DOI:
10.1039/D3QM00245D
(Research Article)
Mater. Chem. Front., 2023,
7, 2620-2627
Graphdiyne/copper sulfide heterostructure for active conversion of CO2 to formic acid†
Received
9th March 2023
, Accepted 28th March 2023
First published on 29th March 2023
Abstract
The synthesis of electrocatalysts with high selectivity, activity, and stability for the CO2 reduction reaction (CO2RR) is a promising and sustainable route to convert CO2 into value-added chemicals at room temperatures and pressures. Here we report a new heterostructured electrocatalyst of graphdiyne/copper sulfide (GDY/CuSx) via the controlled in situ growth of GDY on the surface of CuSx. Our results show that the introduction of GDY can effectively induce the formation of mixed-valence Cu(I, II) and incomplete charge transfer between the GDY and Cu atoms, which enhance the conductivity, produce new active sites, and finally result in a higher catalytic performance. In addition, the GDY grown on the surface of the catalysts endows the sample with a high long-term stability. Benefitting from above advantages, GDY/CuSx shows a high CO2-to-formate conversion performance with a high faradaic efficiency (FE) and long-term stability at room temperatures and ambient pressures.
1. Introduction
The electrocatalytic carbon dioxide reduction reaction (CO2RR) at room temperatures and ambient pressures provides an effective route for the production of high-value-added chemicals and fuels from CO2.1–4 Formic acid (HCOOH), an important hydrogen carrier, plays an important role in the storage and transportation of hydrogen energy.5–7 However, the catalysts reported for the CO2RR suffer from sluggish kinetics due to the high overpotentials required to drive the CO2RR process, in which the competing hydrogen evolution reaction (HER) occurs. Besides, the multi-proton/multi-electron transfer in the CO2RR process commonly results in multiple types of gas- and/or liquid-phase products (carbon monoxide, methane, ethylene, formic acid, formaldehyde, methanol, ethanol, etc.).8–10 These drawbacks of traditional catalysts lead to both low reaction selectivity and activity. During the past decades, many methods, such as alloying,11 chemical doping,12,13 catalyst surface modification,14etc., have been reported to improve the performance of the electrocatalytic CO2RR. The design and synthesis of new electrocatalysts that demonstrate high selectivity (faradaic efficiency (FE)), a high reaction rate (large current densities) and long-term stability for CO2-to-HCOOH conversion are still of great significance.
Graphdiyne (GDY) is a new two-dimensional all-carbon network in which each benzene ring (sp2-hybridized C) is connected via alkyne bonds (sp-hybridized C).15 The specific sp-/sp2-cohybridized structure of GDY endows it with many unique and fascinating properties that are superior to traditional carbon materials, e.g., the presence of abundant carbon chemical bonds, large conjugated π structures, natural cavities, a favourable band gap, etc. More attractively, the highly uneven distribution of surface charges and incomplete charge transfer between GDY and metal atoms can produce more active sites and a higher intrinsic activity, and efficiently regulate the adsorption/desorption behaviour of reaction intermediates on active site surfaces. Another unique property of GDY is that its controlled growth on arbitrary substrates can be carried out under ambient conditions, which shows great advantages in the controlled synthesis of high-performance interface structures for catalysis.15–21 GDY has brought new opportunities for transformative breakthroughs in many fields, including catalysis, energy, photoelectric conversion devices, intelligent information systems, life sciences, and so on.22–45 These advantages make GDY an ideal material for the synthesis of highly active and selective catalysts for the CO2RR.
In this work, we report the controlled synthesis of GDY/CuSx heterostructured catalysts by using the advantage of GDY in that it can be grown on arbitrary substrate surfaces. Experimental results show that the incomplete charge transfer between GDY and copper atoms and the GDY-induced formation of mixed-valence Cu species can produce more active sites and improve the catalytic selectivity and activity for the CO2RR at room temperatures and ambient pressures.
2. Experimental section
2.1 Materials
Tetrahydrofuran, ethyl acetate, acetone, sodium chloride, N,N,N′,N′-tetramethylethylenediamine, pyridine, and thiourea were purchased from Beijing Reagent Company. The copper foam was cleaned using acetone, 3 M HCl, deionized water, and ethanol before use. All organic reagents are analytical reagents.
2.2 Catalyst synthesis
Synthesis of CuSx. Freshly cleaned copper foam was placed in a stainless-steel autoclave containing 10 mL thiourea solution (1.445 mmol L−1) and heated at 150 °C for 5 hours. After completion of the reaction, the sample was washed three times with ethanol and dried under vacuum.
Synthesis of GDY/CuSx. The obtained CuSx was placed in a reactor containing 6 mg mL−1 hexaethynylbenzene (HEB), 10 mL ethyl acetate, 10 mL dichloromethane and 1 mL pyridine for seven days. After completion of the reaction, the sample was washed thoroughly with dichloromethane and acetone and dried under vacuum.
Results and discussion
Fig. 1a shows the synthesis route for GDY/CuSx. Using copper foam as the substrate, a three-dimensional porous CuSx electrode was obtained through a simple hydrothermal reaction. The obtained CuSx electrode was then used directly as the substrate for the in situ growth of GDY via a cross-coupling reaction to synthesize the GDY/CuSx heterostructure. Scanning electron microscopy (SEM) images (Fig. 1b and c) show that the surface of the copper foam (Fig. S1, ESI†) changed from smooth to porous with a granular distribution on the surface of the copper foam. Energy dispersive spectroscopy mapping of CuSx (Fig. 1d) shows that the surface element components are Cu element and S element, which preliminarily confirms the successful synthesis of CuSx. CuSx acts as a catalyst for the cross-coupling reaction and a growth substrate for the controlled growth of GDY. After the Glaser–Hay coupling reaction, a film of GDY nanosheets with a three-dimensional porous morphology was formed on the surface of the CuSx electrode (Fig. 1e and f). The cross-section image (Fig. 1g) further confirmed the successful growth of the GDY film on the surface of copper sulfide. X-ray energy dispersive spectra for GDY/CuSx (Fig. 1h–j) reveal the presence and uniform distribution of C, Cu, and S elements in the catalyst.
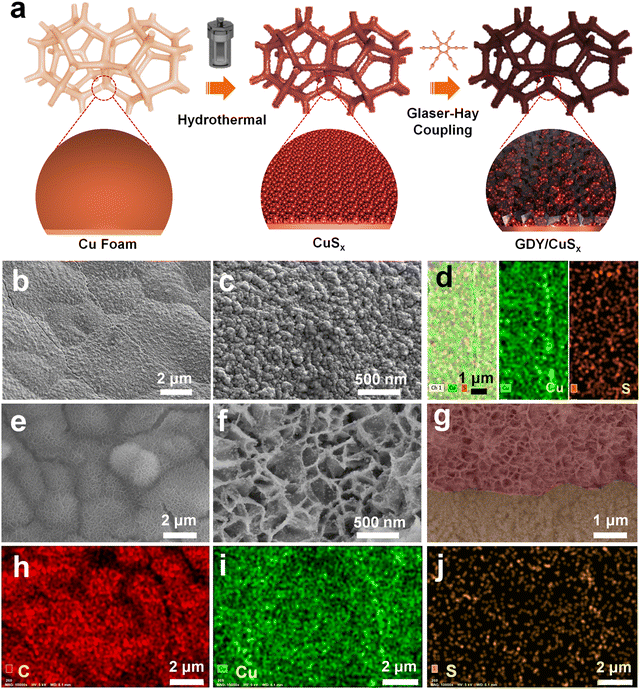 |
| Fig. 1 (a) Schematic representation of the synthesis route of GDY/CuSx; and (b) low- and (c) high-magnification SEM images of CuSx. (d) Energy dispersive spectroscopy mapping of CuSx. (e) Low- and (f) high-magnification SEM images of GDY/CuSx. (g) Cross-section SEM image of GDY/CuSx; and (h, i and j) energy dispersive spectroscopy mapping of GDY/CuSx. | |
The morphology of the samples was next characterized using transmission electron microscopy (TEM). As shown in Fig. 2a and b, the pure GDY electrode has a three-dimensional porous structure comprised of two-dimensional GDY nanosheets. The high-resolution TEM image (Fig. 2c) shows that the spacing distance of GDY is 0.335 nm. The elemental mapping images (Fig. 2d) show the uniform distribution of elemental C over the GDY nanosheets. For GDY/CuSx, the three-dimensional porous morphology was well maintained (Fig. 2e) with nanoparticles uniformly distributed on the surface of GDY (Fig. 2f–h). The HRTEM image (Fig. 2i) shows that the growth of GDY on the CuSx surface had occurred. The elemental mapping results of the GDY/CuSx heterostructure (Fig. 2j–m) reveal the uniform distribution of the Cu, S, and C elements in the heterojunction catalyst. Moreover, full XPS survey spectra confirms the presence of C, Cu, S, and O elements in the sample (ESI,† Fig. S2) once again demonstrating the successful construction of the heterojunction catalyst.
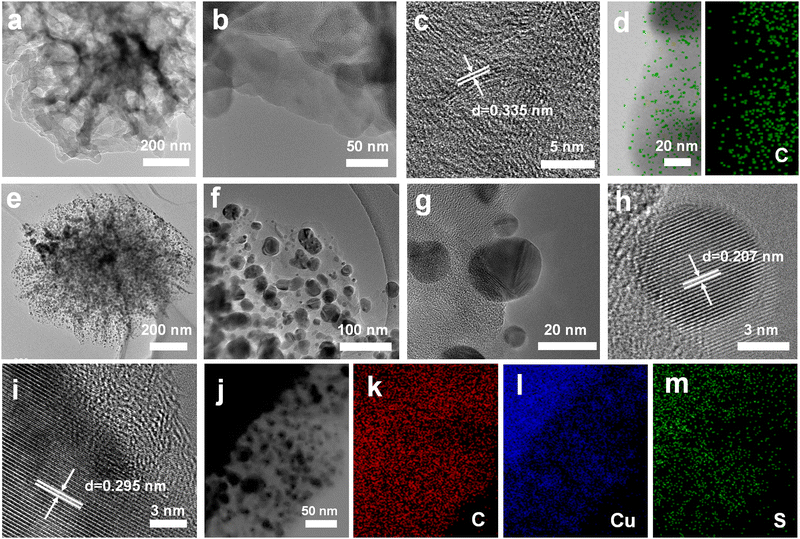 |
| Fig. 2 (a) TEM and (b and c) HRTEM images of the GDY nanosheet. (d) STEM image (left) and corresponding elemental mapping (right) of the elemental C of GDY. (e–g) TEM and (h and i) HRTEM images of GDY/CuSx, (j) STEM image and corresponding elemental mapping of (k) C, (l) Cu, and (m) S elements of the GDY/CuSx electrode. | |
XPS measurements were performed to determine the chemical structures of the samples. As shown in Fig. 3a, four peaks at 932.7/933.6 eV and 952.8/953.7 eV, which correspond to the +1 and +2 valences in Cu 2p3/2 and Cu 2p1/2, respectively, were observed from the high-resolution Cu 2p XPS spectra, revealing the mixed valence of copper in the GDY/CuSx catalyst. Besides, the Cu 2p spectra of GDY/CuSx showed a positive shift of 0.4 eV in binding energy compared with pure copper sulfide, which indicates the loss of electrons from Cu. For the C 1s XPS spectra (Fig. 3b), four characteristic peaks at 284.5 eV, 285.0 eV, 286.9 eV, and 288.5 eV, corresponding to C–C (sp2-C), C–C (sp-C), C–O, and C
O, respectively, were observed. The area ratio of sp2-C to sp-C peaks (0.5) for GDY/CuSx is consistent with that of pure GDY. Compared with pure GDY, the peak of sp-C in GDY/CuSx showed a negative shift of 0.2 eV, indicating that sp-C receives electrons. Fig. 3c shows the S 2p XPS spectra of the samples. The peak positions at 162.3 and 163.6 eV in the figure correspond to the two peaks of S2−2p3/2 and S2−2p1/2, respectively. The XPS results demonstrate the incomplete charge transfer between GDY and metal copper atoms, which is beneficial for enhancing the catalytic activity. The Raman spectra (Fig. 3d) show both the characteristic peaks of CuSx and the characteristic peaks of graphdiyne, which further proves that the GDY/CuSx heterojunction catalyst was successfully synthesized.
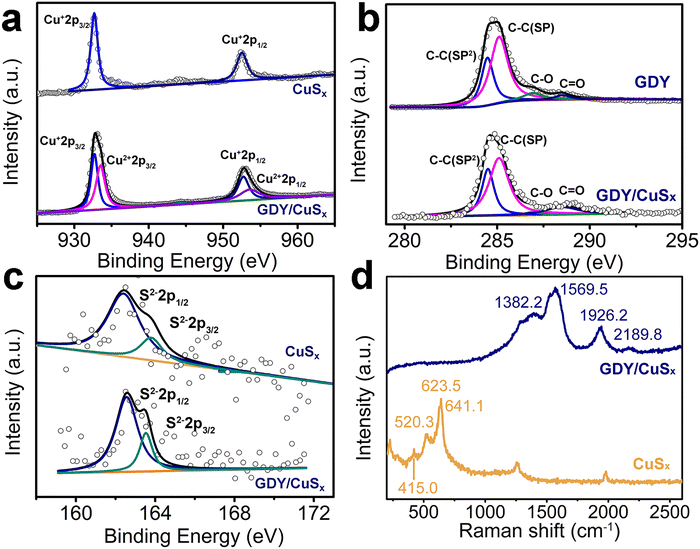 |
| Fig. 3 High-resolution (a) Cu 2p, (b) C 1s, and (c) S 2p XPS spectra of CuSx and GDY/CuSx. (d) Raman spectra of CuSx and GDY/CuSx. | |
A three-electrode system was applied to test the electrocatalytic CO2RR performance to formic acid in 0.1 M KHCO3 electrolyte. Fig. 4a shows the linear sweep voltammetry (LSV) curves of the as-prepared samples. The current density of the GDY/CuSx electrode in the CO2-saturated 0.1 M KHCO3 electrolyte is significantly larger than that in the Ar-saturated 0.1 M KHCO3 electrolyte, which indicates that the GDY/CuSx electrode has a clear carbon dioxide reduction ability. At the same potentials, the current density for GDY/CuSx was larger than that of CuSx. These results confirm that the introduction of GDY can significantly improve the CO2RR activity of the catalyst. The electrolyte after the electrocatalytic CO2RR at different voltages was analysed using NMR (Fig. 4b and c). The faradaic efficiency values of the gas phase products and liquid phase products of GDY/CuSx under different bias voltages are shown in Fig. 4d. It was observed that the gas phase products were mainly hydrogen. Fig. 4e shows the potential-dependent FE and total current density of the CO2RR to formic acid. GDY/CuSx exhibits a high FE for formic acid production of 70% at −0.9 V vs. RHE, with a total current density reaching −65.6 mA cm−2, which are better than that of pure CuSx samples at the same potential (Fig. S3, ESI†). Overall, the side reaction of CO and hydrocarbon production has been significantly inhibited under the bias voltage of −0.9 V vs. RHE. Such a catalytic performance could be maintained over a 4 hour period (Fig. 4f). SEM images for the samples obtained after the stability test show that there is almost no variation in the morphology (Fig. S4, ESI†). In situ ATR-FTIR characterization was employed to identify the chemical structure of the intermediates involved during the electrochemical CO2RR. As shown in Fig. 4g, after subtracting the background of the open circuit potential infrared spectrum, the intensity of three bands of 1640, 2100, and 2340 cm−1 can be observed. The strong positive band in the 1640 cm−1 region can be assigned to interfacial H2O, which accumulates in the electrocatalyst due to catalysis or the increasing negative polarization of the electrode.46 The positive strong band in the 2340 cm−1 region is attributed mainly to the adsorption of CO2 on the electrode surface.47 The positive band located in the 2100 cm−1 region observed between −0.7 V and −1.5 V vs. RHE corresponds to the adsorbed CO (COads) molecules and adsorbed carbonate on the electrode surface.48–50 During the electrolysis process, the CO product ratio was low, which indicates that COads was tightly bound to the surface with a low desorption rate. In Fig. 4h, the bands at 1544 and 1420 cm−1, corresponding to the desorption of carbonate and the desorption of *COOH (carboxylate)/HCOO* (formate),51 are absent. The absence of the HCOO* and COads adsorption bands and the low selectivity for CO indicate that the main formation mechanism of formate is mainly through the reaction of physiosorbed CO2 with Hads (CO2 + Hads + e− → HCOO−).49,52 The Pourbaix diagram of the Cu–S system calculated by Liu et al.53 indicated that S is unable to exist stably at the potential of formic acid production and will dissolve in the electrolyte.49,53 Some monodisperse S will remain, and this residual S exists in substitutive form during the CO2RR due to the sluggish reaction kinetics.52,54,55 These residual S species were supposed to be effective on CO* adsorption, so that specific surface reaction sites are blocked, and a solution-phase CO2 reduction pathway occurs for highly selective HCOOH production. The CO2-to-HCOOH performance reported is better than most other Cu-based catalysts (Fig. 4i). The unique incomplete charge transfer between GDY and metal atoms, and the high CO2 affinity of GDY, can effectively increase the number of active sites and regulate the adsorption/desorption capacity of key reaction intermediates, achieving an efficient and stable CO2-to-HCOOH conversion (Fig. 5).
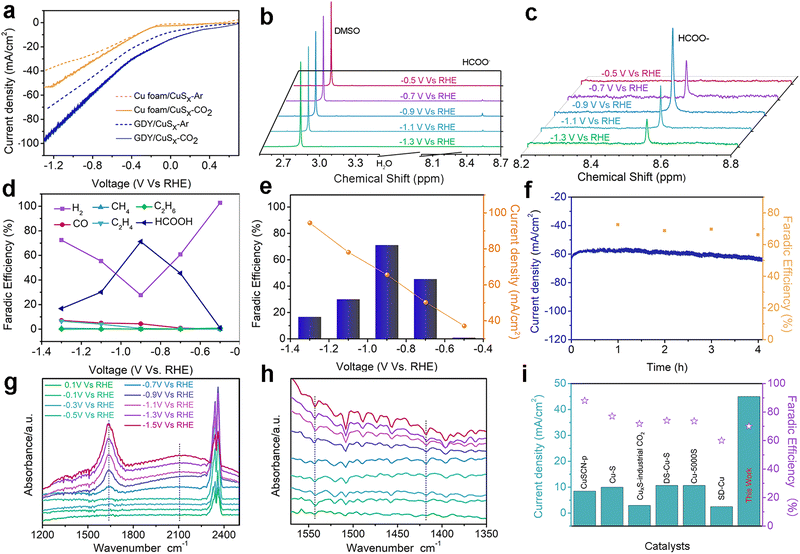 |
| Fig. 4 (a) Linear sweep voltammetry of CuSx and GDY/CuSx recorded in CO2- and Ar- saturated electrolytes. (b and c) 1H NMR spectra of the electrolyte after the CO2RR at the GDY/CuSx electrode at different applied potentials. (d) Potential–faradaic efficiency (FE) curves for the products (HCOOH, H2, CO, C2H4, and C2H6) produced during the CO2RR on GDY/CuSx. (e) Plot of FE and current density of GDY/CuSxvs. the potential. (f) Stability and the corresponding faradaic efficiency for HCOOH produced with GDY/CuSx at −0.9 V vs. RHE in CO2-saturated 0.1 M KHCO3. (g and h) Potential-dependent in situ ATR-FTIR spectra of the GDY/CuSx electrode with CO2 purging. (i) Comparison between GDY/CuSx and reported Cu-based electrodes for the CO2RR. | |
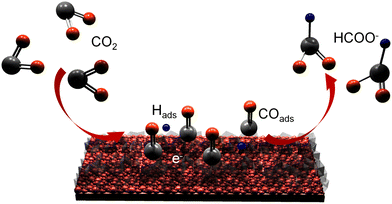 |
| Fig. 5 Proposed reaction pathway of CO2-to-HCOOH conversion. | |
Conclusions
In summary, we have developed an active CO2-to-HCOOH heterostructured electrocatalyst of GDY/CuSxvia the in situ growth of GDY on the surface of CuSx. The incomplete charge transfer between GDY and Cu atoms improved the catalyst conductivity, produced more active sites, and ultimately improved the catalytic performance. The faradaic efficiency of carbon dioxide reduction to formic acid reaches 70%, and a total current density of 65.6 mA cm−2 at −0.9 V vs. RHE is achieved. This work provides an effective strategy to enhance the selective catalysis of formate over low-cost Cu-based catalysts and expands the material options to produce this commercially valuable fuel and chemical.
Author contributions
Y. Li, Y. Xue, and S. Cao conceived the experiments. S. Cao performed the experiments. S. Cao and Y. Xue wrote the manuscript. Y. Li revised the manuscript. X. Chen, C. Zhang and Y. Gao helped with the electrochemical test and the in situ ATR-FTIR. All the authors discussed the results and commented on the manuscript.
Conflicts of interest
There are no conflicts to declare.
Acknowledgements
The authors acknowledge funding from the National Key Research and Development Project of China (2018YFA0703501), the National Natural Science Foundation of China (22021002), the Key Program of the Chinese Academy of Sciences (XDPB13), and the Beijing National Laboratory for Molecular Sciences (2020BMS20040).
References
- T. J. Battin, S. Luyssaert, L. A. Kaplan, A. K. Aufdenkampe, A. Richter and L. J. Tranvik, The boundless carbon cycle, Nat. Geosci., 2009, 2, 598–600 CrossRef CAS.
- M. Reichstein, M. Bahn, P. Ciais, D. Frank, M. D. Mahecha, S. I. Seneviratne, J. Zscheischler, C. Beer, N. Buchmann, D. C. Frank, D. Papale, A. Rammig, P. Smith, K. Thonicke, M. van der Velde, S. Vicca, A. Walz and M. Wattenbach, Climate extremes and the carbon cycle, Nature, 2013, 500, 287–295 CrossRef CAS PubMed.
- N. Mac Dowell, P. S. Fennell, N. Shah and G. C. Maitland, The role of CO2 capture and utilization in mitigating climate change, Nat. Clim. Change, 2017, 7, 243–249 CrossRef CAS.
- C. Liu, B. C. Colon, M. Ziesack, P. A. Silver and D. G. Nocera, Water splitting-biosynthetic system with CO2 reduction efficiencies exceeding photosynthesis, Science, 2016, 352, 1210–1213 CrossRef CAS PubMed.
- R. Sun, Y. Liao, S.-T. Bai, M. Zheng, C. Zhou, T. Zhang and B. F. Sels, Heterogeneous catalysts for CO2 hydrogenation to formic acid/formate: from nanoscale to single atom, Energy Environ. Sci., 2021, 14, 1247–1285 RSC.
- M. Grasemann and G. Laurenczy, Formic acid as a hydrogen source – recent developments and future trends, Energy Environ. Sci., 2012, 5, 8171–8181 RSC.
- B. Loges, A. Boddien, H. Junge and M. Beller, Controlled generation of hydrogen from formic acid amine adducts at room temperature and application in H2/O2 fuel cells, Angew. Chem., Int. Ed., 2008, 47, 3962–3965 CrossRef CAS PubMed.
- P. Saha, S. Amanullah and A. Dey, Selectivity in electrochemical CO2 reduction, Acc. Chem. Res., 2022, 55, 134–144 CrossRef CAS PubMed.
- S. Zhang, Q. Fan, R. Xia and T. J. Meyer, CO2 reduction:from homogeneous to heterogeneous electrocatalysis, Acc. Chem. Res., 2020, 53, 255–264 CrossRef CAS PubMed.
- O. S. Bushuyev, P. De Luna, C. T. Dinh, L. Tao, G. Saur, J. van de Lagemaat, S. O. Kelley and E. H. Sargent, What should we make with CO2 and how can we make it?, Joule, 2018, 2, 825–832 CrossRef CAS.
- A. G. A. Mohamed, E. Zhou, Z. Zeng, J. Xie, D. Gao and Y. Wang, Asymmetric Oxo-bridged ZnPb bimetallic electrocatalysis boosting CO2 -to-HCOOH reduction, Adv. Sci., 2022, 9, e2104138 CrossRef PubMed.
- J. Wang, T. Xia, L. Wang, X. Zheng, Z. Qi, C. Gao, J. Zhu, Z. Li, H. Xu and Y. Xiong, Enabling visible-light-driven selective CO2 reduction by doping quantum dots: trapping electrons and suppressing H2 evolution, Angew. Chem., Int. Ed., 2018, 57, 16447–16451 CrossRef CAS PubMed.
- Z. Chen, K. Mou, X. Wang and L. Liu, Nitrogen-doped graphene quantum dots enhance the activity of Bi2O3 nanosheets for electrochemical reduction of CO2 in a wide negative potential region, Angew. Chem., Int. Ed., 2018, 57, 12790–12794 CrossRef CAS PubMed.
- H. Luo, B. Li, J. G. Ma and P. Cheng, Surface modification of nano-Cu2O for controlling CO2 electrochemical reduction to ethylene and syngas, Angew. Chem., Int. Ed., 2022, 61, e202116736 CAS.
- G. Li, Y. Li, H. Liu, Y. Guo, Y. Li and D. Zhu, Architecture of graphdiyne nanoscale films, Chem. Commun., 2010, 46, 3256–3258 RSC.
- F. He and Y. Li, Advances on theory and experiments of the energy applications in graphdiyne, CCS Chem., 2022, 0, 1–23 Search PubMed.
- C. Huang, Y. Li, N. Wang, Y. Xue, Z. Zuo, H. Liu and Y. Li, Progress in research into 2D graphdiyne-based materials, Chem. Rev., 2018, 118, 7744–7803 CrossRef CAS PubMed.
- Y. Fang, Y. Liu, L. Qi, Y. Xue and Y. Li, 2D graphdiyne: an emerging carbon material, Chem. Soc. Rev., 2022, 51, 2681–2709 RSC.
- X. Gao, H. Liu, D. Wang and J. Zhang, Graphdiyne: synthesis, properties, and applications, Chem. Soc. Rev., 2019, 48, 908–936 RSC.
- R. Matsuoka, R. Sakamoto, K. Hoshiko, S. Sasaki, H. Masunaga, K. Nagashio and H. Nishihara, Crystalline graphdiyne nanosheets produced at a gas/liquid or liquid/liquid interface, J. Am. Chem. Soc., 2017, 139, 3145–3152 CrossRef CAS PubMed.
- Z. Zheng, Y. Xue and Y. Li, A new carbon allotrope: graphdiyne, Trends Chem., 2022, 4, 754–768 CrossRef CAS.
- Y. Liu, Y. Gao, F. He, Y. Xue and Y. Li, Controlled growth interface of charge transfer salts of nickel-7,7,8,8-tetracyanoquinodimethane on surface of graphdiyne, CCS Chem., 2022, 0, 1–11 Search PubMed.
- Y. Gao, Y. Xue, L. Qi, C. Xing, X. Zheng, F. He and Y. Li, Rhodium nanocrystals on porous graphdiyne for electrocatalytic hydrogen evolution from saline water, Nat. Commun., 2022, 13, 5227 CrossRef CAS PubMed.
- Y. Gao, Y. Xue, F. He and Y. Li, Controlled growth of a high selectivity interface for seawater electrolysis, Proc. Natl. Acad. Sci. U. S. A., 2022, 119, e2206946119 CrossRef CAS PubMed.
- H. Yu, Y. Xue, L. Hui, C. Zhang, Y. Fang, Y. Liu, X. Chen, D. Zhang, B. Huang and Y. Li, Graphdiyne-based metal atomic catalysts for synthesizing ammonia, Natl. Sci. Rev., 2021, 8, nwaa213 CrossRef CAS PubMed.
- Y. Fang, Y. Xue, L. Hui, H. Yu and Y. Li, Graphdiyne@Janus magnetite for photocatalytic nitrogen fixation, Angew. Chem., Int. Ed., 2021, 60, 3170–3174 CrossRef CAS PubMed.
- H. Yu, Y. Xue, L. Hui, C. Zhang, Y. Li, Z. Zuo, Y. Zhao, Z. Li and Y. Li, Efficient hydrogen production on a 3D flexible heterojunction material, Adv. Mater., 2018, 30, e1707082 CrossRef PubMed.
- L. Hui, Y. Xue, H. Yu, Y. Liu, Y. Fang, C. Xing, B. Huang and Y. Li, Highly efficient and selective generation of ammonia and hydrogen on a graphdiyne-based catalyst, J. Am. Chem. Soc., 2019, 141, 10677–10683 CrossRef CAS PubMed.
- Y. Xue, B. Huang, Y. Yi, Y. Guo, Z. Zuo, Y. Li, Z. Jia, H. Liu and Y. Li, Anchoring zero valence single atoms of nickel and iron on graphdiyne for hydrogen evolution, Nat. Commun., 2018, 9, 1460 CrossRef PubMed.
- Y. Fang, Y. Xue, Y. Li, H. Yu, L. Hui, Y. Liu, C. Xing, C. Zhang, D. Zhang, Z. Wang, X. Chen, Y. Gao, B. Huang and Y. Li, Graphdiyne interface engineering: highly active and selective ammonia synthesis, Angew. Chem., Int. Ed., 2020, 59, 13021–13027 CrossRef CAS PubMed.
- Z. Wang, Z. Zheng, Y. Xue, F. He and Y. Li, Acidic water oxidation on quantum dots of IrOx/graphdiyne, Adv. Energy Mater., 2021, 11, 2101138 CrossRef CAS.
- Y. Du, W. Zhou, J. Gao, X. Pan and Y. Li, Fundament and application of graphdiyne in electrochemical energy, Acc. Chem. Res., 2020, 53, 459–469 CrossRef CAS PubMed.
- Y. Xue, Y. Li, J. Zhang, Z. Liu and Y. Zhao, 2D graphdiyne materials: challenges and opportunities in energy field, Sci. China: Chem., 2018, 61, 765–786 CrossRef CAS.
- Z. Zuo, D. Wang, J. Zhang, F. Lu and Y. Li, Synthesis and applications of graphdiyne-based metal-free catalysts, Adv. Mater., 2019, 31, e1803762 CrossRef PubMed.
- Y. Xue, L. Hui, H. Yu, Y. Liu, Y. Fang, B. Huang, Y. Zhao, Z. Li and Y. Li, Rationally engineered active sites for efficient and durable hydrogen generation, Nat. Commun., 2019, 10, 2281 CrossRef PubMed.
- C. Xing, Y. Xue, B. Huang, H. Yu, L. Hui, Y. Fang, Y. Liu, Y. Zhao, Z. Li and Y. Li, Fluorographdiyne: A metal-free catalyst for applications in water reduction and oxidation, Angew. Chem., Int. Ed., 2019, 58, 13897–13903 CrossRef CAS PubMed.
- Z. Zheng, L. Qi, Y. Xue and Y. Li, Highly selective and durable of monodispersed metal atoms in ammonia production, Nano Today, 2022, 43, 101431 CrossRef CAS.
- X. Zheng, Y. Xue, C. Zhang and Y. Li, Controlled growth of multidimensional interface for high-selectivity ammonia production, CCS Chem., 2022, 0, 1–10 Search PubMed.
- X. Luan, Z. Zheng, S. Zhao, Y. Xue and Y. Li, Controlled growth of the interface of CdWOx/GDY for hydrogen energy conversion, Adv. Funct. Mater., 2022, 32, 2202843 CrossRef CAS.
- L. Qi, Z. Zheng, C. Xing, Z. Wang, X. Luan, Y. Xue, F. He and Y. Li, 1D nanowire heterojunction electrocatalysts of MnCo2O4/GDY for efficient overall water splitting, Adv. Funct. Mater., 2021, 32, 2107179 CrossRef.
- Y. Zhao, J. Wan, H. Yao, L. Zhang, K. Lin, L. Wang, N. Yang, D. Liu, L. Song, J. Zhu, L. Gu, L. Liu, H. Zhao, Y. Li and D. Wang, Few-layer graphdiyne doped with sp-hybridized nitrogen atoms at acetylenic sites for oxygen reduction electrocatalysis, Nat. Chem., 2018, 10, 924–931 CrossRef CAS PubMed.
- K. Ma, J. Wu, X. Wang, Y. Sun, Z. Xiong, F. Dai, H. Bai, Y. Xie, Z. Kang and Y. Zhang, Periodically interrupting bonding behavior to reformat delocalized electronic states of graphdiyne for improved electrocatalytic hydrogen evolution, Angew. Chem., Int. Ed., 2022, 61, e202211094 CAS.
- X. Gao, J. Zhou, R. Du, Z. Xie, S. Deng, R. Liu, Z. Liu and J. Zhang, Robust superhydrophobic foam: a graphdiyne-based hierarchical architecture for oil/water separation, Adv. Mater., 2016, 28, 168–173 CrossRef CAS PubMed.
- Y. Y. Han, X. L. Lu, S. F. Tang, X. P. Yin, Z. W. Wei and T. B. Lu, Metal-free 2D/2D heterojunction of graphitic carbon nitride/graphdiyne for improving the hole mobility of graphitic carbon nitride, Adv. Energy Mater., 2018, 8, 1702992 CrossRef.
- J. Li, X. Gao, B. Liu, Q. Feng, X. B. Li, M. Y. Huang, Z. Liu, J. Zhang, C.-H. Tung and L.-Z. Wu, Graphdiyne: a metal-free material as hole transfer layer to fabricate quantum dot-sensitized photocathodes for hydrogen production, J. Am. Chem. Soc., 2016, 138, 3954–3957 CrossRef CAS PubMed.
- H. Zhong, M. Ghorbani-Asl, K. H. Ly, J. Zhang, J. Ge, M. Wang, Z. Liao, D. Makarov, E. Zschech, E. Brunner, I. M. Weidinger, J. Zhang, A. V. Krasheninnikov, S. Kaskel, R. Dong and X. Feng, Synergistic electroreduction of carbon dioxide to carbon monoxide on bimetallic layered conjugated metal-organic frameworks, Nat. Commun., 2020, 11, 1409 CrossRef CAS PubMed.
- E. R. Corson, R. Kas, R. Kostecki, J. J. Urban, W. A. Smith, B. D. McCloskey and R. Kortlever, In situ ATR–SEIRAS of carbon dioxide reduction at a plasmonic silver cathode, J. Am. Chem. Soc., 2020, 142, 11750–11762 CrossRef CAS PubMed.
- S. Zhu, T. Li, W.-B. Cai and M. Shao, CO2 electrochemical reduction as probed through infrared spectroscopy, ACS Energy Lett., 2019, 4, 682–689 CrossRef CAS.
- K. R. Phillips, Y. Katayama, J. Hwang and Y. Shao-Horn, Sulfide-derived copper for electrochemical conversion of CO2 to formic acid, J. Phys. Chem. Lett., 2018, 9, 4407–4412 CrossRef CAS PubMed.
- J. Heyes, M. Dunwell and B. Xu, CO2 reduction on Cu at low overpotentials with surface-enhanced in situ spectroscopy, J. Phys. Chem. C, 2016, 120, 17334–17341 CrossRef CAS.
- W. Deng, L. Zhang, L. Li, S. Chen, C. Hu, Z.-J. Zhao, T. Wang and J. Gong, Crucial role of surface hydroxyls on the activity and stability in electrochemical CO2 reduction, J. Am. Chem. Soc., 2019, 141, 2911–2915 CrossRef CAS PubMed.
- T. Cheng, H. Xiao and W. A. Goddard, III, Reaction mechanisms for the electrochemical reduction of CO2 to CO and formate on the Cu(100) surface at 298 K from quantum mechanics free energy calculations with explicit water, J. Am. Chem. Soc., 2016, 138, 13802–13805 CrossRef CAS PubMed.
- D. Liu, Y. Liu and M. Li, Understanding how atomic sulfur controls the selectivity of the electroreduction of CO2 to formic acid on metallic Cu surfaces, J. Phys. Chem. C, 2020, 124, 6145–6153 CrossRef CAS.
- Y. Deng, Y. Huang, D. Ren, A. D. Handoko, Z. W. Seh, P. Hirunsit and B. S. Yeo, On the role of sulfur for the selective electrochemical reduction of CO2 to formate on CuSx catalysts, ACS Appl. Mater. Interfaces, 2018, 10, 28572–28581 CrossRef CAS PubMed.
- J. S. Yoo, R. Christensen, T. Vegge, J. K. Norskov and F. Studt, Theoretical insight into the trends that guide the electrochemical reduction of carbon dioxide to formic acid, ChemSusChem, 2016, 9, 358–363 CrossRef CAS PubMed.
|
This journal is © the Partner Organisations 2023 |