DOI:
10.1039/D2TB00263A
(Paper)
J. Mater. Chem. B, 2022,
10, 6752-6757
Fluorescence detection of milk allergen β-lactoglobulin based on aptamers and WS2 nanosheets†
Received
5th February 2022
, Accepted 30th March 2022
First published on 30th March 2022
Abstract
β-Lactoglobulin (β-Lg), a food allergen, can easily cause allergic reactions in infants and young children. Therefore, it is necessary to develop a rapid, sensitive, and selective detection method to protect individuals prone to allergies. In this paper, a fluorescence assay based on WS2 nanosheets and a fluorescent dye (FAM)-labeled β-Lg aptamer was designed to detect β-Lg rapidly with high sensitivity. In the sensing platform, the β-Lg aptamer is adsorbed on the WS2 nanosheet surface by van der Waals forces, which trigger the phenomenon of fluorescence resonance energy transfer (FRET) and suppress the fluorescence signal in the system. When β-Lg is present, the conformation of the aptamer specifically bound to β-Lg changes. Therefore, the aptamer is separated from the WS2 nanosheet surface, and the fluorescence signal is recovered. This method combines the high quenching efficiency of WS2 nanosheets and good specificity of the β-Lg aptamer. The detection range of this method for β-Lg is 0.1–100 μg mL−1. The detection limit is 20.4 ng mL−1. This method exhibits high sensitivity, selectivity and good reproducibility, and it can be used for β-Lg detection in actual samples.
Introduction
Food allergy reflects the response of the human immune system to certain ingredients in food, which can result in various skin, gastrointestinal and respiratory diseases, and even endanger human life in severe cases.1 In 1995, milk, eggs, peanuts, nuts, wheat, soybeans, fish, and shellfish were recognized by the Food and Agriculture Organization of the United Nations (FAO) as the eight major food categories that cause food allergies.2 Milk is rich in protein, mainly casein and whey protein. Whey protein consists of β-lactoglobulin (β-Lg), α-lactalbumin (α-La), lactoferrin, bovine serum albumin (BSA), and immunoglobulin.3 β-Lg accounts for about 12% of the total milk protein and 50% of the whey protein, and its concentration in milk is 2–4 g L−1.4 Meanwhile, β-Lg is widespread in foods due to its high nutritional value and multiple functions, e.g., foaming, gelation, binding properties, emulsification, and food additives.5,6 However, many research studies have shown that proteins in milk can lead to allergic reactions in 2% to 3% of infants and young children, and that 60% to 80% of milk allergies are caused by β-Lg.7,8 Although food labelling laws and regulations of most countries stipulate that the common allergens must be declared in the ingredient list of food packaging,9 it is still difficult to avoid the mixing of allergens in food processing and packaging. Therefore, it is necessary to establish an effective β-Lg detection strategy to protect public health.
To date, many analytical methods have been developed to detect β-Lg. Traditional chromatography is commonly used for β-Lg monitoring, including high performance liquid chromatography (HPLC),10 ultra-high performance liquid chromatography (UHPLC),11 and liquid chromatography tandem mass spectrometry (LC-MS/MS).12 In addition, some novel detection methods, such as enzyme-linked immunosorbent assay,13 photoelectrochemical immunoassay,14 lateral flow immunoassay,15 real-time quantitative polymerase chain reaction,16 transient isotachophoresis,17 and molecular imprinting,18 have also been used for the detection of β-Lg. Although these reported methods have exhibited very good detection sensitivity and accuracy, there are still some disadvantages, such as the need for expensive analytical equipment and complex sample pre-treatment processes. For instance, immunoassay is costly and requires long analysis time, and the stability of antibodies is very difficult to maintain. A DNA-based polymerase chain reaction relies on specific sequences, and the equipment is expensive and the procedure is time consuming.
Aptamers are single-stranded DNA or RNA selected from a random sequence nucleic acid library through an exponential enrichment (SELEX) process, and they specifically bind to target molecules.19 Compared with antibodies, aptamers possess the advantages of low cost, high stability, easy synthesis, and labelling.20 Meanwhile, owing to their obvious affinity for most biomolecules, many electrochemical, colorimetric, and fluorescence methods have been extensively studied in the field of aptamer-based biomolecule detection.21,22 Among them, fluorescence-based detection methods have attracted much attention due to their simplicity, speed, low cost, and high sensitivity, and been widely used in protein detection.23,24 So far, there have been few fluorescence methods based on aptamers to detect β-Lg.25,26
In recent years, transition metal chalcogenides (e.g., MoS2 and WS2) have been discovered and studied as two-dimensional (2D) nanomaterials similar to graphene oxide.27,28 Compared with graphene oxide, transition metal chalcogenide nanosheets are easier to synthesize on a large scale, and can be directly dispersed in an aqueous solution without any surfactants.29–32 WS2 nanosheets can recognize single-stranded DNA (ssDNA) and double-stranded DNA (dsDNA), due to the presence of van der Waals forces between the cyclic bases and WS2 nanosheets.33 As a result, single-stranded DNA (ssDNA) can be adsorbed on the WS2 nanosheet surface. This feature has been applied to many fluorescence analyses34–36 and makes WS2 nanosheets an ideal material for designing a fluorescence assay for β-Lg detection.
In this work, we exploited WS2 nanosheets to construct a fluorescence assay based on aptamer fluorescence quenching and recovery to detect β-Lg. In this sensing system, when there is no target, the aptamer labelled with a fluorescent dye-FAM is adsorbed on the WS2 nanosheets by van der Waals forces. The fluorescence resonance energy transfer (FRET) phenomenon occurs between the dye molecule and the WS2 nanosheets, resulting in fluorescence quenching. However, when the target β-Lg is present, the aptamer specifically binds to the target, inducing aptamers to form a more rigid conformation. This rigid conformation weakens the van der Waals forces between aptamers and WS2 nanosheets. Therefore, the aptamer labelled with FAM is released from the nanosheet surface, and the fluorescence signal is restored (Fig. 1). This sensing platform exhibits high sensitivity and selectivity for β-Lg detection, and the detection strategy has been successfully applied to actual food products.
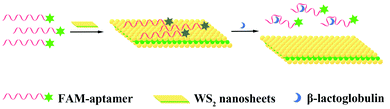 |
| Fig. 1 Schematic diagram for detecting β-Lg based on aptamers and WS2 nanosheets. | |
Experimental
Materials and apparatus
WS2 nanosheets were purchased from Nanjing XF Nano Material Tech Co., Ltd. (Nanjing, China). Bovine serum albumin (BSA), sodium chloride (NaCl), and potassium chloride (KCl) were purchased from Sinopharm Chemical Reagent Co., Ltd (Shanghai, China). Magnesium chloride hexahydrate (MgCl2·6H2O), and γ-globulin were purchased from Aladdin Biochemical Technology Co., Ltd (Shanghai, China). β-Lg, ovalbumin, and α-lactalbumin (α-La) were purchased from Sigma-Aldrich Company (Shanghai, China). Casein from bovine milk, and Tris–HCl were purchased from Shanghai Sangon Biotech Co., Ltd. Pure milk and infant formula samples were purchased from local supermarkets (Xiamen, China). The FAM labelled β-Lg aptamer (5′-FAM-CGACGATCGGACCGCAGTACCCACCCACCAGCCCCAACATCATGCCCATCCGTGTGTG-3′)37 was synthesized and purified by Sangon Biotech Co., Ltd (Shanghai, China). The detection buffer used in this study contained 50 mM Tris–HCl, 5 mM KCl, 100 mM NaCl, and 1 mM MgCl2, at pH 7.4. All chemicals were of analytical grade and used without further purification. All solutions were prepared from ultrapure water obtained from a Milli-Q water purification system (Sartorius, Germany) (resistance > 18.2 MΩ cm). The fluorescence emission spectrum and fluorescence anisotropy were measured on an LS55 fluorescence spectrometer (PerkinElmer Ltd., USA). The UV-vis absorption spectrum was recorded with a Lambda 265 UV-vis spectrophotometer (PerkinElmer Ltd, USA).
Selection of optimum reaction conditions
Typically, 120 μL WS2 nanosheets (200 μg mL−1) and 240 μL β-Lg aptamer (75 nmol L−1) were mixed by shaking and allowed to react at room temperature. In order to determine the quenching time of the β-Lg aptamer by WS2 nanosheets, the fluorescence spectra at different times were recorded. Subsequently, in order to obtain the optimal response time to detect β-Lg, after shaking 120 uL WS2 nanosheets (200 μg mL−1) and 240 μL β-Lg aptamer (75 nmol L−1) at room temperature for 10 min, 120 μL β-Lg (60 μg mL−1) was added to the reaction system. The reaction was carried out at 37 °C on a shaking table. The fluorescence spectra at different reaction times were recorded.
β-Lg detection
Under the optimized conditions, after the β-Lg aptamer and WS2 nanosheets were shaken and reacted at room temperature for 10 min, 120 μL of β-Lg of various concentrations were added to the mixture, and then reacted in a shaker at 37 °C for 1 h. The excitation wavelength was 480 nm. The spectra were recorded between 500 and 600 nm.
Selectivity experiment
Coexisting or similar proteins (α-La, ovalbumin, BSA, casein and γ-globulin) were chosen as interfering substances to evaluate the selectivity. The concentrations of interfering proteins and β-Lg were all 80 μg mL−1. According to the same reaction conditions described above, the effects of those several potential interfering proteins were evaluated.
Detection of β-Lg in food samples
The commercial pure milk and infant formula were pre-treated to avoid the influence of the food matrix on the response of the aptamer. As described in the literature,38 the milk samples were heated at 40 °C for 30 min, centrifuged at 8000 rpm for 20 min and cooled in an ice bath for 15 min. Then, the suspension was acidified with 2 M HCl to pH 4.6 for 20 min. The acidified suspension was further centrifuged at 8000 rpm for 20 min to precipitate casein. The supernatant was collected and filtered with a 0.2 μm polycarbonate membrane. Finally, the solution was neutralized with 1 M NaOH. The pre-treated milk sample was diluted fifty-fold with the detection buffer. For the infant formula sample, 1 g milk powder was dissolved in ultrapure water (5 mL). The dissolved solution was heated to 40 °C and held 30 min; then centrifuged at 15,000 rpm for 15 min.39 The supernatant was acidified to pH 4.6 with 2 M HCl. After 20 min, the supernatant was centrifuged at 8000 rpm for 20 min. The supernatant was collected and filtered with a polycarbonate membrane (size, 0.2 μm). Finally, the solution was neutralized with 1 M NaOH and diluted fifty-fold with the detection buffer. Different concentrations of β-Lg solutions (1, 10, and 100 μg mL−1) were added to the above solution. The determination of β-Lg was carried out according to the steps in β-Lg detection.
Results and discussion
Feasibility study of a fluorescence biosensing platform
First, we investigated the feasibility of the binding state of WS2 nanosheets and the FAM-labelled aptamer through the study of fluorescence anisotropy. Fluorescence anisotropy reflects the rotation ability of fluorescent molecules in the microenvironment. Therefore, it is often used to study the interactions between molecules. The adsorption of aptamers on WS2 nanosheets before and after adding the target β-Lg was investigated by measuring the fluorescence anisotropy of FAM. As shown in Fig. S1 (ESI†), the fluorescence anisotropy (FA) value of the free FAM-labelled β-Lg aptamer in the buffer was 0.149. The value increased to 0.457 after adding WS2 nanosheets, which indicated that the FAM-labelled β-Lg aptamer was assembled on the WS2 nanosheet, limiting the FAM dye rotation. After adding the target β-Lg, the FA value decreased to 0.214, which is close to the FA value of the free FAM-labelled β-Lg aptamer. These results indicated that the β-Lg aptamer adsorbed on the WS2 nanosheet can be dissociated from the nanosheets after the reaction with β-Lg. After that, the FRET phenomenon in the fluorescence quenching process was verified by measuring the emission spectrum of FAM dye and the absorption spectrum of WS2 nanosheets (Fig. 2A). The absorption spectrum was mainly concentrated in the UV region, with the absorption band expanded to the near-infrared range. The overlap of the emission spectrum of the FAM dye and the absorption spectrum of WS2 nanosheets ensured the feasibility of the FRET method.
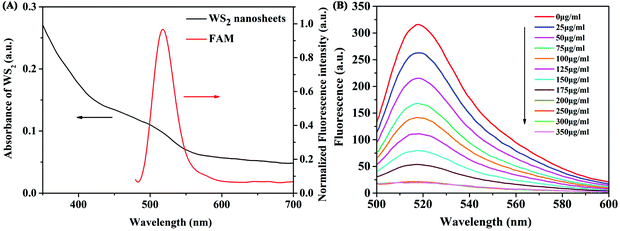 |
| Fig. 2 (A) Spectral overlap between fluorescence emission spectra of FAM fluorescent dye and absorption spectra of WS2 nanosheets. (B) The fluorescence spectra of FAM-labelled β-Lg aptamers incubated with different concentrations of WS2 nanosheets. | |
Optimization of assay conditions for the detection of β-Lg
To acquire the optimal sensing performance, some important parameters were optimized before detecting β-Lg. Because the concentration of WS2 nanosheets used in the experiment has a great influence on the fluorescence quenching reaction, we first optimized the concentration of WS2 nanosheets. As shown in Fig. 2B, as the concentration of WS2 nanosheets increased, the fluorescence intensity of the system gradually decreased. The equation for the fluorescence quenching efficiency is QE = (F0 − Fq)/F0, where F0 is the fluorescence intensity in the system without adding WS2 nanosheets, and Fq is the fluorescence intensity in the system quenched by WS2 nanosheets. When the concentration of WS2 nanosheets was 200 μg mL−1, the quenching efficiency reached 93%. With a further increase of the concentration of WS2 nanosheets, the fluorescence intensity was nearly unchanged. Therefore, 200 μg mL−1 was taken as the optimized concentration, which ensured high quenching efficiency and a low fluorescence background signal.
In addition, by mixing the FAM-labelled β-Lg aptamer with the WS2 nanosheet solution, the quenching kinetics results indicated that the interaction between the aptamer and the WS2 nanosheets was a fairly rapid process, reaching equilibrium within about 10 min (Fig. 3A). Therefore, 10 min was fixed as the reaction time for fluorescence quenching of the aptamer. However, after adding β-Lg to the mixture of the FAM-labelled β-Lg aptamer and WS2 nanosheets, the fluorescence intensity in the reaction system gradually increased, and the fluorescence recovery equilibrium was reached after 60 min (Fig. 3B).
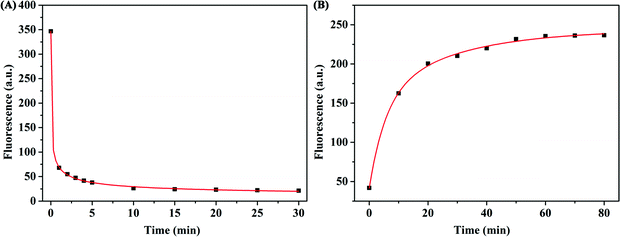 |
| Fig. 3 (A) The fluorescence quenching effect of WS2 nanosheets on FAM-labelled β-Lg aptamer changes with different reaction times. (B) Time-dependent fluorescence changes of the FAM-labelled β-Lg aptamer after adding β-Lg. | |
Detection of β-Lg
Under the optimized detection conditions, a fluorescence assay based on WS2 nanosheets and the β-Lg aptamer was established to detect β-Lg. The changes of fluorescence spectra were measured after adding different concentrations of β-Lg to FAM-complexes of the labelled β-Lg aptamer and WS2 nanosheets. As shown in Fig. 4A, with increasing concentration of the target β-Lg, the fluorescence intensity of the FAM-labelled β-Lg aptamer and WS2 nanosheet complexes gradually increased. The recovery of the fluorescence signal was due to the strong affinity of the aptamer for β-Lg, which caused the aptamer to desorb from the WS2 nanosheet surface. As the distance between the FAM-labelled β-Lg aptamer and WS2 nanosheets increased, the fluorescence signal recovered due to loss of the FRET effect.
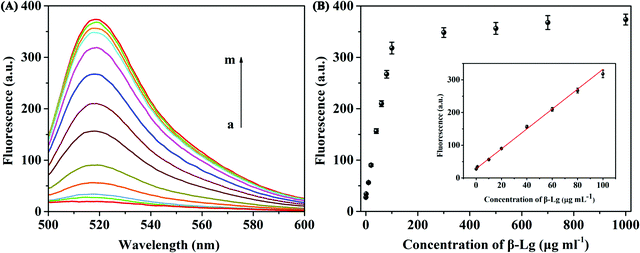 |
| Fig. 4 (A) FAM labelled β-Lg aptamer fluorescence emission spectra of the proposed method at different concentrations of β-Lg (0, 0.1, 1, 10, 20, 40, 60, 80, 100, 300, 500, 700, and 1000 μg mL−1). (B) Diagram of peak fluorescence and target β-Lg concentration. Inset: Linear fitting of fluorescence intensity at 518 nm with the target β-Lg concentration. | |
The fluorescence intensity in the system and the concentration of β-Lg showed a good linear relationship within the range of 0.1–100 μg mL−1 (Fig. 4B). The fitting equation of linear regression was F = 2.934C + 30.82 (R2 = 0.9911), and the limit of detection (LOD) was 20.4 ng mL−1. Compared with other β-Lg detection methods, this method showed similar or better analytical performance (Table S1, ESI†). In order to evaluate the selectivity of the β-Lg detection method, we selected the same concentration of other types of proteins, i.e., α-La, ovalbumin, BSA, casein and γ-globulin, as the interfering substances. As displayed in Fig. 5A, these proteins did not change the fluorescence intensity compared with β-Lg. Meanwhile, the presence of coexisting proteins had no obvious interference on the β-Lg detection (Fig. 5B). The difference of fluorescence enhancement between the β-Lg and other proteins was statistically significant (p < 0.001) (Fig. S2, ESI†). These experimental results proved that this method has good selectivity for the β-Lg detection.
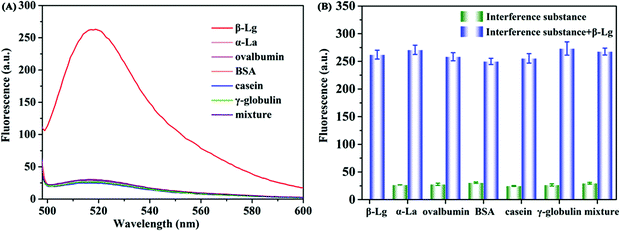 |
| Fig. 5 Selectivity of the method. (A) Fluorescence emission spectra and (B) the fluorescence intensity at 518 nm of the system upon the addition of β-Lg, α-La, ovalbumin, BSA, casein, γ-globulin and mixture proteins. | |
β-Lg detection in food samples
Finally, different milk and infant formula samples were selected to study the applicability of our method in actual sample detection. In the case of the same β-Lg calibration curve, the content of β-Lg in the samples was measured by the as-developed fluorescence method. The fluorescence intensity in the system increased with the increase of the concentration of β-Lg. As shown in Table S2 (ESI†), the detected concentration of β-Lg by this method was consistent with that from commercial ELISA, and the relative standard deviation (RSD) was between 2.6% and 4.9%. The recovery rates of β-Lg in the spiked infant formula samples ranged from 98.1% to 103.5% (Table S3, ESI†). These experimental results showed that this method can be used to detect β-Lg in real samples with good precision and stability.
Conclusions
In conclusion, we developed a simple and sensitive fluorescence method for detecting β-Lg based on a β-Lg aptamer and WS2 nanosheets. The β-Lg aptamer was easily adsorbed on WS2 nanosheets and triggered the fluorescence to be quickly quenched by the WS2 nanosheets. When the target β-Lg was present, the fluorescence could be recovered. The detection limit was 20.4 ng mL−1, and the specificity of the detection method for β-Lg was superior to other interfering proteins. This fluorescence method was further applied to detect the content of β-Lg in actual samples, with good accuracy. Therefore, the as-developed approach shows practical application value in allergen β-Lg detection.
Conflicts of interest
There are no conflicts to declare.
Acknowledgements
This work was supported by the National Natural Science Foundation of China (21804050 and 22004032), the Natural Science Foundation of Fujian Province of China (2019J05098), and the Hunan Provincial Natural Science Foundation of China (2021JJ20020).
Notes and references
- S. H. Sicherer and H. A. Sampson, Food Allergy, 2010, 125, S116–S125 Search PubMed.
- S. McClain, C. Bowman, M. Fernández-Rivas, G. S. Ladics and R. van Ree, Clin. Transl. Allergy, 2014, 4, 1–9 CrossRef PubMed.
- C. Villa, J. Costa, M. B. P. P. Oliveira and I. Mafra, Compr. Rev. Food Sci. Food Saf., 2018, 17, 137–164 CrossRef PubMed.
- J. Y. Yin, J. S. Huo, X. X. Ma, J. Sun and J. Huang, Biomed. Environ. Sci., 2017, 30, 875–886 CAS.
- B. Rullier, M. A. V. Axelos, D. Langevin and B. Novales, J. Colloid Interface Sci., 2010, 343, 330–337 CrossRef CAS PubMed.
- T. Nicolai, M. Britten and C. Schmitt, Food Hydrocolloids, 2011, 25, 1945–1962 CrossRef CAS.
- J. M. Skripak, E. C. Matsui, K. Mudd and R. A. Wood, J. Allergy Clin. Immunol., 2017, 120, 1172–1177 CrossRef PubMed.
- M. Nehra, M. Lettieri, N. Dilbaghi, S. Kumar and G. Marrazza, Sensors, 2020, 20, 32 CrossRef CAS PubMed.
- K. J. Allen, P. J. Turner, R. Pawankar, S. Taylor, S. Sicherer, G. Lack, N. Rosario, M. Ebisawa, G. Wong, E. N. C. Mills, K. Beyer, A. Fiocchi and H. A. Sampson, World Allergy Organ. J., 2014, 7, 1–14 CrossRef PubMed.
- O. Rotkāja, J. Goluško and P. Mekšs, Mater. Sci. Appl. Chem., 2016, 33, 36–39 Search PubMed.
- L. I. Boitz, G. Fiechter, R. K. Seifried and H. K. Mayer, J. Chromatogr. A, 2015, 1386, 98–102 CrossRef CAS PubMed.
- J. Ji, P. Zhu, F. Pi, C. Sun, J. Sun, M. Jia, C. Ying, Y. Zhang and X. Sun, Food Control, 2017, 74, 79–88 CrossRef CAS.
- S. He, X. Li, J. Gao, P. Tong and H. Chen, Food Chem., 2017, 227, 33–40 CrossRef CAS PubMed.
- C. Li, Q. Zhu, H. Chang, M. Jiang, S. Mao, Z. Chen, L. Kong, H. Liu, H. Tian and J. Wang, J. Electroanal. Chem., 2021, 115964 Search PubMed.
- P. Galan-Malo, S. Pellicer, M. D. Pérez, L. Sánchez, P. Razquin and L. Mata, Food Chem., 2019, 293, 41–48 CrossRef CAS PubMed.
- C. Villa, J. Costa, M. B. P. P. Oliveir and I. Mafra, Food Control, 2010, 108, 106823 CrossRef.
- M. Horká, J. Šalplachta, P. Karásek and M. Roth, Food Chem., 2022, 131986 CrossRef PubMed.
- L. Hong, M. Pan, X. Yang, X. Xie, K. Liu, J. Yang, S. Wang and S. Wang, J. Nanobiotechnol., 2022, 20, 1–12 CrossRef PubMed.
- X. Fang and W. Tan, Acc. Chem. Res., 2010, 43, 48–57 CrossRef CAS PubMed.
- S. Song, L. Wang, J. Li, C. Fan and J. Zhao, TrAC, Trends Anal. Chem., 2008, 27, 108–117 CrossRef CAS.
- C. Zhang and L. W. Johnson, Anal. Chem., 2019, 81, 3051–3055 CrossRef PubMed.
- K. Mao, H. Zhang, Z. Wang, H. Cao, K. Zhang, X. Li and Z. Yang, Biosens. Bioelectron., 2020, 148, 111785 CrossRef CAS PubMed.
- Z. Chen, M. Sun, F. Luo, K. Xu, Z. Lin and L. Zhang, Talanta, 2018, 178, 563–568 CrossRef CAS PubMed.
- S. Li, X. Liu, S. Liu, M. Guo, C. Liu and M. Pei, Anal. Chim. Acta, 2021, 1141, 21–27 CrossRef CAS PubMed.
- S. Qi, N. Duan, Y. Sun, Y. Zhou, P. Ma, S. Wu and Z. Wang, Sens. Actuators, B, 2021, 340, 129956 CrossRef CAS.
- M. Shi, Y. Cen, M. Sohail, G. Xu, F. Wei, Y. Ma, X. Xu, Y. Ma, Y. Song and Q. Hu, Microchim. Acta, 2018, 185, 1–8 CrossRef CAS PubMed.
- C. H. Lu, H. H. Yang, C. L. Zhu, X. Chen and G. N. Chen, Angew. Chem., 2009, 121, 4879–4881 CrossRef.
- D. Chimene, D. L. Alge and A. K. Gaharwar, Adv. Mater., 2015, 27, 7261–7284 CrossRef CAS PubMed.
- G. Chen, Y. Li, M. Bick and J. Chen, Chem. Rev., 2020, 120, 3668–3720 CrossRef CAS PubMed.
- N. Zhang, F. Huang, S. Zhao, X. Lv, Y. Zhou, S. Xiang, S. Xu, Y. Li, G. Chen, C. Tao, Y. Nie, J. Chen and X. Fan, Matter, 2020, 2, 1260–1269 CrossRef.
- J. Chen, Y. Huang, N. Zhang, H. Zhou, R. Liu, C. Tao, X. Fan and Z. L. Wang, Nat. Energy, 2016, 1, 1–8 CAS.
- X. Xiao, X. Xiao, Y. Zhou, X. Zhao, G. Chen, Z. Liu, Z. Wang, C. Lu, M. Hu, A. Nashalian, S. Shen, K. Xie, W. Wang, Y. Gong, W. Ding, P. Servati, C. Han, S. X. Dou, W. Li and J. Chen, Sci. Adv., 2021, 7, eabl3742 CrossRef CAS PubMed.
- Q. Xi, D. M. Zhou, Y. Y. Kan, J. Ge, Z. K. Wu, R. Q. Yu and J. H. Jiang, Anal. Chem., 2014, 86, 1361–1365 CrossRef CAS PubMed.
- X. Zuo, H. Zhang, Q. Zhu, W. Wang, J. Feng and X. Chen, Biosens. Bioelectron., 2016, 85, 464–470 CrossRef CAS PubMed.
- I. M. Khan, S. Niazi, Y. Yu, A. Mohsin, B. S. Mushtaq, M. W. Iqbal, A. Rehman, W. Akhtar and Z. Wang, Anal. Chem., 2019, 91, 14085–14092 CrossRef CAS PubMed.
- P. Wang, A. Wang, M. M. Hassan, Q. Ouyang, H. Li and Q. Chen, Sens. Actuators, B, 2020, 320, 128434 CrossRef CAS.
- S. Eissa and M. Zourob, Biosens. Bioelectron., 2017, 91, 169–174 CrossRef CAS PubMed.
- M. Lettieri, O. Hosu, A. Adumitrachioaie, C. Cristea and G. Marrazza, Electroanalysis, 2020, 32, 217–225 CrossRef CAS.
- Q. Qiu, X. Ni, T. Liu, Z. Li, X. An and X. Chen, Analyst, 2021, 146, 6808–6814 RSC.
|
This journal is © The Royal Society of Chemistry 2022 |