DOI:
10.1039/D3VA00194F
(Critical Review)
Environ. Sci.: Adv., 2023,
2, 1488-1504
Promising approaches and kinetic prospects of the microbial degradation of pharmaceutical contaminants
Received
14th July 2023
, Accepted 25th September 2023
First published on 12th October 2023
Abstract
Pharmaceutical pollutants are released into the environment due to their direct outflow from waste disposal, animal discharge, and drug manufacturing. The long-term health effects on humans and animals due to their biological activity are the negative impacts of pharmaceutical pollutants. Microbial degradation is an effective remediation strategy for removing harmful contaminants from contaminated zones by breaking down foreign substances into smaller useable materials. The novel aspect of the review deals with the advancements and kinetic prospects of the microbial degradation of pharmaceutical pollutants. This review illustrates the classifications, toxic effects on health, occurrences and sources of pharmaceutical pollutants. The interaction mechanism between microbes and pollutants and the molecular mechanism under aerobic and anaerobic conditions are clearly demonstrated in this review. This review discusses in depth the advancements in the field of microbial degradation, such as the utilization of genetically engineered microbes and enzyme immobilization techniques for enhancing the degradation of pollutants. The purpose of this review is to describe the microbial degradation kinetics in order to efficiently supervise the pharmaceutical-contaminated sites. Recent advancements and future prospects for the effective removal of pharmaceutical contaminants are also discussed in depth.
Environmental significance
Environmental significance statement for the paper “Promising approaches and kinetic prospects of the microbial degradation of pharmaceutical contaminants.” 1. What is the problem/situation? The release of pharmaceutical pollutants has increased worldwide through improper disposal of medicines, drugs and other used compounds from drug manufacturing units. This hinders the biological activity of many living organisms and has a long-term effect on the ecosystem. 2. Why is it important to address/understand this? The remediation of pharmaceutical contaminants is essential for mitigating the negative effects caused by the compounds in the ecological system. Microbial degradation is considered to be one of the effective remediation strategies due to its lack of toxic byproduct release during the process. Micro-organisms have the ability to degrade complex pharmaceutical compounds into simpler substances in the presence of enzymes. Hence, a clear understanding of the mechanisms and advancements in microbe-based degradation of pharmaceutical pollutants is essential for effectively addressing the pollution problems. 3. What is the key finding and what are the implications of this in relation to 1 and 2 above. The molecular mechanism in the microbial degradation of pharmaceutical pollutants is one of the key findings in this review. The interaction of micro-organisms with pollutants adds on for the better understanding of the degradation process. The factors that need to be optimized during the microbial degradation process have been discussed in detail, of which the type of microbial inoculum, pH, and temperature are crucial for better degradation. Advances such as genetic engineering and immobilization enable the complete degradation of pharmaceutical compounds and also inhibit the release of toxic compounds.
|
1. Introduction
Humans have created a plethora of synthetic compounds for use in a variety of sectors. Some new pollutants have a significant impact on the ecology, as they are not effectively monitored prior to disposal. Pharmaceuticals play a critical role in improving the quality and expectancy of life of people across the globe. Several medications are used each year to treat infections, illnesses, and other health issues.1 Antibiotic consumption is remarkably high in developing countries, such as India, Russia, and South Africa. Following treatment processes, these residual pharmaceutical substances are released into the environment, causing major medical risks. Pharmaceutical chemicals are classified as emerging pollutants due to their continued use, uncertain environmental and health impacts, and resistance to complete degradation. Pharmaceuticals have been found in surface and ground water at concentrations of parts per trillion and billion.2 Most medications are partially metabolised by organisms into inactive forms. Approximately 75% of antibiotics are excreted as active metabolites. Nonsteroidal medications are highly water-soluble chemicals that are partially destroyed and have a negative influence on the environment.3,4
Extensive pharmaceutical distribution is a major concern as it poses serious threats worldwide.5 Active chemicals, such as hormone receptors are detrimental to marine organisms in the setting of an aquatic system. To control the hazardous pharmaceutical pollution, certain discharge regulations are required under standard settings. High aqueous-based discharges must be closely monitored and recognised in order to hypothesise on the best ways for removing and degrading pharmaceutical compounds from water sources. Apart from these, pharmaceutical contaminants also come in the form of agricultural runoff, which might include pesticides.6–8
Pharmaceutical pollutants, including high COD concentrations, vary depending on industrial discharge. Nitrogen usage in antibiotic manufacturing increases nitrogen concentrations, but no legally controlled legislation or system is in place to mitigate these pollutants.9,10 In general, chlorination is the most commonly used procedure for purifying drinking water. Overall, physicochemical methods are used first, followed by secondary treatment processes involving biological reactions. Adsorption is favoured in fundamental physicochemical processes for the elimination of some medicinal substances, and organic and inorganic pollutants. Advanced oxidation and precipitation procedures can be used to eliminate bio-organic substances. However, certain residual medications such as ibuprofen, iopromide, and sulfamethoxazole are still present in the effluent and must be treated using subsequent biological processes.11,12
Microbial species are important xenobiotic degraders that aid in the maintenance of correct ecological balance. Microbial degradation is a highly effective approach for removing dangerous pharmaceutical pollutants from the environment (Ramesh et al., 2023). The pharmaceutical breakdown rates are primarily determined by factors such as the composition of the microbial culture, medicines, pH, and temperature. Microorganisms play an important role in the environment via several biodegradation pathways involving enzymes, metabolites, and co-metabolites.13–15 Furthermore, the use of microbial breakdown mechanisms can greatly lower the toxicity of active medicinal compounds. In nature, the interaction between microorganisms and drugs is non-inhibitory. Basic molecular biology and engineering principles are used in preliminary procedures that provide an imperfect solution to metabolic mechanisms.16
Recent innovations in genetic engineering domains, such as recombinant techniques, allow for a shift in practical applications. Microbial systems are built in such a way that enzyme switching to mineralization of medicinal substances is possible. Synthetic biology and system biology, two topics related to systemic biology, aid in reducing the obstacles and drawbacks connected with earlier conventional microbial degradation processes.17,18 The principles of sustainable metabolic and synthetic engineering help maximise the sustainability of pharmaceutical degradation. Current studies have resulted in the successful rebuilding of metabolic pathways. Another recent breakthrough is the immobilisation of microbial cells in an appropriate carrier material. The immobilisation of biodegrading organisms in biofilm systems has advantages in terms of ease of use, operation, and economics. Enzymes or bacteria/fungi with degrading capacity can be immobilised in an intact system with an inert carrier material. As a result, microbial immobilisation contributes to being a prospective candidate in recent improvements. Different kinetic models can be used to predict the mechanism of biodegradation.19–21 The literature previously has not focused on the recent advancements with the kinetic aspects of the microbial mediated remediation of pharmaceutical pollutants. This review article specifically focusses on the kinetic prospects and advances like metabolic or genetic engineering with immobilization for the microbial degradation of pharmaceuticals.
The primary concern of the review has been on the mechanisms and advances in the microbial degradation of pharmaceuticals. The paper provides a comprehensive summary of several pharmaceutical pollutant groups, their sources, and the accompanying health impacts. The interaction of microorganisms and pharmaceutical pollutants, as well as their chemical mechanism, has been described. The review discusses the recent advances in the microbial realm, such as metabolic engineering and cell immobilisation. The description of the degradation kinetic analysis aids in the determination of the mechanisms involved in pharmaceutical contaminant elimination.
2. Pharmaceutical pollutants
2.1 Classifications
Antivirals, anti-inflammatories, anti-convulsants, antibiotics, and analgesics are the major prevalent pharmaceutical pollutants. Table 1 lists the classification and characteristics of pharmaceutical pollutants.22–45 Antiviral medications are used to treat viral infections such as influenza, hepatitis, polio, measles, and small pox by inhibiting the pathogen growth. Antiviral medicines are more active in nature during viral propagation. Antiviral medications impede viral attachment entry into the cell, nucleic acid synthesis with protein synthesis, and eventually packaging and vital release into the environment. Amantadine, gancyclovir, zidovudine, nevirapine, and emtricitabine are examples of common antiviral medicines.46,47 Antiviral medications are commonly found in aquatic systems such as wastewater, effluents, surface water, and ground water. Antibiotics are additional developing pharmaceutical contaminants that are widely used in the veterinary and health industries. Antibiotics are medication classes used to treat bacterial illnesses in humans and animals by inhibiting growth or metabolism and killing the bacterium. Antibiotics are primarily produced by microorganisms to accomplish a variety of activities. They can function as predators with an attacking mechanism or as a chemical weapon with a defensive mechanism.48,49 The first antibiotics are penicillin compounds produced from organic components via chemical synthesis or modification. Antibiotics are further categorised as bacteriostatic or bactericidal based on their method of action.50
Table 1 Classification and characteristics of pharmaceutical pollutants
S. no. |
Classification of pharmaceutical |
Pharmaceutical compounds |
Chemical formula |
CAS ID |
Molar mass (g mol−1) |
Boiling point (°C) |
Melting point (°C) |
pKa |
References |
1. |
Antivirals |
Acyclovir |
C8H11N5O3 |
59277-89-3 |
225.21 |
595 |
256.6 |
2.52 |
22
|
Adefovir |
C8H12N5O4P |
142340-99-6 |
273.186 |
632.5 |
102 |
1.35 |
23
|
Amantadine |
C10H17N |
665-66-7 |
151.25 |
360 |
180 |
10.1 |
24
|
2. |
Non-steroidal anti-inflammatory drug |
Aspirin |
C9H8O4 |
50-78-2 |
180.158 |
140 |
136 |
2.97 |
25
|
Ibuprofen |
C13H18O2 |
15687-27-1 |
206.29 |
157 |
75–77 |
5.2 |
26
|
Naproxen |
C14H13NaO3 |
22204-53-1 |
230.26 |
403.9 |
153 |
4.2 |
27
|
Diclofenac |
C14H11Cl2NO2 |
15307-86-5 |
296.148 |
412 |
302–310 |
4 |
28
|
Celecoxib |
C17H14F3N3O2S |
169590-42-5 |
381.373 |
529 |
161–164 |
11.1 |
29
|
Etoricoxib |
C18H15ClN2O2S |
202409-33-4 |
358.842 |
510 |
135–137 |
4.5 |
30
|
3. |
Anti-convulsant |
Pregabalin |
C8H17NO2 |
148553-50-8 |
159.23 |
85 |
196 |
4.2 |
31
|
Phenytoin |
C15H12N2O2 |
57-41-0 |
252.268 |
464 |
298 |
2.3, 8.3 |
32
|
Ethosuximide |
C7H11NO2 |
77-67-8 |
141.168 |
265.3 |
64.5 |
8.2 |
33
|
Topiramate |
C12H21NO8S |
97240-79-4 |
339.363 |
438.7 |
125 |
1.4, 4.3 |
34
|
4. |
Antibiotics |
Erythromycin |
C37H67NO13 |
114-07-8 |
733.93 |
818.4 |
135–140 |
8.88 |
35
|
Sulfamethoxazole |
C10H11N3O3S |
723-46-6 |
253.279 |
482.1 |
169 |
3.92 |
36
|
Azithromycin |
C38H72N2O12 |
83905-01-5 |
785 |
822.1 |
129–135 |
8.5 |
37
|
Trimethoprim |
C14H18N4O3 |
738-70-5 |
290.32 |
405.2 |
199–203 |
7.1 |
38
|
Levofloxacin |
C18H20FN3O4 |
100986-85-4 |
361.368 |
571.5 |
213–218 |
5.35 |
39
|
Cephalexin |
C16H17N3O4S |
15686-71-2 |
347.39 |
727.4 |
326.8 |
3.45 |
40
|
5. |
Analgesics |
Codeine |
C18H21NO3 |
76-57-3 |
299.364 |
462 |
154 |
8.2 |
41
|
Fentanyl |
C22H28N2O |
437-38-7 |
336.471 |
466 |
87.5 |
8.05 |
42
|
Hydrocodone |
C18H21NO3 |
125-29-1 |
299.368 |
65 |
118–128 |
8.9 |
43
|
Meperidine |
C15H21NO2 |
57-42-1 |
247.33 |
328.9 |
186–189 |
8.63 |
44
|
Methadone |
C21H27NO |
76-99-3 |
309.445 |
423.7 |
235.54 |
9.2 |
45
|
Non-steroidal anti-inflammatory medications are a large class of therapeutics with a vast functional variety that is used to alleviate pain and inflammation. Aromatic groups with acidic functional moieties are common in anti-inflammatory medicines.51 A broad classification based on chemical forms includes oxicams, salicylates, acid, indole derivatives, and anthranilates. The majority of anti-inflammatory medications are attached to plasma proteins, which improves the bioavailability by allowing them to cross the organ barrier. Anti-inflammatory medications have notable effects such as tumour cell induction, DNA damage protection, and neogenesis inhibition.52
Anticonvulsants, frequently referred to as antiseizure or antiepileptic medications, have the ability to regulate convulsions caused by electrical activity in the brain.53 Antiepileptic medications give the necessary seizure relief. Phenytoin, benzodiazepine, primidone, and phenobarbital are examples of common anticonvulsants. More than 30% of persons are resistant to anticonvulsant medications that result in significant side effects during seizure management. Hormones are the most common drug classes in the pharmaceutical industry. Underactive hormone secretion will be treated with synthetic hormone replacement therapy.54,55 Other pharmaceutical pollutants found in the environment include antidepressants and antipyretics. Certain classes of pesticides also come under such disinfectants, and wormicides also come under pharmaceutical class of contaminants. Fig. 1 represents the classification and health effects of pharmaceutical pollutants.
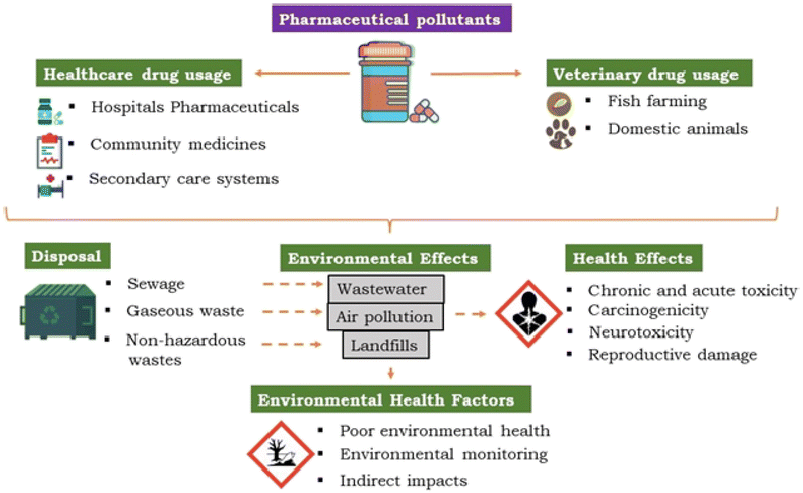 |
| Fig. 1 Classification and health effects of pharmaceutical pollutants. | |
2.2 Health effects
Pharmaceuticals react biologically differently to various particular and non-specific species. Pharmaceuticals with low concentrations can be found in the environment for extended periods of time. The bioavailability of pharmaceuticals and their associated health ailments are tabulated in Table 2.56–67 The toxic effects on aquatic organisms exposed to pharmaceutical contaminants alter their lifetime. Analgesic exposure in the environment causes kidney diseases and morphological abnormalities in the gills. Pollutants at 1000–3000 g L−1 concentrations might cause acute renal failures with changes in foetal anomalies.68,69 Fish are noteworthy organisms harmed by excessive pharmacological concentrations. Pharmaceutical pollutants cause structural disturbance with changes in gene expression and the reproductive system, which are the major governing mechanisms. Aside from fish, several algae are vulnerable to the negative effects of medications.70 In plants, fatty acid production is a critical step in the photosynthetic process. Chronic toxicity in the photosynthetic machinery of both algae and plants has been found, impairing chloroplast function. Because of their active nature, hormones, even at low doses, can pose substantial health risks. This causes considerable endocrine disturbance in fish, resulting in estrogenic effects. Cyanobacteria and algae have a higher histopathological index and are more antibiotic resistant.71,72 When exposed to tetracycline chemicals, oxidative DNA damage with metal complex formation has been reported. Atorvastatin exposure had a significant impact on lipid regulators with beta blockers in both target and non-target organisms. Because of their high sorption nature and affinity in sediments, enzymes involved in the beta receptor activity represent a risk to aquatic animals. Neurotoxicity is the most common health problem associated with psychiatric medicines in effluents or municipal wastewater systems. Anticancer drugs at concentrations ranging from 0.1 to 0.3 mg L−1 have an effect on optical acuity and mutagenicity. Endocrine disruptors induce reproductive and digestive system disruption. Males experience hypogonadism as a result of ibuprofen contraindications.73–75 These pesticide compounds may enter into water systems through surface run-off or leaching. Indirect toxic effects on the fishes with impairment in the metabolic system of primary producers are few ailments related to aquatic species. Hormonal imbalance, neurological dysfunction, blood disorders, and immune impairment are the known effects of pesticides in the health system.76 As a result, pharmaceutical exposure leads to expanding health and environmental problems.
Table 2 Bioavailability of the pharmaceutical and their associated health ailments
S. no. |
Pharmaceuticals |
ChEMBL Id |
Bioavailability |
Administrative route |
Dosage |
Health ailments |
References |
1. |
Acetaminophen |
112 |
70–90% |
Oral, rectal, and intravenous |
150 mg kg−1 |
Abdominal pain, diarrhea, irritability, and vomiting |
56
|
2. |
Cimetidine |
30 |
60% |
Oral, and intravenous |
400 mg/per day |
Reversible impotence or gynecomastia |
57
|
3. |
Digoxigenin |
1153 |
70–80% |
Oral |
0.25 mg/per day |
Ventricular arrhythmias, hypotension, symptomatic bradycardia |
58
|
4. |
Carbamazepine |
108 |
75–85% |
Oral |
200 mg/per day |
Coma, imbalance, dizziness, and drowsiness |
59
|
5. |
Warfarin |
1464 |
100% |
Oral |
7.5 mg/per day |
Joint pain, bleeding, vision change, significant hemorrhage |
60
|
6. |
Albuterol |
714 |
21–27% |
Inhalation route |
4 mg/3–4 times a day |
Cough, throat irritation, vomiting |
61
|
7. |
Ciprofloxacin |
8 |
70% |
Oral, and intravenous |
1000 mg/per day |
Tendinitis, tendon rupture |
62
|
8. |
Codeine |
485 |
60% |
Oral |
240 mg/per day |
Acute pancreatitis, liver damage |
63
|
9. |
Dehydro nifedipine |
193 |
45–68% |
Oral, and intravenous |
120 mg/per day |
Flushing, peripheral edema, dizziness, and headache |
64
|
10. |
Diltiazem |
23 |
40% |
Oral |
240 mg/per day |
Headache, allergic reactions |
65
|
11. |
Doxycycline |
1433 |
73–95% |
Oral |
200 mg/per day |
Bloating, blistering, decreased appetite |
66
|
12. |
Metformin |
1431 |
40–60% |
Oral |
500 mg/per day |
Lactic acidosis |
67
|
2.3 Sources and occurrence
Without suitable treatment techniques, pharmaceutical chemicals are widely discharged into the environment. Pharmaceuticals are also used in different agricultural elements for disease prevention and treatment. Pharmaceutical use has increased in recent years due to its physicochemical and biochemical action modes. Certain molecules are metabolised during drug delivery, while others stay intact prior to elimination. Because of their low volatile and highly polar character, these metabolites remain stern and are not excreted into systems or disposed of in waste effluents. Pharmaceutical contaminants are unlikely to enter the environment via industrial disposal channels.77,78 Pharmaceutical chemicals are classified into two types: point sources and non-point sources. Pharmaceuticals are most typically introduced into the environment by sewage sludge dumping, groundwater leaching, and surface run off. Medical chemicals have been found in municipal and hospital effluent sewage. Hospital sludge has a higher concentration than that of municipal sludge. Expired medications are dumped in home sewage. Pharmaceuticals are also present in landfills; however, they are less concentrated due to their sorption or breakdown capabilities. Primary and secondary point sources of pharmaceutical pollution are landfills and wastewater. Sewage treatment plants contain considerable amounts of medicines that remain unmetabolized for extended periods of time.79 Duan et al. (2021) investigated the occurrence and source analysis of pharmaceutically active components in China City's aquatic systems. Diclofenac acid, carbamazepine, and caffeine are the most commonly detected substances. Besides, high lincomycin concentrations (81.1 ng L−1) are frequent in the antibiotic class. Other medications detected in average concentrations (16 to 21 ng L−1) include sulfamethoxazole, roxithromycin, and erythromycin.80,81 The investigation also revealed that the target compounds were detected in low amounts and that non-antibiotics were more frequent in the ecosystems.
3. Microbial degradation
3.1 Molecular mechanism and interaction of microbes with pharmaceutical pollutants
Many organic and inorganic compounds are degraded significantly by microorganisms. Microbial systems play a significant role in ecosystem functioning since they are a key community. When medicines enter the ecosystem, many processes such as sorption, hydrolysis, and biodegradation occur, which prevents ecotoxicity in environmental systems. Microbial degradation is the best strategy in the environmental aspect for effective harmful pollutant removal.82 Natural creatures in water and soil are significant players in environmental process management. They also control the release of drugs into the environment. Microbial species participate in the degradation and purification processes via metabolic and co-metabolic pathways. The two primary biodegradation pathways are bio-oxidation and hydrolysis. The enzymes involved in metabolic and co-metabolic pathways aid in pharmacological transformation.83Fig. 2 depicts the interaction mechanism between microbes and pharmaceutical pollutants.
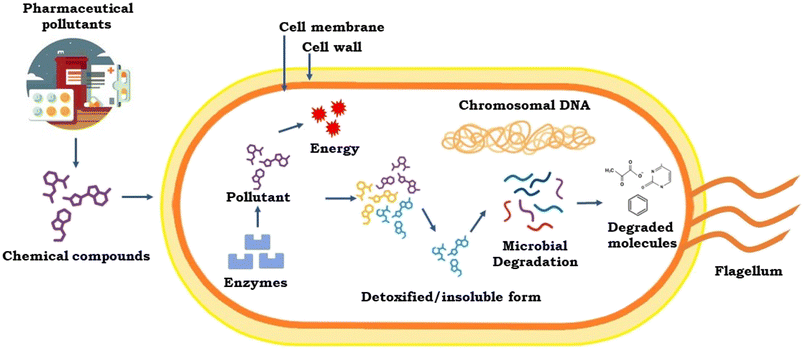 |
| Fig. 2 Interaction mechanism between microbes and pharmaceutical pollutants. | |
Microbial biodegradation can be categorised into three stages: bioattenuation, biostimulation, and bioaugmentation.84 Bioattenuation is the inclusiveness of organisms to improve metabolic activity and pharmaceutical breakdown. Limited nutrients are added to enable the microbial species to degrade harmful contaminants. Supplementary organisms are added to microflora for specific impurities during the bioaugmentation process. By metabolising pollutants, microorganisms use pharmaceutical contaminants as their sole source of growth. Pharmaceutical contaminants can be completely mineralized during the biodegradation process.85,86 The biodegradation of pharmaceuticals has the benefit of both aerobic and anaerobic mineralization. In terms of bacteria-based biodegradation, a mixed bacterial consortium is favoured since it has a variety of enzymes capable of degrading the chemicals. Several investigations have been conducted on the breakdown of particular medicinal components by specific bacterial species. In one investigation, the Achromobacter sp. destroyed sulfamethoxazole. Pharmaceutical wastewater sediments were used to isolate the bacterial species. The bacterial culture must first adjust to its new habitat before beginning the degradation process. For strain growth, sulfamethoxazole was used as an electron donor and carbon source.87,88
Diclofenac was also subjected to biodegradation by Labrys portucalensis sp. in another investigation. This mechanism was primarily identified by the detection of metabolites. The reaction begins with hydroxylation reactions, which result in isomer formation. The hydroxylation reaction takes place using mono and dioxygenase enzymes released by Labrys sp. as the first step in the microbial degradation process. The enzyme methyl transferase adds a methyl group to 4-hydroxy diclofenac molecules. The decarboxylase enzymes then participate in the intermediate process. The auto-oxidation of hydroxylated metabolites occurs via an alternative pathway, resulting in benzoquinone molecules. Diol dehydrogenases fission rings, resulting in Kreb's cycle intermediates and simpler metabolite molecules.89 After 24 hours of treatment, diclofenac had a comparable response to Chlamydomonas reinhardtii. The concentration of metabolites was also observed to rise with the cell biomass.90
Other microbial species that participate in the pharmaceutical breakdown process include fungi. Pharmaceutical substances are broken down by extracellular multi-enzyme complexes. Olicon-Hernandez et al. (2019)91 investigated ascomycete fungus species' degradation of diclofenac compounds. Penicillium oxalicum was isolated from hydrocarbon-polluted materials and employed in flask and bench scale reactors for biodegradation. For free Penicillium sp., the clearance rate was greater than 99%. During biodegradation mechanisms, hydroxylated metabolites linked with phase 1 and phase 2 detoxification pathways are formed concurrently. The inclusion of transferase enzyme in the process mechanism facilitates the formation of high conjugate metabolites – diclofenac acyl glucuronide.91Trametes pubescens was used to breakdown clofibric acid, another pharmaceutically active molecule. Trametes sp. consumes clofibric acid as a substrate, which has both antagonistic and synergistic effects. Trametes, a white rot fungal, undergoes oxidation followed by the production of metabolite intermediates. The fungal-based microbial degradation reached around 30% degradation.92 In the microbial degradation of pesticides, pesticides are utilized as microbial nutrient sources, which are consequently degraded into small compounds. Mechanisms involved in the degradable pathways are hydrolysis, reduction, decarboxylation, condensation, dehydrogenation, and oxidation. Initially, adsorption of pesticides occurs on the cell membrane surface. Second, mineralization of the organic compounds to simple inorganic compounds takes place under the influence of enzymatic action. In addition, microbial pesticide degradation includes co-metabolism as a primary mechanism.93,94 Chen et al.93 explored the metabolic pathway for the chloracetamide degradation by microbial species. Different biological and physiological reactions such as hydroxylation, dechlorination, and dealkylation have been observed in the microbial degradation of chloracetamide pesticides.93 Different microbial remediation studies on the removal efficiency of pharmaceutical pollutants are listed in Table 3.95–119
Table 3 Microbial remediation studies on the removal efficiency of pharmaceutical pollutants
S. no. |
Microbes |
Pharmaceuticals |
Operating condition |
Technique used |
Efficiency |
Time |
References |
Bacteria |
1. |
Geobacter, and Acetoanaerobium |
Erythromycin |
500 mV, 27 °C |
SEM |
99% |
4 days |
95
|
2. |
Bacillus cereus H38 |
Sulfamethazine |
25 °C, pH 7.0, and inoculation amount 5% |
LCMS, whole genome sequencing |
100% |
3 days |
96
|
3. |
Pseudomonas stutzeri, and Shewanella putrefaciens |
Sulfamethoxazole |
30 ± 1 °C, 180 rpm, 24 h |
HPLC-MS |
61.79%, and 68.67% |
5 days |
97
|
4. |
Delftia lacustris
|
Erythromycin |
37 °C, 180 rpm, 18 h |
— |
45.18% |
5 days |
98
|
5. |
Providencia stuartii
|
Tylosin |
15 h, 40 °C, pH 7.0, and 1% (v/v) inoculation |
Whole-genome sequencing |
100% |
3.5 days |
99
|
6. |
Sphingomonas sp. |
Thiamphenicol |
25 °C, 150 rpm |
Proteomic and metabolomic analysis |
93.9% |
1.5 days |
100
|
7. |
Bacillus clausii
|
Cefuroxime, cefotaxime, and cefpirome mixture |
37 °C, 220 rpm |
Uv-spec, RT-PCR |
100% |
0.5 day |
101
|
8. |
Klebsiella sp. |
Tetracycline |
25 °C, 5 min, 630 W |
UPLC-Q-TOF, UPLC-UV |
58.64% |
6 days |
102
|
9. |
Pseudomonas aeruginosa
|
Dicloxacillin |
30 °C, 2 h |
UV, PCR, HPLC |
100% |
2.2 days |
103
|
10. |
Micrococcus luteus N.ISM.1 |
Gabapentin |
35 °C, 120 rpm, 72 h |
FE-SEM, LC-ESI-MS |
47% |
40 days |
104
|
11. |
Klebsiella sp. |
Diclofenac sodium |
30 °C, 120 rpm |
LCMS |
79.14% |
3 days |
105
|
Fungus |
12. |
Lentinula edodes and Imleria badia |
Cephalosporin |
Ultrasound at 49 kHz, 20 min |
UPLC/MS |
100% |
7 days |
106
|
13. |
Leptosphaerulina sp. |
Oxacillin |
28 °C, pH 5.6, and 160 rpm for 15 days |
Biotransformation and MTT assay |
100% |
6 days |
107
|
14. |
L. edodes
|
Testosterone |
25 °C ± 2 °C, 140 rpm |
UPLC |
100% |
21 days |
108
|
15. |
L. edodes
|
17α-Ethinylestradiol |
25 °C ± 2 °C, 100 rpm |
HPLC, UV-Spec |
100% |
21 days |
109
|
16. |
Penicillium oxalicum
|
Diclofenac |
28 °C, 120 rpm |
HPLC, UPLC |
100% |
1 day |
110
|
17. |
Penicillium commune
|
Oxytetracycline |
27 °C, pH 7.3 |
Optical and scanning electron microscopy |
78% |
15 days |
111
|
18. |
Penicillium oxalicum RJJ-2 |
Erythromycin |
35 °C, pH 6.0, 96 h |
HPLC-MS |
84% |
4 days |
112
|
19. |
Trichoderma citrinoviride AJAC3 |
17-β-estradiol (E2) |
28 °C ± 2 °C, 120 rpm |
HPLC, GCMS, SEM, FTIR |
100% |
4 days |
113
|
20. |
Trichoderma sp. |
Ciprofloxacin |
25 °C, 135 rpm |
LCMS |
81% |
13 days |
114
|
21. |
Phanerochaete chrysosporium
|
Sulfadiazine |
30 °C, pH 5.7 |
Q-Exactive-MS, RNA sequencing |
100% |
6 days |
115
|
22. |
Trametes versicolor
|
Diclofenac |
4 pH, 1 mL min−1 flow, 33 h (hydraulic retention time) |
Q-TOF LC/MS |
98% |
7 days |
116
|
Algea |
23. |
Chlorella sp. |
Florfenicol |
5000 ± 100 lux illumination intensity, 30 ± 1 °C |
HPLC-UV |
97% |
7 days |
117
|
24. |
Chlorella sp. |
Thiamphenicol |
4 °C, 5000 rpm, 10 min |
Uv, HPLC |
97% |
5 days |
118
|
Microbial consortium—bacteria, fungus and yeast |
25. |
Klebsiella pneumoniae, Achromobacter sp., Candida manassasensis, Trichosporon asahii |
Ofloxacin, and norfloxacin |
30 °C, 120 rpm, 48 h |
PCR |
80% |
20 days |
119
|
3.2 Influencing factors
Certain biotic and abiotic variables generally influence the biodegradation process. In terms of microbial degradation, the factors associated with microbial growth and processing are growth source, temperature, pH, and nutrient source. Besides, pharmaceutical features and microbial culture incubation with medicines have an important influence on the degradation process.120
pH is a vital component in the microbe-based degradation process because it influences enzymatic activity, proliferation, adaptability, shape, and certain membrane properties. The acidic and alkaline pH values are intimately related to the ionic form of medicinal substances, which influences the degradation process indirectly.121 In a study of ibuprofen degradation by Bacillus thuringiensis, it was discovered that a pH in the range of 6 to 7 was optimal for maximum ibuprofen degradation. The bacterial enzymes hydroquinone monooxygenase and hydroxyquinol 1,2-dioxygenase are most active at pH levels between 7 and 8, which may have altered the breakdown process. The uncharged form of ibuprofen interacts with the bacterial cell surface releasing enzymes, leading to disintegration.122
Temperature is an essential factor that influences the microbial breakdown process. The chemical reaction doubles or rises four times for every 10 °C temperature increase. The temperature is directly related to the operation of cell enzymes and cell membranes. High temperatures cause protein denaturation, whereas low temperatures affect the cell viscosity and stiffness, limiting the enzyme performance. Temperature has also been shown to have a considerable impact on the metabolic development and activity of cultures.122–124 In a study by Yu et al.,125 the effect of different parameters on sulfamethoxazole degradation by Pseudomonas koreensis and Paenarthrobacter ureafaciens was analysed. The effects of temperatures ranging from 10 to 50 °C have been investigated. The bacterial consortium grew better at temperatures ranging from 20 to 40 degrees Celsius. The ideal temperature for maximum sulfamethoxazole was found to be 30 °C. Microbial development was not possible at low temperatures. Similarly, no pharmacological decrease was observed at high temperatures.125
Microbial inoculum is a key aspect in the degradation of medicinal substances. When the inoculum is large, it can occasionally hinder the degrading capacity due to poisonous material release. The ageing of the inoculum affects the organism's ability to degrade.126 Yang et al.97 studied the impact of several parameters on the degradation of chloramphenicol by Pseudomonas and Shewanella sp. For maximum breakdown ability, bacterial inoculation amounts of 1, 2, and 3% were changed. The effectiveness of biodegradation increased, as the bacterial count increased from 1% to 2%. Yet, at 3% bacterial concentration, the degradation remains steady without further rise. At a bacterial load of 2%, a maximum of 60–65% degradation efficiency was achieved.98 The nature and characteristics of medicinal compounds play an additional role in biodegradation. The more stable and complex pharmacological substances are more difficult to breakdown.127
4. Recent advancements
4.1 Metabolic or genetic engineering
Genetic and metabolic engineering is a rapidly growing discipline globally for the production of novel microbes with desired features. In general, genetically modified organisms are bacteria that have had their genetic code altered via the recombinant DNA process. Designing genetic and metabolically modified organisms is required for creating new metabolism pathways for pharmaceutical degradation, reducing hazardous metabolite accumulation, increasing metabolic catabolism, and improving organism substrate flux. The introduction of novel genes aids in the adaptation of microorganisms to pharmaceutical pollutants.128,129 The steps involved in the production of metabolically engineered microbes are shown in Fig. 3. The recombinant DNA field enables improved energy generating methods, increased copy number, and inclusion of desired genes. The metabolic engineering method has included novel aspects relevant to limiting factors and pathways. Various genetic methods have been used to reinforce optimal gene expression and change the metabolic pathways of enzymes.130 Some fundamental elements must be present in order to regulate the complicated enzyme system and metabolism. Four parameters must be observed when it comes to the breakdown of pharmaceutical contaminants: enzyme nature, metabolic management of the breakdown pathway, creation with metabolic pathway regulation, and lastly, overall process development for improved genetic modification. Fungal genetic engineering plays a significant role in modifying enzyme targets and affinity for medicinal drugs.131,132
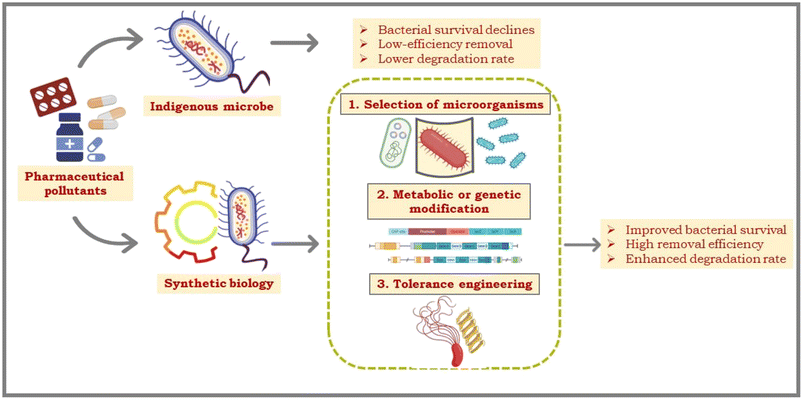 |
| Fig. 3 Steps involved in the production of metabolically engineered microbes. | |
Another new subject that offers potential solutions for pharmaceutical degradation is metabolic engineering. In vitro and in vivo approaches are used to create a synthetic metabolic pathway. In silico technologies can be used to anticipate probable metabolic pathway paths. Another emerging subject is enzyme engineering, which involves the development of novel enzymes with activating/deactivating roles in the metabolic breakdown pathway.133,134 Aulestia et al.135 applied genetic characterisation to determine the metabolic pathway for ibuprofen degradation in a recent research study. Rhizorhabdus wittichii eliminates the pharmaceutical pollutant ibuprofen by the generation of metabolites. Genetic approaches have been used to identify IBU genes with non-colored and leaky mutants. For a better understanding of pathways, molecular biology methods such as mutant insertion and sequence analysis were used.135 A research study employed Escherichia coli metabolic engineering to complete naphthalene breakdown. A total of 17 genes involved in the degradation pathway were optimized and rebuilt. It is possible to recreate the metabolic modules involved in the conversion of naphthalene into catechol and other metabolites. In that study, consecutive naphthalene degradation was achieved by altering the BL-CA-cat genes.136 Similarly, recent studies have focused on the genetic and metabolic alteration of microbial species for improved pharmaceutical contaminant breakdown.
4.2 Immobilization
Enzymes play a crucial role in the microbial breakdown of medications by metabolising the complex compounds. These enzymes can be used directly to degrade the substances. The enzymes can be introduced into the degradation medium as free cells or immobilized. Because enzymes in their free state are difficult to separate, they can be immobilized into solid support materials that only enable the transfer of degrading chemicals into it.137,138 The immobilization procedure will also considerably increase the enzymes' stability and reusability. The two ways for immobilizing enzymes onto solid substrates are physical and chemical. Chemical approaches include cross-linking and covalent bonding, whereas physical methods include encapsulation, entrapment, and adsorption. Physical or ionic attachment of enzymes to solid supports is one way of immobilization via adsorption. Fig. 4 denotes the microbial immobilization for the removal of pharmaceutical pollutants. Silica gel, activated carbon, porous glass, biomass, and other organic porous substances are the commonly utilized support materials.139,140 Because adsorption does not necessitate the use of expensive materials, enzyme or cell structure will not be altered, resulting in high enzymatic activity. Encapsulation encompasses immobilizing enzymes in a spherical shaped non-permeable membrane, whereas entrapment involves enclosing enzymes or cells in a closed fiber-like network. Because physical trapping of enzymes is sometimes ineffective, resulting in enzyme leakage, chemical methods of immobilization are used. Partially deformed enzyme structures and molecular networks produce a stable and strong bond with the support matrix. Chemical immobilisation techniques such as covalent, disulphide, and ionic bonding allow many treatment and reuse cycles.141–143
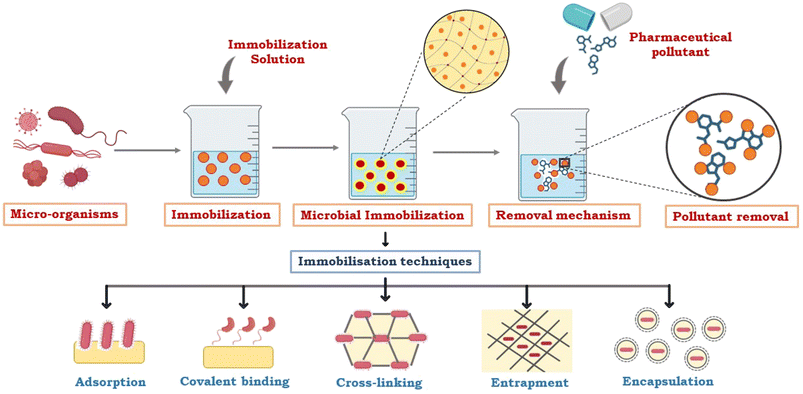 |
| Fig. 4 Microbial immobilization for the removal of pharmaceutical pollutants. | |
Cross linking is another type of immobilisation in which enzymes can be immobilised as crystals or aggregates. Primozic et al.144 immobilised a laccase enzyme as a cross-linked aggregate for diclofenac biodegradation. Laccase precipitation in propanol and aminosilane magnetic nanoparticle solutions was used to form the aggregates. The degradation process was carried out in a stirred batch reactor. The ability to remove diclofenac was approximately 13–16 g diclofenac/g laccase. Even after the fourth regeneration cycle, the immobilised enzyme's half-life remained constant. Immobilised laccases also exhibit good stability.144 Laccase was immobilised in a polyvinylidene fluoride nanocomposite and nanotube containing diclofenac and carbamazepine for breakdown in another recent investigation by Masjoudi et al.141 Initially, phase inversion was used to create multiwalled carbon nanotubes using polyvinylidene fluoride nanocomposites. The laccase enzyme was immobilised in the produced samples and used as a catalyst in pharmaceutical degradation. In the membrane reactor, an activity recovery of 38.31% was recorded with immobilised laccase, with a removal efficiency of 27%.141 Whole cells can also be immobilised for pharmacological degradation. Zur et al.145 immobilised the entire Pseudomonas moorei strain in bacterial cellulose for degrading purposes. Immobilisation was performed for 72 hours in order to improve its effectiveness, and it was examined for auto aggregation and co-aggregation capacity. This complete cell immobilised system decomposed around 150 mg L−1 of paracetamol. Hydroquinone 1,2-dioxygenase, deaminase, and acyl amidohydrolase are major enzymes implicated in the paracetamol breakdown route via entire immobilisation.145 As a result, an immobilised enzyme system with reusable capacity can be successfully used for pharmaceutical degradation.
5. Microbial degradation: kinetic models
The kinetics section is critical for determining the breakdown of pharmaceutical contaminants. Microbial degradation kinetics aids in the accurate evaluation of microorganisms and their environments for pollutant degradation. Besides, model building is critical in regulatory considerations for predicting biodegradation at different time intervals. For the microbe-based degradation of pharmaceutical contaminants, many kinetic models have been developed.146–148 One such model for determining degradation kinetics is the Haldane equation. This equation addresses the kinetics of cell development on hazardous substrates. The Haldane equation can be represented as follows:149 | 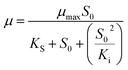 | (1) |
When the inhibition phenomenon occurs, the Haldane equation is more appropriate. The significant parameters are max, KS, and Ki. Certain investigations show that low values of max are due to low biomass concentrations. At high pharmacological concentrations, substrate inhibition can occur, resulting in the incomplete mineralization of intermediates.150 Calero-Diaz et al.151 used kinetic modelling analysis to better understand the biodegradation of ibuprofen, ciprofloxacin, and carbamazepine in a membrane bioreactor system. The following equation was used in the investigation to determine the decay coefficient and kinetics.
| 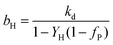 | (2) |
where the decay efficient is represented by
bH and the volatile biomass fraction is denoted by the term 1 −
fP. The addition of pharmaceuticals ibuprofen, ciprofloxacin, and carbamazepine to the membrane bioreactor system resulted in a higher biodegradation rate, which doubled as the cell growth rate increased. With various pharmacological concentrations and an average decay rate of 1–80 g (h
−1 mg
−1), 83–100% elimination was obtained.
151 In addition to the proposed models, the pseudo-first-order kinetic model is a commonly utilised technique of pharmacological degradation. In the first-order kinetics, the reaction rate can be calculated and is proportional to the change in initial pharmaceutical concentration with time. Ciprofloxacin biodegradation has also been studied in another study using
Thermus sp. A bacterium that degrades pharmaceuticals has been identified from the pharmaceutical sludge. The linear relationship between time and diminishing medication concentration can be described as follows:
| ln Ct = −kt + ln C0 | (3) |
The first-order kinetic model agreed well with the experimental data, indicating that microorganisms were responsible for the majority of the deterioration. Sodium acetate increased Thermus sp. biomass production by acting as an electron donor in the non-growth factor metabolism.152 As a result, various kinetic models have been created and confirmed by researchers based on their research.
6. Future outlooks and challenges
One of the key challenges facing pharmaceutical microbial degradation is the lack of standard methodologies for assessing and comparing the degrading ability of different microbial species. Some typical biodegrading analysis methods are inaccurate, which have to be investigated further. The presence of many pollutants in the system reduces the degrading ability. The effect of antibiotics, for example, on the pharmaceutical transport behaviour is critical for successful clearance. This effect's hazy concept needs to be researched further. To anticipate the optimum period for degradation, the microbial species' adaptation time to the pharmaceutical wastewater environment must be well characterised. The mineralization process mostly degrades pharmaceutical substances. However, certain microorganisms biotransform pharmaceutical components, producing intermediates that are more dangerous than the primary pollutants. As a result, a mixed microbial consortium might be used for complete pollutant mineralization rather than a single pure culture, which lacks this potential. The utilisation of extra substrates, which aids in biodegradation via co-metabolic processes, can also improve the biodegradation efficiency. The adoption of optimum statistical approaches can improve the growth conditions of microbial cultures. The incorporation of statistics and bioinformatics fields facilitates process condition optimisation. While the subject of genetic engineering has been developed for the alteration of microbial strains to aid in enhanced biodegradation processes, there is a dearth of research studies focusing on this issue. As a result, the scope of genetic engineering should be properly studied, and pathways should be clarified. To gain a better understanding, various biodegradation routes used by microorganisms must be precisely identified. The reusability of enzymes or entire cells involved in the pharmaceutical degradation process can now be achieved because of the recent advances in immobilisation. However, some mass transfer constraints impact the movement of intermediates and metabolites into and out of the immobilised support system. The discovery of microbial degradation pathways enables modern developments such as metabolic or genetic engineering and immobilisation to significantly increase pharmaceutical biodegradation. A few numbers of kinetic investigations on pharmaceutical biodegradation have been conducted. As a result, additional research incorporating other process parameters is required for a better prediction of degradation rate and kinetic mechanism.
7. Conclusion
Substantial progress in the field of microbial remediation has been the focus in recent years. The presence of microbial communities determines the capacity and efficiency with which medicinal substances can be destroyed. Persistent pharmaceutical pollutants in the environment can be minimised via several metabolic and co-metabolic transformation processes. Hydrolysis and bio-oxidation are the two major degradation pathways for the microbe-mediated pharmaceutical remediation. The use of mixed microbial cultures is desirable for improving THE biodegradation activity. Advanced statistical and bioinformatics tools have been used to optimize the factors impacting the microbial degradation process. The advancement of immobilization techniques with various solid supports improves the reusability of materials used in the remediation processes. The ideal support systems should overcome the mass transfer constraint in the immobilised system. The field of metabolic and genetic engineering is a recent development in the microbial breakdown of pharmaceutical contaminants. Metabolic engineering approaches aid in developing new degradation pathways of metabolism, which enhance the ecological pharmaceutical removal. Of the different kinetic models facilitating the selection of several optimum techniques for polluted sites, the pseudo-first-order model is the best suited due to its better coherence with the process conditions. The future prospects for enzyme engineering improve the aspect of pharmaceutical degradation. Novel in silico techniques in the metabolic engineering field could enhance the contaminant removal from the ecosystem.
Conflicts of interest
There are no conflicts to declare.
References
- Y. Huang, S. F. Chen, W.-J. Chen, X. Zhu, S. Mishra, P. Bhatt and S. Chen, Efficient biodegradation of multiple pyrethroid pesticides by Rhodococcus pyridinivorans strain Y6 and its degradation mechanism, Chem. Eng. J., 2023, 469, 143863, DOI:10.1016/j.cej.2023.143863.
- A. Sengar and A. Vijayanandan, Human health and ecological risk assessment of 98 pharmaceuticals and personal care products (PPCPs) detected in Indian surface and wastewaters, Sci. Total Environ., 2022, 807, 150677, DOI:10.1016/j.scitotenv.2021.150677.
- N. Das, J. Madhavan, A. Selvi and D. Das, An overview of cephalosporin antibiotics as emerging contaminants: a serious environmental concern, Biotech, 2019, 9, 231, DOI:10.1007/s13205-019-1766-9.
- K. Samal, S. Mahapatra and M. H. Ali, Pharmaceutical wastewater as Emerging Contaminants (EC): Treatment technologies, impact on environment and human health, Energy Nexus, 2022, 6, 100076, DOI:10.1016/j.nexus.2022.100076.
- B. K. Zaied, M. Rashid, M. Nasrullah, A. W. Zularisam, D. Pant and L. Singh, A comprehensive review on contaminants removal from pharmaceutical wastewater by electrocoagulation process, Sci. Total Environ., 2020, 726, 138095, DOI:10.1016/j.scitotenv.2020.138095.
- H. K. Khan, M. Y. A. Rehman and R. N. Malik, Fate and toxicity of pharmaceuticals in water environment: An insight on their occurrence in South Asia, J. Environ. Manage., 2020, 271, 111030, DOI:10.1016/j.jenvman.2020.111030.
- W.-J. Chen, W. Zhang, Q. Lei, S. F. Chen, Y. Huang, K. Bhatt, L. Liao and X. Zhou,
Pseudomonas aeruginosa based concurrent degradation of beta-cypermethrin and metabolite 3-phenoxybenzaldehyde, and its bioremediation efficacy in contaminated soils, Environ. Res., 2023, 236, 116619, DOI:10.1016/j.envres.2023.116619.
- P. Bhatt, K. Bhatt, A. Sharma, W. Zhang, S. Mishra and S. Chen, Biotechnological basis of microbial consortia for the removal of pesticides from the environment, Crit. Rev. Biotechnol., 2021, 41, 317–338, DOI:10.1080/07388551.2020.1853032.
- E. S. Okeke, T. P. C. Ezeorba, C. O. Okoye, Y. Chen, G. Mao, W. Feng and X. Wu, Environmental and health impact of unrecovered API from pharmaceutical manufacturing wastes: A review of contemporary treatment, recycling and management strategies, Sustainable Chem. Pharm., 2022, 30, 100865, DOI:10.1016/j.scp.2022.100865.
- D. Lobato-Peralta, E. Duque-Brito, A. Ayala-Cortés, D. M. Arias, A. Longoria, A. K. Cuentas-Gallegos, P. J. Sebastian and P. U. Okoye, Advances in activated carbon modification, surface heteroatom configuration, reactor strategies, and regeneration methods for enhanced wastewater treatment, J. Environ. Chem. Eng., 2021, 9, 105626, DOI:10.1016/j.jece.2021.105626.
- M. A. Tufail, J. Iltaf, T. Zaheer, L. Tariq, M. B. Amir, R. Fatima, A. Asbat, T. Kabeer, M. Fahad, H. Naeem, U. Shoukat, H. Noor, M. Awais, W. Umar and M. Ayyub, Recent advances in bioremediation of heavy metals and persistent organic pollutants: A review, Sci. Total Environ., 2022, 850, 157961, DOI:10.1016/j.scitotenv.2022.157961.
- P. R. Rout, T. C. Zhang, P. Bhunia and R. Y. Surampalli, Treatment technologies for emerging contaminants in wastewater treatment plants: A review, Sci. Total Environ., 2020, 753, 141990, DOI:10.1016/j.scitotenv.2020.141990.
- F. Khalid, M. Z. Hashmi, N. Jamil, A. Qadir and M. I. Ali, Microbial and enzymatic degradation of PCBs from e-waste-contaminated sites: a review, Environ. Sci. Pollut. Res., 2021, 28, 10474–10487, DOI:10.1007/s11356-020-11996-2.
- A. Silva, C. Delerue-Matos, S. A. Figueiredo and O. M. Freitas, The Use of Algae and Fungi for Removal of Pharmaceuticals by Bioremediation and Biosorption Processes: A Review, Water, 2019, 11, 1555, DOI:10.3390/w11081555.
- S. F. Chen, W.-J. Chen, Y. Huang, M. Wei and C. Chang, Insights into the metabolic pathways and biodegradation mechanisms of chloroacetamide herbicides, Environ. Res., 2023, 229, 115918, DOI:10.1016/j.envres.2023.115918.
- F. Ansari, A. Momina, A. Ahmad and M. Rafatullah, Review on bioremediation technologies of polycyclic aromatic hydrocarbons (PAHs) from soil: Mechanisms and future perspective, Int. Biodeterior. Biodegrad., 2023, 179, 105582, DOI:10.1016/j.ibiod.2023.105582.
- M. N. Abbasi, J. Fu, X. Bian, H. Wang, Y. Zhang and A. Li, Recombineering for Genetic Engineering of Natural Product Biosynthetic Pathways, Trends Biotechnol., 2020, 38, 715–728, DOI:10.1016/j.tibtech.2019.12.018.
- P. Patra, M. Das, P. Kundu and A. Ghosh, Recent advances in systems and synthetic biology approaches for developing novel cell-factories in non-conventional yeasts, Biotechnol. Adv., 2021, 47, 107695, DOI:10.1016/j.biotechadv.2021.107695.
- E. Sagir and S. Alipour, Photofermentative hydrogen production by immobilized photosynthetic bacteria: Current perspectives and challenges, Renewable Sustainable Energy Rev., 2021, 141, 110796, DOI:10.1016/j.rser.2021.110796.
- R. A. Sheldon, A. Basso and D. Brady, New frontiers in enzyme immobilisation: robust biocatalysts for a circular bio-based economy, Chem. Soc. Rev., 2021, 50, 5850–5862, 10.1039/D1CS00015B.
- A. Rahmani, M. Salari, K. Tari, A. Shabanloo, N. Shabanloo and S. Bajalan, Enhanced degradation of furfural by heat-activated persulfate/nZVI-rGO oxidation system; Degradation pathway and improving the biodegradability of oil refinery wastewater, J. Environ. Chem. Eng., 2020, 8, 104468, DOI:10.1016/j.jece.2020.104468.
- M. A. Garcia, M. B. Bolger, S. Suarez-Sharp and P. Langguth, Predicting pharmacokinetics of multisource acyclovir oral products through physiologically based biopharmaceutics modeling, J. Pharm. Sci., 2022, 111, 262–273, DOI:10.1016/j.xphs.2021.10.013.
- G. Wang, R. Chen, P. Huang, J. Hong, J. Cao, Q. Wu, W. Zheng, L. Lin, Q. Han, Y. Chen and N. Xia, Adefovir dipivoxil efficiently inhibits the proliferation of pseudorabies virus in vitro and in vivo, Antiviral Res., 2021, 186, 105014, DOI:10.1016/j.antiviral.2021.105014.
- W. Danysz, A. Dekundy, A. Scheschonka and P. Riederer, Amantadine: reappraisal of the timeless diamond—target updates and novel therapeutic potentials, J. Neural Transm., 2021, 128, 127–169, DOI:10.1007/s00702-021-02306-2.
- J. Rajeev, K. Kamalasanan, H. Sapa, M. Sabitha and C. Abhi, Controlled release nanomedicine (CRNM) of aspirin using “biomimetic niosomal nanoparticles (BNNs)” for Covid-19 and cardiovascular treatment: DOE based optimization, OpenNano, 2023, 9, 100119, DOI:10.1016/j.onano.2022.100119.
- A. Newman, S. M. Reutzel-Edens and G. Zografi, Coamorphous active pharmaceutical ingredient–small molecule mixtures: considerations in the choice of coformers for enhancing dissolution and oral bioavailability, J. Pharm. Sci., 2018, 107, 5–17, DOI:10.1016/j.xphs.2017.09.024.
- K. Dolle and Q. Wang, Application of subsurface bioreactor for wastewater treatment, Int. J. Earth Environ. Sci., 2017, 2, 1–6, DOI:10.15344/2456-351X/2017/132.
- M. L. Tkachenko, M. A. Loseva, L. E. Zhnyakina and A. V. Lyamin, Binary Medicinal System: Antiinflammatory Activity Of The Binary Eutectic System, Probl. Biol., Med. Pharm. Chem., 2021, 24, 35–41, DOI:10.29296/25877313-2021-02-06.
- P. Saxena, P. K. Sharma and P. Purohit, A journey of celecoxib from pain to cancer, Prostaglandins Other Lipid Mediators, 2020, 147, 106379, DOI:10.1016/j.prostaglandins.2019.106379.
- R. R. Tjandrawinata, A. Setiawati, D. Nofiarny, L. W. Susanto and E. Setiawati, Pharmacokinetic equivalence study of nonsteroidal anti-inflammatory drug etoricoxib, Clin. Pharmacol., 2018, 10, 43–51, DOI:10.2147/CPAA.S161024.
- A. Mansoori, F. Zahednezhad, A. Bavili Tabrizi and J. Shahbazi Mojarrad, Development of a new synthesis approach for S-pregabalin by optimizing the preparation stages, J. Iran. Chem. Soc., 2020, 17, 89–101, DOI:10.1007/s13738-019-01759-3.
- L. Gaohua, X. Miao and L. Dou, Crosstalk of physiological pH and chemical pKa under the umbrella of physiologically based pharmacokinetic modeling of drug absorption, distribution, metabolism, excretion, and toxicity, Expert Opin. Drug Metab. Toxicol., 2021, 17, 1103–1124, DOI:10.1080/17425255.2021.1951223.
- H. Nabizadeh, A. Mohammadi, R. Dolatabadi, S. Nojavan and F. Vahabizad, Sensitive determination of ethosuximide in human fluids by electromembrane extraction coupled with high performance liquid chromatography ultraviolet spectroscopy, J. Chin. Chem. Soc., 2023, 70, 76–86, DOI:10.1002/jccs.202200423.
- J. Choi, D. Yoon, M. Park, K. I. Joung and J. Y. Shin, Topiramate-related adverse events: Pattern and signals in the Korea Adverse Event Reporting System, 2010-2017, Medicine, 2020, 99, e22669, DOI:10.1097/MD.0000000000022669.
- S. K. Ettaboina, L. N. R. Katakam and T. Dongala, Development and validation of a stability-indicating RP-HPLC method for the determination of erythromycin related impurities in topical dosage form, Pharm. Chem. J., 2022, 56, 131–137, DOI:10.1007/s11094-022-02610-5.
- R. Zhuan and J. Wang, Degradation of sulfamethoxazole by ionizing radiation: Kinetics and implications of additives, Sci. Total Environ., 2019, 668, 67–73, DOI:10.1016/j.scitotenv.2019.03.027.
- F. Y. S. Kong, P. Horner, M. Unemo and J. S. Hocking, Pharmacokinetic considerations regarding the treatment of bacterial sexually transmitted infections with azithromycin: a review, J. Antimicrob. Chemother., 2019, 74, 1157–1166, DOI:10.1093/jac/dky548.
- J. Berges, S. Moles, M. P. Ormad, R. Mosteo and J. Gómez, Antibiotics removal from aquatic environments: adsorption of enrofloxacin, trimethoprim, sulfadiazine, and amoxicillin on vegetal powdered activated carbon, Environ. Sci. Pollut. Res., 2021, 28, 8442–8452, DOI:10.1007/s11356-020-10972-0.
- S. Ghimire, B. Maharjan, E. M. Jongedijk, J. G. Kosterink, G. R. Ghimire, D. J. Touw, T. S. van der Werf, B. Shrestha and J. W. C. Alffenaar, Evaluation of saliva as a potential alternative sampling matrix for therapeutic drug monitoring of levofloxacin in patients with multidrug-resistant tuberculosis, Antimicrob. Agents Chemother., 2019, 63, e0237918, DOI:10.1128/aac.02379-18.
- D. Dixit, P. Thanekar and V. M. Bhandari, Dual activity cavitation reactors for increased efficacy in degradation of refractory pollutants–A case study on cephalexin degradation, Chem. Eng. Res. Des., 2023, 192, 310–322, DOI:10.1016/j.cherd.2023.02.037.
- R. Pratiwi, E. Noviana, R. Fauziati, D. B. Carrão, F. A. Gandhi, M. A. Majid and F. A. Saputri, A review of analytical methods for codeine determination, Molecules, 2021, 26, 800, DOI:10.3390/molecules26040800.
- G. Del Vecchio, D. Labuz, J. Temp, V. Seitz, M. Kloner, R. Negrete, A. Rodriguez-Gaztelumendi, M. Weber, H. Machelska and C. Stein, pKa of opioid ligands as a discriminating factor for side effects, Sci. Rep., 2019, 9, 1–9, DOI:10.1038/s41598-019-55886-1.
- T. W. Hale and K. Krutsch, Opioid use in breastfeeding mothers and neonatal risks, Clin. Pharmacol. Ther., 2021, 109, 573–575, DOI:10.1002/cpt.2118.
- B. M. Kapur and K. Aleksa, What the lab can and cannot do: clinical interpretation of drug testing results, Crit. Rev. Clin. Lab. Sci., 2020, 57, 548–585, DOI:10.1080/10408363.2020.1774493.
- E. Naghdi and A. R. Fakhari, Simultaneous chiral separation of tramadol and methadone in tablets, human urine, and plasma by capillary electrophoresis using maltodextrin as the chiral selector, Chirality, 2018, 30, 1161–1168, DOI:10.1002/chir.23008.
- S. L. Badshah, S. Faisal, A. Muhammad, B. G. Poulson, A. H. Emwas and M. Jaremko, Antiviral activities of flavonoids, Biomed. Pharmacother., 2021, 140, 111596, DOI:10.1016/j.biopha.2021.111596.
- C. Nannou, A. Ofrydopoulou, E. Evgenidou, D. Heath, E. Heath and D. Lambropoulou, Antiviral drugs in aquatic environment and wastewater treatment plants: A review on occurrence, fate, removal and ecotoxicity, Sci. Total Environ., 2020, 699, 134322, DOI:10.1016/j.scitotenv.2019.134322.
- M. Hutchings, A. Truman and B. Wilkinson, Antibiotics: past, present and future, Curr. Opin. Microbiol., 2019, 51, 72–80, DOI:10.1016/j.mib.2019.10.008.
- J. Murugaiyan, P. A. Kumar, G. S. Rao, K. Iskandar, S. Hawser, J. P. Hays, Y. Mohsen, S. Adukkadukkam, W. A. Awuah and R. A. M. Jose,
et al., Progress in Alternative Strategies to Combat Antimicrobial Resistance: Focus on Antibiotics, Antibiotics, 2022, 11, 200, DOI:10.3390/antibiotics11020200.
- P. Krasucka, B. Pan, Y. Sik Ok, D. Mohan, B. Sarkar and P. Oleszczuk, Engineered biochar - A sustainable solution for the removal of antibiotics from water, Chem. Eng. J., 2020, 405, 126926, DOI:10.1016/j.cej.2020.126926.
- N. K. Panchal and E. P. Sabina, Non-steroidal anti-inflammatory drugs (NSAIDs): A current insight into its molecular mechanism eliciting organ toxicities, Food Chem. Toxicol., 2023, 172, 113598, DOI:10.1016/j.fct.2022.113598.
- S. Bindu, S. Mazumder and U. Bandyopadhyay, Non-steroidal anti-inflammatory drugs (NSAIDs) and organ damage: a current perspective, Biochem. Pharmacol., 2020, 180, 114147, DOI:10.1016/j.bcp.2020.114147.
- R. Pal, B. Kumar, M. J. Akhtar and P. A. Chawla, Voltage gated sodium channel inhibitors as anticonvulsant drugs: A systematic review on recent developments and structure activity relationship studies, Bioorg. Chem., 2021, 115, 105230, DOI:10.1016/j.bioorg.2021.105230.
- E. Tampakakis and A. I. Mahmoud, The role of hormones and neurons in cardiomyocyte maturation, Semin. Cell Dev. Biol., 2021, 118, 136–143, DOI:10.1016/j.semcdb.2021.03.026.
- S. J. Sills and M. A. Rogawski, Mechanisms of action of currently used antiseizure drugs, Neuropharmacology, 2020, 168, 107966, DOI:10.1016/j.neuropharm.2020.107966.
- N. Ohashi and T. Kohno, Analgesic effect of acetaminophen: a review of known and novel mechanisms of action, Front. Pharmacol., 2020, 11, 580289, DOI:10.3389/fphar.2020.580289.
- A. Jafarzadeh, M. Nemati, H. Khorramdelazad and Z. M. Hassan, Immunomodulatory properties of cimetidine: Its therapeutic potentials for treatment of immune-related diseases, Int. Immunopharmacol., 2019, 70, 156–166, DOI:10.1016/j.intimp.2019.02.026.
- S. Zhao, R. Zhang, Y. Gao, Y. Cheng, S. Zhao, M. Li, H. Li and J. Dong, Immunosensor for Rapid and Sensitive Detection of Digoxin, ACS Omega, 2023, 8, 15341–15349, DOI:10.1021/acsomega.3c00571.
- H. Li, M. Zhang, L. Xiong, W. Feng and R. O. Williams III, Bioavailability improvement of carbamazepine via oral administration of modified-release amorphous solid dispersions in rats, Pharmaceutics, 2020, 12, 1023, DOI:10.3390/pharmaceutics12111023.
- J. Lee, X. Kong, B. Haymart, E. Kline-Rogers, S. Kaatz, V. Shah, M. A. Ali, J. Kozlowski, J. Froehlich and G. D. Barnes, Outcomes in patients undergoing periprocedural interruption of warfarin or direct oral anticoagulants, J. Thromb. Haemostasis, 2022, 20, 2571–2578, DOI:10.1111/jth.15850.
- Z. Cai, Y. Lin and J. Liang, Efficacy of salbutamol in the treatment of infants with bronchiolitis: A meta-analysis of 13 studies, Medicine, 2020, 99, e18657, DOI:10.1097/MD.0000000000018657.
- N. M. Ibrahim, S. H. Fahim, M. Hassan, A. E. Farag and H. H. Georgey, Design and synthesis of ciprofloxacin-sulfonamide hybrids to manipulate ciprofloxacin pharmacological qualities: Potency and side effects, Eur. J. Med. Chem., 2022, 228, 114021, DOI:10.1016/j.ejmech.2021.114021.
- A. Olasunkanmi and A. Isreal, A narrative review of codeine and preventive measures in mitigating against the widespread of its abuse and misuse, J. Alcohol Drug Depend., 2019, 7, 323, DOI:10.4172/2329-6488.1000323.
- H. J. Wang, E. C. H. Tan, T. Y. Chiang, W. C. Chen, C. C. Shen and Y. F. Ueng, Effect of repeated Shengmai-San administration on nifedipine pharmacokinetics and the risk/benefit under co-treatment, J. Food Drug Anal., 2022, 30, 111–127, DOI:10.38212/2224-6614.3401.
- S. Tavakoli-Dastjerdi, M. Motavasselian, S. A. Emami, M. Mansourian, A. Sahebkar and A. Teimouri, Efficacy of a combination of herbal gel versus topical diltiazem (2%) in chronic anal fissure healing: a randomized doubleblind clinical trial, J. HerbMed Pharmacol., 2019, 8, 139–145, DOI:10.15171/jhp.2019.22.
- M. Fiorillo, F. Tóth, F. Sotgia and M. P. Lisanti, Doxycycline, Azithromycin and Vitamin C (DAV): A potent combination therapy for targeting mitochondria and eradicating cancer stem cells (CSCs), Aging (Albany NY), 2019, 11, 2202–2216, DOI:10.18632/aging.101905.
- J. L. Tarry-Adkins, I. D. Grant, S. E. Ozanne, R. M. Reynolds and C. E. Aiken, Efficacy and side effect profile of different formulations of metformin: a systematic review and meta-analysis, Diabetes Ther., 2021, 12, 1901–1914, DOI:10.1007/s13300-021-01058-2.
- N. A. Khan, S. U. Khan, S. Ahmed, I. H. Farooqi, M. Yousefi, A. A. Mohammadi and F. Changani, Recent Trends in Disposal and Treatment Technologies of Emerging-Pollutants-A Critical Review, Trends Anal. Chem., 2020, 122, 115744, DOI:10.1016/j.trac.2019.115744.
- Z. Dang, M. Arena and A. Kienzler, Fish toxicity testing for identification of thyroid disrupting chemicals, Environ. Pollut., 2021, 284, 117374, DOI:10.1016/j.envpol.2021.117374.
- B. Huerta, S. Rodriguez-Mozaz, J. Lazorchak, D. Barcelo, A. Batt, J. Wathen and L. Stahl, Presence of pharmaceuticals in fish collected from urban rivers in the U.S. EPA 2008–2009 National Rivers and Streams Assessment, Sci. Total Environ., 2018, 634, 542–549, DOI:10.1016/j.scitotenv.2018.03.387.
- D. Reynolds, M. Huesemann, S. Edmundson, A. Sims, B. Hurst, S. Cady, N. Beirne, J. Freeman, A. Berger and S. Gao, Viral inhibitors derived from macroalgae, microalgae, and cyanobacteria: A review of antiviral potential throughout pathogenesis, Algal Res., 2021, 57, 102331, DOI:10.1016/j.algal.2021.102331.
- M. D. Machado and E. V. Soares, Impact of erythromycin on a non-target organism: Cellular effects on the freshwater microalga Pseudokirchneriella subcapitata, Aquat. Toxicol., 2019, 208, 179–186, DOI:10.1016/j.aquatox.2019.01.014.
- J. Katz, A. Y. Kargi and R. Ramasamy, Re: Ibuprofen Alters Human Testicular Physiology To Produce a State of Compensated Hypogonadism, Eur. Urol., 2018, 74, 394–395, DOI:10.1016/j.eururo.2018.03.039.
- S. Yadav, S. Kumar and A. K. Haritash, A comprehensive review of chlorophenols: Fate, toxicology and its treatment, J. Environ. Manage., 2023, 342, 118254, DOI:10.1016/j.jenvman.2023.118254.
- P. Sharma, P. Kaur, P. Ghanghas, J. Kaur and N. Kaushal, Selenium Ameliorates Ibuprofen Induced Testicular Toxicity by Redox Regulation, Reprod. Toxicol., 2020, 96, 349–358, DOI:10.1016/j.reprotox.2020.08.005.
- S. Pang, Z. Lin, W.-J. Chen, S.-F. Chen, Y. Huang, Q. Lei, P. Bhatt, S. Mishra, S. Chen and H. Wang, High-efficiency degradation of methomyl by the novel bacterial consortium MF0904: Performance, structural analysis, metabolic
pathways, and environmental bioremediation, J. Hazard. Mater., 2023, 452, 131287, DOI:10.1016/j.jhazmat.2023.131287.
- E. Felis, J. Kalka, A. Sochacki, K. Kowalska, S. Bajkacz, M. Harnisz and E. Korzeniewska, Antimicrobial pharmaceuticals in the aquatic environment - occurrence and environmental implications, Eur. J. Pharmacol., 2019, 866, 172813, DOI:10.1016/j.ejphar.2019.172813.
- A. S. Adeleye, J. Xue, Y. Zhao, A. A. Taylor, J. E. Zenobio, Y. Sun, Z. Han, O. A. Salawu and Y. Zhu, Abundance, fate, and effects of pharmaceuticals and personal care products in aquatic environments, J. Hazard. Mater., 2022, 424, 127284, DOI:10.1016/j.jhazmat.2021.127284.
- D. Khurana, R. Pulicharla and S. K. Brar, Antibiotic-metal complexes in wastewaters: fate and treatment trajectory, Environ. Int., 2021, 157, 106863, DOI:10.1016/j.envint.2021.106863.
- L. Duan, Y. Zhang, B. Wang, Y. Zhou, F. Wang, Q. Sui, D. Xu and G. Yu, Seasonal occurrence and source analysis of pharmaceutically active compounds (PhACs) in aquatic environment in a small and medium-sized city, China, Sci. Total Environ., 2021, 769, 144272, DOI:10.1016/j.scitotenv.2020.144272.
- Y. Kuang, X. Guo, J. Hu, S. Li, R. Zhang, Q. Gao, X. Yang, Q. Chen and W. Sun, Occurrence and risks of antibiotics in an urban river in northeastern Tibetan Plateau, Sci. Rep., 2020, 10, 20054, DOI:10.1038/s41598-020-77152-5.
- J. P. Bavumiragira, J. Ge and H. Yin, Fate and transport of pharmaceuticals in water systems: A processes review, Sci. Total Environ., 2022, 823, 153635, DOI:10.1016/j.scitotenv.2022.153635.
- I. Eheneden, R. Wang and J. Zhao, Antibiotic removal by microalgae-bacteria consortium: Metabolic pathways and microbial responses, Sci. Total Environ., 2023, 891, 164489, DOI:10.1016/j.scitotenv.2023.164489.
- Y. Chen, W.-J. Chen, Y. Huang, J. Li, J. Zhong, W. Zhang, Y. Zou, S. Mishra, P. Bhatt and S. Chen, Insights into the microbial degradation and resistance mechanisms of glyphosate, Environ. Res., 2022, 215, 114153, DOI:10.1016/j.envres.2022.114153.
- S. Bose, P. Senthil Kumar, D. V.-N. Vo, N. Rajamohan and R. Saravanan, Microbial degradation of recalcitrant pesticides: a review, Environ. Chem. Lett., 2021, 19, 3209–3228, DOI:10.1007/s10311-021-01236-5.
- N. Haleyur, E. Shahsavari, S. S. Jain, E. Koshlaf, V. B. Ravindran, P. D. Morrison, A. M. Osborn and A. S. Ball, Influence of bioaugmentation and biostimulation on PAH degradation in aged contaminated soils: Response and dynamics of the bacterial community, J. Environ. Manage., 2019, 238, 49–58, DOI:10.1016/j.jenvman.2019.02.115.
- D. H. Liang, Y. Hu, J. Cheng and Y. Chen, Simultaneous sulfamethoxazole biodegradation and nitrogen conversion in low C/N ratio pharmaceutical wastewater by Achromobacter sp. JL9, Sci. Total Environ., 2019, 703, 135586, DOI:10.1016/j.scitotenv.2019.135586.
- M. Narayanan, S. S. Ali and M. El-Sheekh, A comprehensive review on the potential of microbial enzymes in multipollutant bioremediation: Mechanisms, challenges, and future prospects, J. Environ. Manage., 2023, 334, 117532, DOI:10.1016/j.jenvman.2023.117532.
- I. S. Moreira, V. S. Bessa, S. Murgolo, C. Piccirillo, G. Mascolo and P. M. L. Castro, Biodegradation of Diclofenac by the bacterial strain Labrys portucalensis F11, Ecotoxicol. Environ. Saf., 2018, 152, 104–113, DOI:10.1016/j.ecoenv.2018.01.040.
- I. Liakh, D. Harshkova, P. Hrouzek, K. Bisova, A. Aksmann and B. Wielgomas, Green alga Chlamydomonas reinhardtii can effectively remove diclofenac
from the water environment – A new perspective on biotransformation, J. Hazard. Mater., 2023, 455, 131570, DOI:10.1016/j.jhazmat.2023.131570.
- D. R. Olicon-Hernandez, M. Ortuzar, C. Pozo, J. González-López and E. Aranda, Metabolic capability of Penicillium oxalicum to transform high concentrations of anti-inflammatory and analgesic drugs, Appl. Sci., 2020, 10, 2479, DOI:10.3390/app10072479.
- C. V. Ungureanu, L. Favier and G. E. Bahrim, Improving Biodegradation of Clofibric Acid by Trametes pubescens through the Design of Experimental Tools, Microorganisms, 2020, 8, 1243, DOI:10.3390/microorganisms8081243.
- P. Bhatt, E. R. Rene, Y. Huang, X. Wu, Z. Zhou, J. Li, A. J. Kumar, A. Sharma and S. Chen, Indigenous bacterial consortium-mediated cypermethrin degradation in the presence of organic amendments and Zea mays plants, Environ. Res., 2022, 212, 113137, DOI:10.1016/j.envres.2022.113137.
- W. Zhang, W. J. Chen, S.-F. Chen, Q. Lei, J. Li, P. Bhatt, S. Mishra and S. Chen, Cellular Response and Molecular Mechanism of Glyphosate Degradation by Chryseobacterium sp. Y16C, J. Agric. Food Chem., 2023, 71, 6650–6661, DOI:10.1021/acs.jafc.2c07301.
- T. Hua, S. Li, F. Li, B. S. Ondon, Y. Liu and H. Wang, Degradation performance and microbial community analysis of microbial electrolysis cells for erythromycin wastewater treatment, Biochem. Eng. J., 2019, 146, 1–9, DOI:10.1016/j.bej.2019.02.008.
- Z. Dong, X. Yan, J. Wang, L. Zhu, J. Wang, C. Li, W. Zhang, S. Wen and Y. M. Kim, Mechanism for biodegradation of sulfamethazine by Bacillus cereus H38, Sci. Total Environ., 2022, 809, 152237, DOI:10.1016/j.scitotenv.2021.152237.
- F. Yang, H. Jian, C. Wang, Y. Wang, E. Li and H. Sun, Effects of biochar on biodegradation of sulfamethoxazole and chloramphenicol by Pseudomonas stutzeri and Shewanella putrefaciens: Microbial growth, fatty acids, and the expression quantity of genes, J. Hazard. Mater., 2021, 406, 124311, DOI:10.1016/j.jhazmat.2020.124311.
- J. Ren, Z. Wang, L. Deng, D. Niu, B. Fan, T. Huhe, Z. Li, J. Zhang and C. Li, Biodegradation of erythromycin by Delftia lacustris RJJ-61 and characterization of its erythromycin esterase, J. Basic Microbiol., 2021, 61, 55–62, DOI:10.1002/jobm.202000613.
- J. Yang, Z. Q. Zhao, M. Wang, K. F. Yu, T. Zhang, H. Lin and H. B. Zheng, Biodegradation of tylosin in swine wastewater by Providencia stuartii TYL-Y13: Performance, pathway, genetic background, and risk assessment, J. Hazard. Mater., 2022, 440, 129716, DOI:10.1016/j.jhazmat.2022.129716.
- J. Zhang, X. Li, H. Lei, R. Zhao, W. Gan, K. Zhou and B. Li, New insights into thiamphenicol biodegradation mechanism by Sphingomonas sp. CL5. 1 deciphered through metabolic and proteomic analysis, J. Hazard. Mater., 2022, 426, 128101, DOI:10.1016/j.jhazmat.2021.128101.
- X. X. Kong, J. L. Jiang, B. Qiao, H. Liu, J. S. Cheng and Y. J. Yuan, The biodegradation of cefuroxime, cefotaxime and cefpirome by the synthetic consortium with probiotic Bacillus clausii and investigation of their potential biodegradation pathways, Sci. Total Environ., 2019, 651, 271–280, DOI:10.1016/j.scitotenv.2018.09.187.
- S. Shao, Y. Hu, J. Cheng and Y. Chen, Biodegradation mechanism of tetracycline (TEC) by strain Klebsiella sp. SQY5 as revealed through products analysis and genomics, Ecotoxicol. Environ. Saf., 2019, 185, 109676, DOI:10.1016/j.ecoenv.2019.109676.
- E. Rivera-Gutierrez, J. J. Ramírez-García, S. H. Pavón Romero, M. M. Rodríguez, A. Ramírez-Serrano and A. Jiménez-Marin, Dicloxacillin degradation with free-living bacteria, Water, Air, Soil Pollut., 2020, 231, 1–13, DOI:10.1007/s11270-020-4456-7.
- N. Kamal, A. Tarafdar, A. Sinha and V. Kumar, Effect of Glucose Cometabolism on Biodegradation of Gabapentin (an Anticonvulsant Drug) by Gram-Positive Bacteria Micrococcus luteus N. ISM. 1, Appl. Biochem. Microbiol., 2020, 56, 433–440, DOI:10.1134/S0003683820040067.
- S. Sharma, H. Setia and A. P. Toor, Assessing the bioremediation potential of indigenously isolated Klebsiella sp. WAH1 for diclofenac sodium: optimization, toxicity and metabolic pathway studies, World J. Microbiol. Biotechnol., 2021, 37, 1–12, DOI:10.1007/s11274-021-02998-4.
- M. Dabrowska, B. Muszyńska, M. Starek, P. Żmudzki and W. Opoka, Degradation pathway of cephalosporin antibiotics by in vitro cultures of Lentinula edodes and Imleria badia, Int. Biodeterior. Biodegrad., 2018, 127, 104–112, DOI:10.1016/j.ibiod.2017.11.014.
- L. S. Copete-Pertuz, J. Plácido, E. A. Serna-Galvis, R. A. Torres-Palma and A. Mora, Elimination of isoxazolyl-penicillins antibiotics in waters by the ligninolytic native colombian strain Leptosphaerulina sp. considerations on biodegradation process and antimicrobial activity removal, Sci. Total Environ., 2018, 630, 1195–1204, DOI:10.1016/j.scitotenv.2018.02.244.
- B. Muszynska, P. Żmudzki, J. Lazur, K. Kała, K. Sułkowska-Ziaja and W. Opoka, Analysis of the biodegradation of synthetic testosterone and 17α-ethynylestradiol using the edible mushroom Lentinula edodes, 3 Biotech, 2018, 8, 1–15, DOI:10.1007/s13205-018-1458-x.
- J. de Jesus Menk, A. I. S. do Nascimento, F. G. Leite, R. A. de Oliveira, A. F. Jozala, J. M. de Oliveira Junior, M. V. Chaud and D. Grotto, Biosorption of pharmaceutical products by mushroom stem waste, Chemosphere, 2019, 237, 124515, DOI:10.1016/j.chemosphere.2019.124515.
- D. R. Olicon-Hernandez, M. Ortuzar, C. Pozo, J. González-López and E. Aranda, Metabolic capability of Penicillium oxalicum to transform high concentrations of anti-inflammatory and analgesic drugs, Appl. Sci., 2020, 10, 2479, DOI:10.3390/app10072479.
- R. Ahumada-Rudolph, V. Novoa, J. Becerra, C. Cespedes and J. R. Cabrera-Pardo, Mycoremediation of oxytetracycline by marine fungi mycelium isolated from salmon farming areas in the south of Chile, Food Chem. Toxicol., 2021, 152, 112198, DOI:10.1016/j.fct.2021.112198.
- J. Ren, Z. Wang, L. Deng, D. Niu, Huhetaoli, Z. Li, L. Dong, J. Zhang, R. Zhang and C. Li, Degradation of erythromycin by a novel fungus, Penicillium oxalicum RJJ-2, and the degradation pathway, Waste Biomass Valorization, 2021, 12, 4513–4523, DOI:10.1007/s12649-021-01343-y.
- A. Chatterjee and J. Abraham, Mycoremediation of 17 β-Estradiol Using Trichoderma citrinoviride Strain AJAC3 along with Enzyme Studies, Environ. Prog. Sustainable Energy, 2019, 38, 13142, DOI:10.1002/ep.13142.
- R. Manasfi, S. Chiron, N. Montemurro, S. Perez and M. Brienza, Biodegradation of fluoroquinolone antibiotics and the climbazole fungicide by Trichoderma species, Environ. Sci. Pollut. Res., 2020, 27, 23331–23341, DOI:10.1007/s11356-020-08442-8.
- T. Zhang, L. Cai, B. Xu, X. Li, W. Qiu, C. Fu and C. Zheng, Sulfadiazine biodegradation by Phanerochaete chrysosporium: Mechanism and degradation product identification, Chemosphere, 2019, 237, 124418, DOI:10.1016/j.chemosphere.2019.124418.
- A. Stenholm, M. Hedeland, T. Arvidsson and C. E. Pettersson, Removal of diclofenac from a non-sterile aqueous system using Trametes versicolor with an emphasis on adsorption and biodegradation mechanisms, Environ. Technol., 2019, 40, 2460–2472, DOI:10.1080/09593330.2018.1444098.
- C. Song, Y. Wei, Y. Qiu, Y. Qi, Y. Li and Y. Kitamura, Biodegradability and mechanism
of florfenicol via Chlorella sp. UTEX1602 and L38: experimental study, Bioresour. Technol., 2019, 272, 529–534, DOI:10.1016/j.biortech.2018.10.080.
- C. Song, Y. Wei, J. Sun, Y. Song, S. Li and Y. Kitamura, Biodegradation and metabolic fate of thiamphenicol via Chlorella sp. UTEX1602 and L38, Bioresour. Technol., 2020, 296, 122320, DOI:10.1016/j.biortech.2019.122320.
- L. Jalowiecki, G. Płaza, H. Ejhed and M. Nawrotek, Aerobic biodegradation of norfloxacin and ofloxacin by a microbial consortium, Arch. Environ. Prot., 2019, 45, 40–47, DOI:10.24425/aep.2019.130240.
- S. Shao and X. Wu, Microbial degradation of tetracycline in the aquatic environment: a review, Crit. Rev. Biotechnol., 2020, 40, 1010–1018, DOI:10.1080/07388551.2020.1805585.
- S. N. Malik, P. C. Ghosh, A. N. Vaidya and S. N. Mudliar, Hybrid ozonation process for industrial wastewater treatment: Principles and applications: A review, J. Water Process Eng., 2020, 35, 101193, DOI:10.1016/j.jwpe.2020.101193.
- A. Marchlewicz, U. Guzik, W. Smulek and D. Wojcieszynska, Exploring the Degradation of Ibuprofen by Bacillus thuringiensis B1(2015b): The New Pathway and Factors Affecting Degradation, Molecules, 2017, 22, 1676, DOI:10.3390/molecules22101676.
- J. Wang and S. Wang, Microbial degradation of sulfamethoxazole in the environment, Appl. Microbiol. Biotechnol., 2018, 102, 3573–3582, DOI:10.1007/s00253-018-8845-4.
- A. S. Al Hosni, J. K. Pittman and G. D. Robson, Microbial degradation of four biodegradable polymers in soil and compost demonstrating polycaprolactone as an ideal compostable plastic, Waste Manage., 2019, 97, 105–114, DOI:10.1016/j.wasman.2019.07.042.
- L. Yu, Y. Wang, X. Shan, F. Ma and H. Guo, Harnessing Paenarthrobacter ureafaciens YL1 and Pseudomonas koreensis YL2 Interactions to Improve Degradation of Sulfamethoxazole, Microorganisms, 2022, 10, 648, DOI:10.3390/microorganisms10030648.
- J. P. Fernandes, C. M. R. Almeida, M. A. Salgado, M. F. Carvalho and A. P. Mucha, Pharmaceutical Compounds in Aquatic Environments—Occurrence, Fate and Bioremediation Prospective, Toxics, 2021, 9, 257, DOI:10.3390/toxics9100257.
- T. Velempini, E. Prabakaran and K. Pillay, Recent developments in the use of metal oxides for photocatalytic degradation of pharmaceutical pollutants in water—a review, Mater. Today Chem., 2021, 19, 100380, DOI:10.1016/j.mtchem.2020.100380.
- Y. Xue, P. Du, A. A. I. Shendi and B. Yu, Mercury bioremediation in aquatic environment by genetically modified bacteria with self-controlled biosecurity circuit, J. Cleaner Prod., 2022, 337, 130524, DOI:10.1016/j.jclepro.2022.130524.
- I. Hussain, G. Aleti, R. Naidu, M. Puschenreiter, Q. Mahmood, M. M. Rahman, F. Wang, S. Shaheen, J. H. Syed and T. G. Reichenauer, Microbe and plant assisted-remediation of organic xenobiotics and its enhancement by genetically modified organisms and recombinant technology: A review, Sci. Total Environ., 2018, 628, 1582–1599, DOI:10.1016/j.scitotenv.2018.02.037.
- R. Kent and N. Dixon, Contemporary Tools for Regulating Gene Expression in Bacteria, Trends Biotechnol., 2020, 38, 316–333, DOI:10.1016/j.tibtech.2019.09.007.
- P. Sharma, A. Bano, S. P. Singh, S. Sharma, C. Xia, A. K. Nadda, S. S. Lam and Y. W. Tong, Engineered microbes as effective tools for the remediation of polyaromatic aromatic hydrocarbons and heavy metals, Chemosphere, 2022, 306, 135538, DOI:10.1016/j.chemosphere.2022.135538.
- M. F. Adegboye, O. B. Ojuederie, P. M. Talia and O. O. Babalola, Bioprospecting of microbial strains for biofuel production: metabolic engineering, applications, and challenges, Biotechnol. Biofuels, 2021, 14, 5, DOI:10.1186/s13068-020-01853-2.
- P. Bhatt, K. Bhatt, A. Sharma, W. Zhang, S. Mishra and S. Chen, Biotechnological basis of microbial consortia for the removal of pesticides from the environment, Crit. Rev. Biotechnol., 2021, 41, 317–338, DOI:10.1080/07388551.2020.1853032.
- P. Sharma, P. Kaur, P. Ghanghas, J. Kaur and N. Kaushal, Selenium Ameliorates Ibuprofen Induced Testicular Toxicity by Redox Regulation, Reprod. Toxicol., 2020, 96, 349–358, DOI:10.1016/j.reprotox.2020.08.005.
- M. Aulestia, A. Flores, S. Acosta-Jurado, E. Santero and E. M. Camacho, Genetic Characterization of the Ibuprofen-Degradative Pathway of Rhizorhabdus wittichii MPO218, Appl. Environ. Microbiol., 2022, 8, e0038822, DOI:10.1128/aem.00388-22.
- A. B. Patel, S. Shaikh, K. R. Jain, C. Desai and D. Madamwar, Polycyclic aromatic hydrocarbons: sources, toxicity, and remediation approaches, Front. Microbiol., 2020, 11, 562813, DOI:10.3389/fmicb.2020.562813.
- K. T. Sriwong and T. Matsuda, Recent Advances in Enzyme Immobilization Utilizing Nanotechnology for Biocatalysis, Org. Process Res. Dev., 2022, 26, 1857–1877, DOI:10.1021/acs.oprd.1c00404.
- M. U. Saeed, N. Hussain, A. Sumrin, A. Shahbaz, S. Noor, M. Bilal, L. Aleya and H. M. N. Iqbal, Microbial bioremediation strategies with wastewater treatment potentialities – A review, Sci. Total Environ., 2022, 818, 151754, DOI:10.1016/j.scitotenv.2021.151754.
- D. Pandey, A. Daverey and K. Arunachalam, Biochar: Production, Properties and Emerging role as a Support for Enzyme Immobilization, J. Cleaner Prod., 2020, 255, 120267, DOI:10.1016/j.jclepro.2020.120267.
- A. R. Ismail and K. H. Baek, Lipase immobilization with support materials, preparation techniques, and applications: Present and future aspects, Int. J. Biol. Macromol., 2020, 163, 1624–1639, DOI:10.1016/j.ijbiomac.2020.09.021.
- M. Masjoudi, M. Golgoli, Z. G. Nejad, S. Sadeghzadeh and S. M. Borghei, Pharmaceuticals Removal by Immobilized Laccase on Polyvinylidene Fluoride Nanocomposite with Multi-Walled Carbon Nanotubes, Chemosphere, 2020, 263, 128043, DOI:10.1016/j.chemosphere.2020.128043.
- L. Wang, S. Guan, J. Bai, J. Gao, Y. Song, X. Zheng and Y. Jiang, Enzyme immobilized in BioMOFs: Facile synthesis and improved catalytic performance, Int. J. Biol. Macromol., 2020, 144, 19–28, DOI:10.1016/j.ijbiomac.2019.12.054.
- P. R. Yaashikaa, M. Keerthana Devi and P. Senthil Kumar, Advances in the application of immobilized enzyme for the remediation of hazardous pollutant: A review, Chemosphere, 2022, 299, 134390, DOI:10.1016/j.chemosphere.2022.134390.
- M. Primozic, G. Kravanja, Z. Knez, A. Crnjac and M. Leitgeb, Immobilized laccase in the form of (magnetic) cross-linked enzyme aggregates for sustainable diclofenac (bio)degradation, J. Cleaner Prod., 2020, 275, 124121, DOI:10.1016/j.jclepro.2020.124121.
- J. Zur, A. Piński, J. Michalska, K. Hupert-Kocurek, A. Nowak, D. Wojcieszyńska and U. Guzik, A whole-cell immobilization system on bacterial cellulose for the paracetamol-degrading Pseudomonas moorei KB4 strain, Int. Biodeterior. Biodegrad., 2020, 149, 104919, DOI:10.1016/j.ibiod.2020.104919.
- X. Wu, W. J. Chen, Z. Lin, Y. Huang, T. N.-M. E. Sebai, N. Alansary, D. E. El-Hefny, S. Mishra, P. Bhatt, H. Lu and S. Chen, Rapid Biodegradation of the Organophosphorus Insecticide Acephate by a Novel Strain Burkholderia sp. A11 and Its Impact on the Structure of the Indigenous Microbial Community, J. Agric. Food Chem., 2023, 71, 5261–5274, DOI:10.1021/acs.jafc.2c07861.
- N. Kamal, R. Sindhu and P. C. Bhargava, Biodegradation of emerging organic pollutant gemfibrozil: Mechanism, kinetics and pathway modelling, Bioresour. Technol., 2023, 374, 128749, DOI:10.1016/j.biortech.2023.128749.
- Y. Huang, W.-J. Chen, J. Li, M. A. Ghorab, N. Alansary, D. E. El-Hefny, G. S. El-Sayyad, S. Mishra, X. Zhang, P. Bhatt and S. Chen, Novel mechanism and degradation kinetics of allethrin using Bacillus megaterium strain HLJ7 in contaminated soil/water environments, Environ. Res., 2022, 214, 113940, DOI:10.1016/j.envres.2022.113940.
- S. P. Sam, S. L. Ng and A. Rohana, Kinetics of biodegradation of phenol and p-nitrophenol by acclimated activated sludge, J. Phys. Sci., 2018, 29, 107–113, DOI:10.21315/jps2018.29.s1.14.
- S. S. Patil and H. M. Jena, Process optimization and kinetic study of biodegradation of dimethyl phthalate by KS2, a novel strain of Micrococcus sp., Sci. Rep., 2023, 13, 3900, DOI:10.1038/s41598-023-29256-x.
- G. Calero-Diaz, A. Monteoliva-García, J. C. Leyva-Díaz, C. López-López, J. Martín-Pascual, J. C. Torres and J. M. Poyatos, Impact of ciprofloxacin, carbamazepine and ibuprofen on a membrane bioreactor system: Kinetic study and biodegradation capacity, J. Chem. Technol. Biotechnol., 2017, 92, 2944–2951, DOI:10.1002/jctb.5316.
- L. Pan, J. Li, C. Li, X. Tang, G. Yu and Y. Wang, Study of ciprofloxacin biodegradation by a Thermus sp. isolated from pharmaceutical sludge, J. Hazard. Mater., 2018, 343, 59–67, DOI:10.1016/j.jhazmat.2017.09.009.
|
This journal is © The Royal Society of Chemistry 2023 |