N. R. Choudhury, Resilin-mimetics as a smart biomaterial platform for biomedical applications, Nat. Commun., 2021, 12(1), 149 CrossRef CAS PubMed
.
- F. G. Quiroz and A. Chilkoti, Sequence heuristics to encode phase behaviour in intrinsically disordered protein polymers, Nat. Mater., 2015, 14(11), 1164–1171 CrossRef CAS PubMed
.
- M. Abbas, W. P. Lipiński, K. K. Nakashima, W. T. S. Huck and E. Spruijt, A short peptide synthon for liquid–liquid phase separation, Nat. Chem., 2021, 13(11), 1046–1054 CrossRef CAS PubMed
.
- S. Yang, H. Yu, X. Xu, T. Yang, Y. Wei, R. Zan, X. Zhang, Q. Ma, H. C. Shum and Y. Song, AIEgen-Conjugated Phase-Separating Peptides Illuminate Intracellular RNA through Coacervation-Induced Emission, ACS Nano, 2023, 17(9), 8195–8203 CrossRef CAS PubMed
.
- K. Jaqaman and J. A. Ditlev, Biomolecular condensates in membrane receptor signaling, Curr. Opin. Cell Biol., 2021, 69, 48–54 CrossRef CAS PubMed
.
- S. F. Banani, H. O. Lee, A. A. Hyman and M. K. Rosen, Biomolecular condensates: organizers of cellular biochemistry, Nat. Rev. Mol. Cell Biol., 2017, 18(5), 285–298 CrossRef CAS PubMed
.
- P. Zhao, B. Yang, X. Xu, N. C. Lai, R. Li, X. Yang and L. Bian, Nanoparticle-assembled vacuolated coacervates control macromolecule spatiotemporal distribution to provide a stable segregated cell microenvironment, Nat. Commun., 2021, 12, 7162 CrossRef CAS PubMed
.
- P. Zhao, X. Xia, X. Xu, K. K. C. Leung, A. Rai, Y. Deng, B. Yang, H. Lai, X. Peng, P. Shi and H. Zhang, Nanoparticle-assembled bioadhesive coacervate coating with prolonged gastrointestinal retention for inflammatory bowel disease therapy, Nat. Commun., 2021, 12(1), 7162 CrossRef CAS PubMed
.
- N. T. Qazvini, S. Bolisetty, J. Adamcik and R. Mezzenga, Self-healing fish gelatin/sodium montmorillonite biohybrid coacervates: structural and rheological characterization, Biomacromolecules, 2012, 13(7), 2136–2147 CrossRef CAS PubMed
.
- C. Forenzo and J. Larsen, Complex Coacervates as a Promising Vehicle for mRNA Delivery: A Comprehensive Review of Recent Advances and Challenges, Mol. Pharm., 2023, 20(9), 4387–4403 CrossRef CAS PubMed
.
- S. Liu, Y. Zhang, M. Li, X. Li, Z. Zhang, X. Yang, X. He, K. Wang, J. Liu and S. Mann, Enzyme-mediated nitric oxide production in vasoactive erythrocyte membrane-enclosed coacervate protocells, Nat. Chem., 2020, 12, 1165–1173 CrossRef CAS PubMed
.
|
This journal is © The Royal Society of Chemistry 2024 |