DOI:
10.1039/D0RA04651E
(Review Article)
RSC Adv., 2020,
10, 26742-26751
Tumor microenvironment triggered biodegradation of inorganic nanoparticles for enhanced tumor theranostics
Received
26th May 2020
, Accepted 10th July 2020
First published on 16th July 2020
Abstract
Inorganic nanoparticles (NPs)-mediated tumor theranostics have attracted widespread attention due to their unique physicochemical properties, such as optical, electrical, magnetic, and thermal properties. In the past decade, great advancements have been made in inorganic NPs-associated drug delivery, multimodal tumor imaging, and tumor therapy. However, the potential toxicity of inorganic NPs due to their low biodegradability, background signals interference and treatment side effects limit their clinical application. Therefore, developing biodegradable and intelligent NPs is beneficial to avoid excessive metal ions deposition, specific tumor imaging and treatment. In this review, we summarize the recent advances in tumor microenvironment (TME)-triggered biodegradation of inorganic NPs accompanied by imaging signal amplification and the released ions-mediated tumor therapy. First, the feature characteristics of the TME are introduced, including mild acidity, hypoxia, overexpressed reactive oxygen species (ROS), glutathione (GSH), and enzymes et al.; then, the biodegradation of NPs in a TME-induced activation of imaging signals, such as magnetic resonance (MR) imaging and fluorescence imaging is described; furthermore, tumor therapies through “Fenton”, “Fenton-like” reactions, and interference of biological effects in cells is presented. Finally, the challenges and outlook for improving the degradation efficiency, imaging, specificity and efficiency of tumor imaging and treatment are discussed.
1. Introduction
Malignant tumors are one of the most severe threats to human health, and the incidence and mortality remain very high worldwide.1,2 In recent decades, the advent of nanotechnology has provided new opportunities for tumor theranostics due to the unique nanoeffect of nanoparticles (NPs). Among of which, inorganic NPs have been the subject of great attention based on their good stability and excellent physicochemical properties in many areas, including light, heat, sound, electricity, and magnetism et al. Compared with organic NPs, inorganic NPs exhibit more stable properties and can be reused multiple times. Moreover, these properties can be converted each other, which enrich the use of inorganic NPs.
To date, a series of inorganic NPs have been well known to be applied for tumor imaging and therapeutic research. For instance, metal nanoclusters,3 quantum dots (QDs),4 upconversion NPs (UCNPs),5 and persistent luminescence NPs6,7 have been proven to have significant antiphotobleaching properties in terms of cell fluorescent labeling and in vivo tumor fluorescence imaging. Gadolinium oxide and iron oxide are recognized as classic contrast agents (CAs) for magnetic resonance (MR) imaging;8,9 Nanoparticles containing heavy metal elements (Au, Bi, Pt, and Hf) were usually used as computerized tomography (CT) imaging CAs depending on the strong attenuation of X-rays.10,11 Metal sulfides, such as CuS and Bi2S3, can effectively and repeatedly convert the absorbed near-infrared (NIR) light into heat, which can be used for photothermal treatment and photoacoustic (PA) imaging of tumors;12,13 Inorganic photosensitizers or sonosensitizers, such as TiO2, Au25(Capt)18 nanoclusters, and PtCu3 NPs can inhibit tumor growth by producing reactive oxygen species (ROS) under laser or ultrasonic excitation;14–16 in particular, inorganic NPs with multimodal imaging and therapeutic functions have been continuously developed for precise tumor theranostics. For instance, our group synthesized Gd:CuS@BSA NPs by using an albumin-mediated biomimetic strategy. The obtained Gd:CuS@BSA NPs exhibited significant absorbance in NIR with high photothermal conversion efficacy (32.3%) and a significant longitudinal relaxation rate (r1 = 16.032 mM−1 s−1) than the clinical MR contrast agent (Magnevist, r1 = 3.843 mM−1 s−1). Thus, they were applied for in vivo tumor MR/PA imaging-guided photothermal therapy.17 Overall, inorganic NPs demonstrate their versatility and excellent properties in tumor diagnosis and treatment. However, there are still some problems that need to be resolved: (1) compared with organic NPs, in vivo degradability and toxicity of inorganic nanoparticles have been a concern for a long time. After intravenous injection, inorganic NPs accumulate in tumors as well as normal tissues, and long-term deposition often produces toxicity, which limits its clinical application.18 Therefore, degradability will become the primary consideration when synthesizing inorganic NPs in the future; (2) currently, most obtained inorganic NPs employ an “always-on” mode for tumor theranostics, which brings about some advantages. For tumor imaging, the “always-on” signal can produce strong background interferences in vivo and significantly reduce the signal-to-noise ratio (SNR) in the tumor site, hindering diagnostic efficiency.19,20 For tumor therapy, if laser or ultrasonic treatments accidentally irradiate normal tissues, the inorganic NPs can also produce ROS or heat and cause notable side effects.
Considering the significantly different characteristics between the TME and normal tissues, such as mild acidity, hypoxia, overexpressed ROS, and glutathione (GSH) et al., developing TME-responsive inorganic NPs with intrinsic imaging signal and therapeutic function activation can significantly reduce background signal interference (high SNR) and normal tissue toxicity, achieving tumor specific theranostics. Therefore, biodegradation and a “turn-on” theranostic strategy are essential for the future development and clinical transformation of inorganic NPs.
In this review, we summarize the recent advances in the TME-triggered biodegradation of inorganic NPs accompanied by imaging signal amplification and released ions-mediated tumor therapy (Scheme 1). First, the feature characteristics of the TME are introduced, including mild acidity, hypoxia, overexpressed ROS, and glutathione (GSH). Then, the induced biodegradation of NPs in the TME to enhance imaging signals, such as MR imaging and fluorescence imaging is described. Furthermore, tumor therapies, through the generation of toxic ROS by the released ions from mediated “Fenton” and “Fenton-like” reactions and the interference of biological effects in cells, are reviewed. Finally, the challenges and outlook for improving the degradation efficiency, imaging, specificity and efficiency of tumor imaging and treatment are discussed.
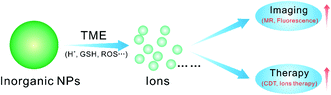 |
| Scheme 1 Schematic illustration showing the tumor microenvironment (H+, GSH, ROS, etc.) triggering the biodegradation of inorganic NPs for enhanced tumor imaging (MR imaging, fluorescence imaging) and therapy (chemodynamic therapy, ions therapy). | |
2. Feature characteristics of the TME
The tumor microenvironment (TME), which is created by interactions between nontransformed and malignant cells, is a complex physical and biochemical system that plays a crucial role in tumor occurrence, growth, and metastasis.21,22 Compared with normal tissues, there are many significant differences in the TME, which are listed as follows:
(1) Mild acidity: mild acidity is regarded as one of the most archetypal characteristics of the TME. According to previous inferences, this mild acidity is the result of the accumulation of lactate (pKa = 3.86), the metabolite of excessive aerobic glycolysis (Warburg effect).23 In 2008, Gallagher et al.24 exploited the dynamic nuclear polarization (DNP) technique to realize the in vivo MR imaging of tissue pH based on the ratio of hyperpolarized bicarbonate (H13CO3−) and 13CO2 signal intensities after the intravenous injection of hyperpolarized H13CO3−. After MR imaging of an EL4 tumor pH in vivo, they found that the pH value of tumor interstitials was lower than that of the surrounding tissues. According to previous research, the pH value range of the tumor extracellular and intracellular environment is within 6.0–7.0 and 6.0–6.5, respectively.24
(2) Overexpressed glutathione (GSH): glutathione (GSH) is the most abundant intracellular cysteine-containing small molecule biothiol. Its role is to maintain the balance of the redox state in the cell and is a vital endogenous antioxidant. The ratio of reduced glutathione (GSH) to its oxidized form (GSSH) is an important indicator of the redox state of the cell and determines the antioxidant capacity of the cell. It has been reported that the GSH level in tumor cells is ∼10 mM, which is significantly higher than that in normal cells (∼2 μM).25 Thus, GSH can be used as an endogenous tumor stimulator to achieve reduction-responsive drug release.26
(3) Hypoxia: tumor hypoxia is caused by the rapid proliferation of tumor cells, which consumes a large amount of oxygen and decreases oxygen delivery efficiency due to the abnormal structure and function of tumor tissue.27,28 In some solid tumors, the partial pressure of oxygen is close to 0 mmHg, while the partial pressure of oxygen in normal tissue is 30 mmHg. Furthermore, tumor cells that are farther away from the blood vessel have a lower partial pressure of oxygen, making it difficult to deliver drugs into the tumor tissue. As a result, the treatment efficiency of radiotherapy and chemotherapy on hypoxic cells is weakened.29 Thus, some conjugated polymers have been designed and synthesized to encapsulate drugs, followed by their release under hypoxic conditions.
(4) H2O2: in a previous study, it has been found that tumor cells can produce higher concentrations of H2O2 than normal cells.30,31 H2O2 can induce DNA damage and mutations as well as increase genetic instability.32 At the same time, an increase in H2O2 concentration can also activate HIF-1, which plays a role in apoptosis resistance, invasion/metastasis, angiogenesis and immortality.33 By leveraging the overexpressed H2O2 in tumors, some inorganic NPs can be designed to catalyze H2O2 to O2, thus relieving tumor hypoxia. In addition, H2O2 can trigger the hydrophilic–hydrophobic transition of some polymers, causing the drug release in tumors while avoiding side effects in normal tissues.34,35
3. TME-triggered biodegradation of NPs for enhanced tumor imaging
3.1. TME-activated MR imaging
As a powerful imaging technique, MR imaging has been widely used in tumor detection due to a series of advantages, such as its high spatial resolution, lack of ionizing radiation, no penetration depth limit, and excellent soft tissue contrast et al.36,37 Currently, most nanoscale MR contrast agents (CAs) have a high relaxivity and exhibit a good contrast effect, improving the accuracy of tumor detection. However, most of these CAs are difficult to degrade, resulting in long-term toxicity. To resolve this problem, during the past few years, biodegradable nanoscale CAs have been developed to amplify by MR signals in tumors.
3.1.1 Mn-based MR CAs. Manganese(II), which has five unpaired electrons and high r1 relaxivity, is a typical paramagnetic ion that has been widely used for MR imaging.38 In comparison, Mn4+ ions exhibited a lower r1 relaxivity due to fewer unpaired electrons, thus manganese dioxide (MnO2) NPs show an insignificant MR contrast effect. Nonetheless, MnO2 NPs has still attracted research interest in the past decades due to their biodegradability in the TME, yielding Mn2+ and improving r1 relaxivity. For instance, Chen et al.39 found that the r1 relaxivity of synthesized 2D MnO2 nanosheets was as low as 0.007 mM−1 s−1. However, after an acidic buffer solution treatment (pH = 6.0 and 4.6), the r1 relaxivity exhibited a significant increase from 0.007 mM−1 s−1 to 3.4 mM−1 s−1 and 4.0 mM−1 s−1, respectively, due to the emergence of Mn2+. Then, the 2D-MnO2 nanosheets were employed as ultrasensitive pH-responsive MR CAs for in vivo imaging. In addition to MnO2 NPs, other Mn-doped inorganic NPs have also been developed to explore TME-activated MR imaging. For instance, Mi et al.40 doped Mn2+ into pH-sensitive calcium phosphate (CaP) NPs comprising a poly(ethylene glycol) shell. After intravenous injection and accumulation in the tumor, the low pH of the TME could trigger the disintegration of CaP, resulting in the release of Mn2+ ions. Thus, the significant tumor-to-normal (T/N) tissue contrast ratio was significantly increased by approximately 170% for several hours, which is higher than that of clinically used MR CAs (Gd-DTPA, T/N = 120%).In addition, other Mn-doped inorganic NPs, such as Mn-doped layered double hydroxide (LDHs)41 and Mn-doped biodegradable SiO2 (ref. 42) have been reported as pH- or redox-sensitive MR probes for in vivo tumor imaging. Very recently, Nie et al.43 synthesized ultrasmall manganese ferrite NPs, followed by modification with a tumor-targeting CREKA (Cys–Arg–Glu–Lys–Ala) peptide to form UMFNP–CREKA NPs. In the presence of mild acidity and H2O2, Mn2+ was released from UMFNP–CREKA NPs, resulting in a significant increase in the T1 signal. Moreover, the accumulated Mn2+ could bind with proteins in the cancerous tissues, which further improved the T1 MR signal and brightened the metastatic tumor (Fig. 1A). As displayed in Fig. 1B, the T1-weighted MR signal of the tumor was significantly enhanced 50 min after the injection of UMFNP–CREKA NPs, while the signals of the other two control groups exhibited a negligible increase. These results demonstrated that the in vivo T1 MR signal was activated by the Mn2+ release due to the TME (mild acidity and H2O2).
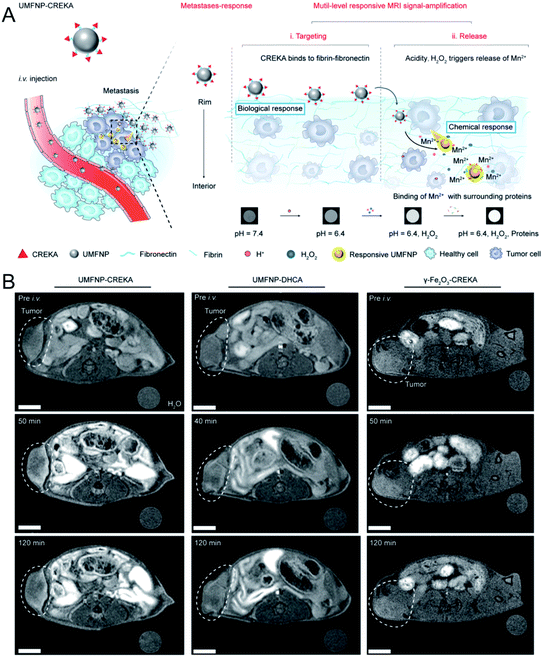 |
| Fig. 1 Schematic illustration showing UMFNP–CREKA NPs as a TME (mild acidity and H2O2)-activated T1-weighted MR imaging probe; (B) in vivo time-dependent MR images before and after the intravenous injection of UMFNP–CREKA NPs, UMFNP–DHCA NPs, and γ-Fe2O3–CREKA NPs, from left to right, respectively.43 Copyright from Wiley, 2020. | |
3.1.2 Fe-based MR CAs. Similar to Mn(II), the outer unpaired electrons of Fe(III) make it another magnetic element candidate for designing MR CAs. For example, Fe-based inorganic NPs, such as magnetic iron oxide (>5 nm), are well known T2-weighted MR CAs, and Fe3+-based metal organic complexes exhibit a remarkable T1 contrast enhancement effect.44 However, T2-weighted MR CAs usually produce dark signals in lesions that are easily disturbed by other hypointense regions such as calcification, air, blood clots, and hemorrhage, while bright signals produced by T1-weighted MR CAs often interfere with the background signal from normal tissues. Thus, developing tumor-specific T1-weighted MR imaging probes is of great importance in improving the significant T/N tissue contrast ratio.In a previous study, Yu et al.46 focused on the synthesis and tumor theranostics of iron carbide NPs (Fe5C2). They measured the transverse relaxivity (r2) of Fe5C2 NPs to be 312 mM−1 s−1, indicating a remarkable T2 contrast enhancement effect. To realize tumor-activated MR imaging, in recent work, they fabricated Fe5C2@Fe3O4 NPs with a core–shell structure, which were highly magnetic and demonstrated T2-weighted MR imaging effects in normal tissue. However, Fe5C2@Fe3O4 NPs show pH sensitivity in acidic environments, accompanied by the release of paramagnetic ferric ions (Fig. 2A). After dispersing the Fe5C2@Fe3O4 NPs in acidic phosphate-buffered saline (PBS) (pH = 5.4), the transverse relaxation rate (r2) was reduced from 203.83 mM−1 s−1 to 64.18 mM−1 s−1, while the longitudinal relaxation rate (r1) was increased from 0.26 mM−1 s−1 to 1.01 mM−1 s−1 (Fig. 2B). Thus, after 24 h of intravenous injection, the T1-weighted MR signal in the tumor was significantly enhanced from 99.1% to 123.7%, successfully achieving an acidic tumor environment-activated MR imaging enhancement (Fig. 2C).45
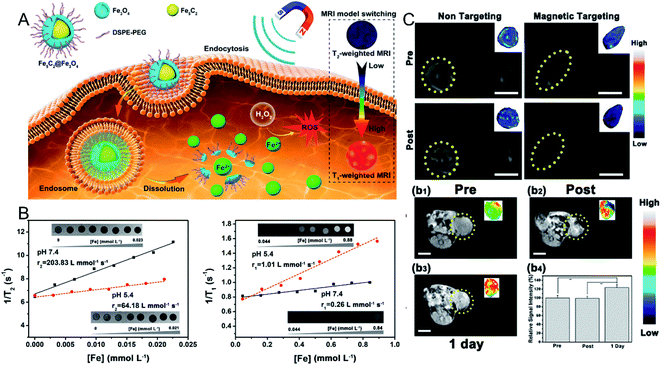 |
| Fig. 2 (A) Schematic illustration showing the Fe5C2@Fe3O4 NPs as a TME-activated T1-weighted MR imaging probe. (B) The in vitro MR images, transverse and longitudinal relaxation rates of Fe5C2@Fe3O4 NPs in different pH conditions (7.4 and 5.5); (C) in vivo T2-weighted MR images before and after the intravenous injection of Fe5C2@Fe3O4 NPs with and without magnetic targeting; in vivo T1-weighted MR images before and after the intratumoral injection of Fe5C2@Fe3O4 NPs, and the relative signal intensities.45 Copyright from American Chemical Society, 2019. | |
3.2. TME-activated fluorescence imaging
Compared with MR imaging, the most significant advantage of fluorescence imaging is its high sensitivity. To date, a series of materials with excellent fluorescence properties have been developed, including organic dyes, quantum dots (QDs), metal nanoclusters, and upconversion NPs (UCNPs) et al. However, the greatest obstacle to in vivo fluorescence imaging is autofluorescence interference from tissues. Thus, a “turn-on” strategy is usually designed to specifically activate the fluorescence signal at the tumor site and achieve low background fluorescence during imaging. For instance, manganese dioxide (MnO2)NPs, a classic biodegradable material in the TME, have been widely used as a surface modification of fluorescence probes. As a result, the fluorescence signal is quenched due to the fluorescent resonance energy transfer (FRET) effect that occurs between the MnO2 and fluorescent probes. Thus, after intravenous injection, no fluorescence signal can be detected in normal tissues and organs. However, after accumulation in the tumor, the MnO2 NPs modified on the surface of the probe can be decomposed by GSH, H2O2, and the acidic TME, resulting in the recovery of the fluorescence signal of the probe and realizing tumor-specific fluorescence imaging. For instance, Kong et al.47 synthesized silicon quantum dots (Si QDs), followed by conjugation with photosensitizer (Ce6) and MnO2 nanoparticles, respectively. As a result, the obtained BSA–Ce6–Si–MnO2 nanoparticles exhibited weak fluorescence due to FRET effect induced by MnO2. After entering into tumor cells, the fluorescence signal of Si QDs and Ce6 were recovered due to MnO2 degradation in the presence of acid condition and H2O2, realizing tumor specific fluorescence imaging. To further increase light penetration and reduce background signal interference, Liu et al.48 constructed UCNP–MnO2 FRET nanosystem using a similar strategy, which was applied for GSH detection.
In addition, Yang et al.49 reported another strategy to realize TME-activated fluorescence imaging by assembling a disulfide-bond-linked hydroxyethyl starch paclitaxel (HES–SS–PTX) conjugate and a near-infrared (NIR) cyanine fluorophore DiR. First, the fluorescence signal was quenched due to the aggregation-caused quenching (ACQ) effect. However, after the NPs were internalized by tumor cells, the disulfide bond (S–S) of HES–SS–PTX could be cleaved by intracellular GSH, resulting in the synchronized release of conjugated PTX and loaded DiR. The released DiR dyes restored the fluorescent properties of the NPs. Two hours after the injection, the fluorescence signal was detected at the tumor site, and the signal of nanoparticles was significantly higher than that of free DiR dyes.
4. TME-triggered biodegradation of nanoparticles for enhanced tumor therapy
4.1. Tumor chemodynamic therapy (CDT)
Traditional cancer treatment strategies such as surgical resection, chemotherapy and radiotherapy have obvious side effects. Emerging phototherapy strategies, such as photodynamic therapy and photothermal therapy, are often limited by the penetration depth of light. Therefore, developing an efficient and safe treatment strategy is particularly important.
The Fenton reaction, a classic inorganic chemical reaction, is a process in which Fe2+ ions catalyze the decomposition of hydrogen peroxide (H2O2) to produce hydroxyl radicals (·OH), which are widely used in wastewater treatment due to their effectiveness in removing refractory organic pollutants. In the past few years, because ·OH can induce protein denaturation, DNA breakage, phospholipid membrane damage, and mitochondrial destruction of tumor cells, the Fenton reaction has begun to be turned toward tumor therapy, which was named as tumor chemodynamic therapy.
4.1.1 Fe ions-mediated tumor chemodynamic therapy. Superparamagnetic iron oxide NPs (SPIONs) have been broadly explored in versatile biomedical applications, including magnetic hyperthermia, magnetic-targeted drug delivery, and MR imaging. In addition, the SPIONs-mediated Fenton reaction has been explored in tumor therapy applications. For instance, Huo et al.50 synthesized biodegradable dendritic silica NPs with large pore (∼40 nm), followed by loading ultrasmall Fe3O4 NPs (∼2 nm) and glucose oxidase (6.0 nm
×
5.2 nm
×
7.7 nm) (Fig. 3A). After the intravenous injection and accumulation of the above NPs in tumors, glucose oxidase could decrease the amount of glucose in tumor cells and provide sufficient H2O2 for the subsequent Fenton reaction that was mediated by Fe ions derived from the degradation of the Fe3O4 NPs in the mildly acidic TME. Both electron spin resonance (ESR) measurements (Fig. 3B) and 3,3′,5,5′-tetramethylbenzidine (TMB) absorption spectra (Fig. 3C) confirmed the generation of ·OH. As displayed in Fig. 3D–G, the produced ·OH significantly inhibited the growth of 4T1 tumors (Fig. 3D and E) and U87 glioblastoma tumors (Fig. 3F and G) without obvious side effects. In addition to SPIONs, other Fe-based NPs have been developed for the combination of CDT and other treatment strategies. For instance, Hu et al.51 designed a NO-associated CDT system by mixing Fe3+ and coordination polymers (1, 5-bis[(L-proline-1-yl)diazen-1-ium-1, 2-diol O2-yl]-2,4-dinitrobenzene BPDB, NO donor), followed by ion exchange, forming Fe(II)-BNCP NPs. Subsequently, it was found that Fe(II)-BNCP could both efficiently catalyze H2O2 to produce ·OH and generate NO in the presence of GSH. Thus, tumor growth of the group treated with Fe(II)-BNCP was significantly suppressed compared with other control groups. This work not only demonstrated the great promise of gas therapy-CDT-enhanced antitumor activity but also provided a novel strategy to design prodrug-based nanoplatform with high drug loading efficiency for precise tumor treatment. Chen et al.52 prepared CuFeS2 NPs by using a protein biomimetic strategy. The CuFeS2 NPs exhibited not only a significant ability to produce ·OH but also high photothermal conversion efficacy. Consequently, tumor growth was efficiently inhibited by the combined CDT-photothermal therapy. Thus, combination of CDT and other treatment strategies will be promising for cancer therapy.
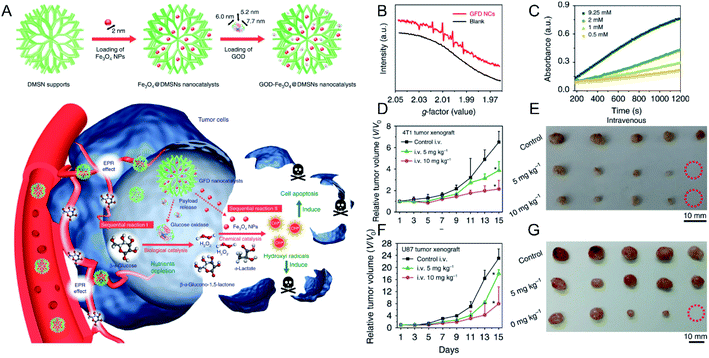 |
| Fig. 3 (A) Schematic illustration showing GOD–Fe3O4@DMSN for tumor selective catalytic therapy. (B) ESR spectra of GOD–Fe3O4@DMSN with and without the addition of glucose oxidase; (C) time-dependent absorbance of TMB with different concentrations of glucose oxidase; tumor growth curves and tumor images of (D and E) 4T1 and (F and G) U87 tumor-bearing mice.50 Copyright from Springer Nature, 2017. | |
4.1.2 Cu ions-mediated tumor chemodynamic therapy. Although the Fe2+-mediated Fention reaction has been developed as a safe strategy for cancer therapy, the reaction efficiency is high only in strongly acidic conditions (pH = 2–4).53 Thus, the production of ·OH is limited in weakly acidic tumor environments (pH = 5.5–6.5). In contrast, it has been reported that the reaction rate of Cu+-catalyzed Fenton-like reactions is as high as 1 × 104 M−1 s−1 under weakly acidic and neutral conditions, which is a ∼160-fold increase over that of Fe2+-catalyzed Fenton reactions.54,55 For instance, Li et al.56 synthesized copper–cysteine NPs (Cu–Cys NPs) that depended on the high coordination interaction between the sulfhydryl group (–SH) and Cu ions. After entering the cell by endocytosis, the Cu2+ in the Cu–Cys NPs could be reduced to Cu+ by GSH, followed by catalyzing H2O2 to trigger the Fenton-like reaction (Fig. 4A). As shown in Fig. 4B, the absorption peak of methylene blue (MB) exhibited a time-dependent downward trend, indicating the gradual generation of ·OH. More importantly, the reaction rate differs by only 10% under different pH conditions (pH = 5.5, 6.4, and 7.4), which ensured the high efficiency of the Cu–Cys NP-mediated CDT therapy of tumors. It can be seen from the tumor growth curve and tumor images (Fig. 4C) that the CDT efficiency was significantly higher than that of conventional chemotherapy (DOX-injection group). Thus, it can be seen the efficiency of Cu ions-mediated Fenton reaction is higher than Fe ions, which will be more effective for tumor therapy.
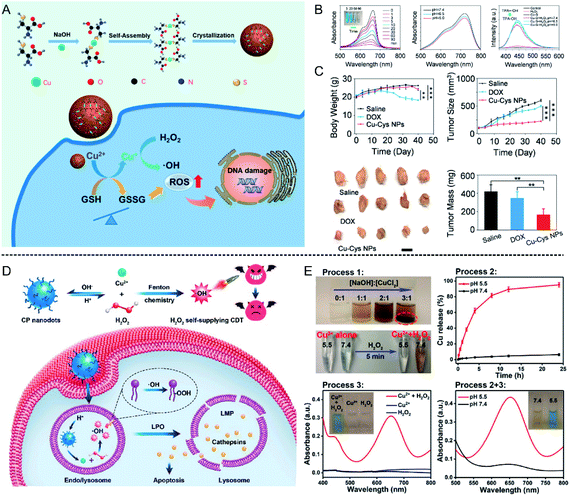 |
| Fig. 4 (A) Schematic illustration showing Cu–Cys NPs for tumor chemodynamic therapy; (B) characterization of hydroxyl radical (·OH) generation; (C) body weight, tumor size, tumor image, and tumor mass of different treatment groups (saline injection, DOX injection, and Cu–Cys NPs injection).56 Copyright from American Chemical Society, 2019. (D) Schematic illustration showing copper peroxide (CP) nanodots for self-supplying H2O2-enhanced chemodynamic therapy of tumors; (E) characterization of hydroxyl radical (·OH) generation in different pH conditions (5.5 and 7.4).57 Copyright from American Chemical Society, 2019. | |
Furthermore, the content of H2O2 is a crucial factor affecting the efficiency of the Fenton reaction. Therefore, to further increase the H2O2 concentration at the tumor site, Lin et al.57 synthesized copper peroxide (CP) nanodots by a coordination of Cu2+ and H2O2 in the presence of hydroxide ions (OH−), which could realize a self-supply of H2O2 at an acidic pH for enhanced CDT (Fig. 4D). Under acidic conditions, CP nanodots could release Cu2+ as well as H2O2, thus ·OH was produced, which was confirmed by using TMB as an indicator (Fig. 4E). The synthesized CP nanodots are the first example of a Fenton-type metal peroxide NPs, which provides new guidance for the development of other peroxides to enhance the CDT of tumors. In addition, Cu ion-mediated CDT can also be combined with other treatment strategies to enhance tumor therapy. Copper sulfide (CuS) is a common photothermal agent, and the application of CuS for tumor photothermal therapy has been systematically studied. Wu et al.58 found that the fabricated ultrasmall Cu2−x S-PEG nanodots could catalyze H2O2 into ·OH, and this reaction could be enhanced under laser irradiation at 1064 nm due to the heat generated by the Cu2−x S-PEG photothermal agent. Consequently, the combination of photothermal therapy (PTT) and CDT significantly suppressed tumor growth 2 days after treatment, and the tumors disappeared after 14 days, indicating good combined treatment efficiency.
4.1.3 Other ions-mediated tumor chemodynamic therapy. In addition to Fe and Cu ions, other metal ions, such as Co, Mn, and Ti ion-mediated Fenton-like reactions for CDT of tumors have been reported in recent years. Liang et al.59 synthesized CaO2 NPs, followed by loading DOX and adding Co2+ and 2-methylimidazole to forme CaO2@DOX@ZIF-67 NPs. In this work, ZiF-67 could be degraded under acidic conditions (pH = 5.0), leading to the release of Co2+ and triggering the Fenton-like reaction. More importantly, H2O2 could be efficiently self-supplied through the reaction between CaO2 and H2O to enhance the production of ·OH. Moreover, DOX could also be released in the TME, achieving a combined chemo/chemodynamic treatment of tumors. In addition to increasing the H2O2 concentration, reducing the GSH level is another strategy to ensure the generation of ·OH rather than being removed by overexpressed GSH in tumor cells. Lin et al.60 first reported a MnO2 CDT agent that could deplete GSH through a reaction and degrade into Mn2+. Subsequently, they found that H2O2 could be catalyzed by Mn2+ into ·OH in the presence of HCO3−1. The activated MR signals guided the CDT of tumor. TiO2 is a well-known sonosensitizer for sonodynamic therapy (SDT) of tumors. Very recently, Cheng et al.61 reported that Ti3+ is another alternative to iron ions for Fenton-like reactions. First, they synthesized ultrathin titanium monoxide (TiO1+x) nanorods and found that the ROS productivity under ultrasonic excitation was significantly higher than that of common TiO2 NPs. More importantly, the Ti ion-mediated Fenton-like reaction could be dramatically enhanced by ultrasonic excitation, achieving a combination of SDT and ultrasound-enhanced CDT of tumors. Thus, in our opinion, other metal elements with variable valence can be considered to be used for tumor therapy through Fenton reaction.
4.2. Ions interference therapy (IIT)
Unlike the above metal ions that generate ROS through the Fenton reaction to kill tumor cells (Table 1), ion interference therapy is a new tumor treatment strategy that induces cell apoptosis by blocking the biological effects and functions of cells, which was first reported in last year. Inspired by the emergence of microcalcifications in tumors after radiotherapy or chemotherapy (Fig. 5A), Zhang et al.63 synthesized ultrasmall CaO2 NPs (∼5 nm, Fig. 5B), followed by coating a sodium hyaluronate layer on their surface to obtain SH-CaO2 NPs. In the tumor lesion area, the protective layer was decomposed by overexpressed hyaluronidase, thus the exposed CaO2 NPs were accelerated to decompose in the acidic TME, producing H2O2 and free Ca2+. Due to the downregulation of catalase expression in tumor cells, H2O2 accumulated in the tumor cells and induced oxidative stress, which further led to the dysfunction of Ca-related ion channels. Thus, the Ca2+ concentration in tumor cells was unable to be regulated, resulting in the long-term retention of Ca. Over time, the abnormal Ca2+ aggregation triggered persistent calcium overload, hindered the metabolism of tumor cells, and induced metabolic disorders and functional dysfunction of tumor cells, thereby inducing cell death. At the same time, the local increase in Ca2+ concentration could also promote the development of microcalcifications in tumor lesions. This type of tumor cell death, which is induced by ultrasmall calcium peroxide NPs, is defined as “calcium death”. As displayed in CT images of tumors before and after injection (Fig. 5C), the CT signal is slightly enhanced 3 h after injection, but becomes significantly brighter after 3 days, indicating the existence of tumor calcification. Correspondingly, tumor growth is inhibited 14 days after treatment.
Table 1 Comparison between chemodynamic therapy (CDT) and ions interference therapy (IIT)
|
Ions |
Conditions |
Mechanism of tumor therapy |
Advantages |
Disadvantages |
Ref. |
CDT |
Fe2+ |
H2O2, H+ |
Ions catalyze the decomposition of H2O2 to produce ·OH through fenton reaction, inducing protein denaturation, DNA breakage, and mitochondrial destruction of tumor cells |
Safe, specific tumor therapy without side effects |
The efficiency is limited by amount of H2O2 |
50–52 |
Cu+ |
H2O2, H+ |
15,56 and 57 |
Mn2+ |
H2O2, H+, HCO3− |
60 |
Co2+ |
H2O2, H+ |
59,62 |
IIT |
Ca2+ |
— |
Inducing cell apoptosis by blocking the biological effects and functions of tumor cells |
Safe, high efficiency, specific tumor therapy without side effects |
The efficiency is constrained by the self-regulatory function of the cell and requires combination with other strategies |
63,64 |
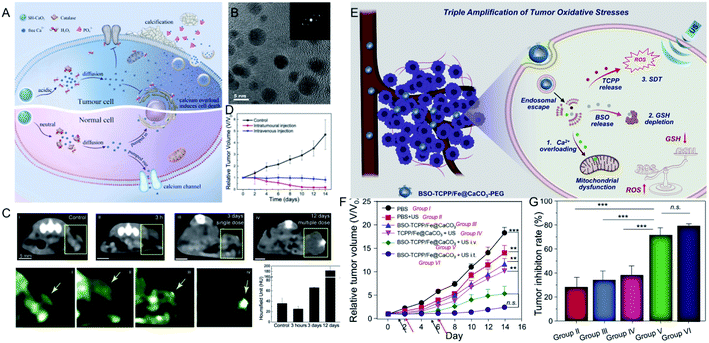 |
| Fig. 5 (A) Schematic illustration showing SH-CaO2 NPs for Ca2+ ion interference therapy of tumors; (B) TEM image of SH-CaO2 NPs; (C) in vivo time-dependent CT images of tumors before and after SH-CaO2 NPs injection; (D) tumor growth curve of the control group, the intratumoral injection of SH-CaO2 NPs, and the intravenous injection of SH-CaO2 NPs;63 copyright from Elsevier, 2019. (E) Schematic illustration showing BSO-TCPP/Fe@CaCO3-PEG NPs for the triple amplification of tumor oxidative stresses and tumor therapy; (F) tumor growth curve and tumor inhibition rate of different treatment groups.64 Copyright from Elsevier, 2020. | |
Furthermore, Ca2+-mediated ion interference therapy can be combined with other treatment strategies. Recently, Liu et al.64 synthesized hollow CaCO3 NPs, followed by loading an inhibitor for GSH biosynthesis (L-buthionine sulfoximine, BSO) and a sonosensitizer (meso-tetra-(4-carboxyphenyl)porphine, TCPP). As shown in Fig. 5E, the CaCO3 NPs were degraded under acidic conditions, and the released Ca2+ ions overloaded the tumor cells and induced mitochondrial damage. Moreover, the TCPP sonosensitizer was able to produce ROS under ultrasonic excitation, and the released BSO ensured that the ROS could not be removed by GSH. Eventually, the triple amplification of tumor oxidative stresses was applied toward tumor therapy. As displayed in Fig. 5F and G, the tumors of mice treated with BSO-TCPP/Fe3+@CaCO3 NPs and ultrasonication showed extremely slow growth compared with other groups, and the corresponding tumor inhibition rates were 70% (intravenous injection) and 80% (intratumoral injection), respectively. Besides Ca2+, other ions should be explored for their effect on the biological effects of tumor cells and then attempted for tumor therapy.
5. Summary and outlook
In conclusion, we have summarized recent achievements in the biodegradable inorganic NPs for enhanced tumor theranostics, including MR imaging, fluorescence imaging, chemodynamic therapy, and ion interference therapy of tumors. Regarding tumor imaging, the degradation of NPs and subsequent release of metal ions can activate signal, realizing low/no background tumor imaging; regarding tumor therapy, the released metal ions in the TME can produce ROS through the Fenton reaction or block the biological effects and functions of cells to inhibit tumor growth without side effects in normal tissues. Moreover, the degradation of NPs can accelerate their metabolism in the body, avoiding the toxicity from long-term accumulation. We believe that this review paper can guide researchers to find and design these degradable inorganic nanoparticles for tumor theranostics, which is very promising for clinical transformation.
Despite substantial progress made by many groups in designing biodegradable inorganic NPs for tumor imaging and therapy, it is fair to admit that there are still many problems that need to be addressed in the future: (1) the in vivo potential toxicity induced by released metal ions after degradation should be considered, as those metal ions can easily bind to proteins or enzymes. (2) The degradation of inorganic NPs is too monotonous and needs to be expanded. Currently, acidic conditions are predominant in decomposing inorganic NPs, but the degradation efficiency is not sufficient. Therefore, according to the characteristics of the TME (pH, GSH, hypoxia, H2O2, and enzymes), designing NPs that respond to multiple triggers is very effective in accelerating degradation. (3) After degradation, the efficacy of metal ion-mediated tumor treatment is still not efficient, especially in terms of the tumor inhibitory rate. Therefore, the earlier diagnosis and treatment of tumors combined with other strategies are particularly necessary. In essence, great effort should be devoted to resolving the in vivo degradation efficiency of inorganic NPs and enhancing tumor theranostics.
Conflicts of interest
There are no conflicts to declare.
Acknowledgements
This research was funded by National Nature Science Foundation of China (NSFC, 81801823), State Key Laboratory of Molecular Engineering of Polymers (Fudan University, K2020-16), and Shanghai Sailing Program (19YF1450700).
References
- C. Allemani, T. Matsuda, V. Di Carlo, R. Harewood, M. Matz, M. Nikšić, A. Bonaventure, M. Valkov, C. J. Johnson and J. Estève, Lancet, 2018, 391, 1023–1075 CrossRef.
- L. A. Torre, F. Bray, R. L. Siegel, J. Ferlay, J. Lortet-Tieulent and A. Jemal, Ca-Cancer J. Clin., 2015, 65, 87–108 CrossRef PubMed.
- A. Yahia-Ammar, D. Sierra, F. Mérola, N. Hildebrandt and X. Le Guével, ACS Nano, 2016, 10, 2591–2599 CrossRef CAS PubMed.
- M. N. Rhyner, A. M. Smith, X. Gao, H. Mao, L. Yang and S. Nie, Nanomedicine, 2006, 1, 209–217 CrossRef CAS PubMed.
- A. Gautam and P. Komal, Coord. Chem. Rev., 2018, 376, 393–404 CrossRef CAS.
- S.-K. Sun, H.-F. Wang and X.-P. Yan, Acc. Chem. Res., 2018, 51, 1131–1143 CrossRef CAS PubMed.
- T. Maldiney, A. Bessière, J. Seguin, E. Teston, S. K. Sharma, B. Viana, A. J. Bos, P. Dorenbos, M. Bessodes and D. Gourier, Nat. Mater., 2014, 13, 418–426 CrossRef CAS PubMed.
- M. Wu, Y. Xue, N. Li, H. Zhao, B. Lei, M. Wang, J. Wang, M. Luo, C. Zhang and Y. Du, Angew. Chem., Int. Ed., 2019, 58, 6880–6885 CrossRef CAS PubMed.
- P. Li, P. Chevallier, P. Ramrup, D. Biswas, D. Vuckovich, M.-A. Fortin and J. K. Oh, Chem. Mater., 2015, 27, 7100–7109 CrossRef CAS.
- F. Ratto, S. Centi, C. Avigo, C. Borri, F. Tatini, L. Cavigli, C. Kusmic, B. Lelli, S. Lai and S. Colagrande, Adv. Funct. Mater., 2016, 26, 7178–7185 CrossRef CAS.
- R. Meir, K. Shamalov, O. Betzer, M. Motiei, M. Horovitz-Fried, R. Yehuda, A. Popovtzer, R. Popovtzer and C. J. Cohen, ACS Nano, 2015, 9, 6363–6372 CrossRef CAS PubMed.
- K. Cherukula, K. Manickavasagam Lekshmi, S. Uthaman, K. Cho, C.-S. Cho and I.-K. Park, Nanomaterials, 2016, 6, 76 CrossRef PubMed.
- X. Yi, K. Yang, C. Liang, X. Zhong, P. Ning, G. Song, D. Wang, C. Ge, C. Chen and Z. Chai, Adv. Funct. Mater., 2015, 25, 4689–4699 CrossRef CAS.
- V. Deepagan, D. G. You, W. Um, H. Ko, S. Kwon, K. Y. Choi, G.-R. Yi, J. Y. Lee, D. S. Lee and K. Kim, Nano Lett., 2016, 16, 6257–6264 CrossRef CAS PubMed.
- X. Zhong, X. Wang, L. Cheng, Y. a. Tang, G. Zhan, F. Gong, R. Zhang, J. Hu, Z. Liu and X. Yang, Adv. Funct. Mater., 2019, 1907954 Search PubMed.
- P. Liu, W. Yang, L. Shi, H. Zhang, Y. Xu, P. Wang, G. Zhang, W. R. Chen, B. Zhang and X. Wang, J. Mater. Chem. B, 2019, 7, 6924–6933 RSC.
- W. Yang, W. Guo, W. Le, G. Lv, F. Zhang, L. Shi, X. Wang, J. Wang, S. Wang and J. Chang, ACS Nano, 2016, 10, 10245–10257 CrossRef CAS PubMed.
- E. B. Ehlerding, F. Chen and W. Cai, Adv. Sci., 2016, 3, 1500223 CrossRef PubMed.
- D. Reichel, M. Tripathi, P. Butte, R. Saouaf and J. M. Perez, Nanotheranostics, 2019, 3, 196 CrossRef PubMed.
- J. Zhang, L. Ning, J. Huang, C. Zhang and K. Pu, Chem. Sci., 2020, 11, 618–630 RSC.
- Z. Zhou and Z.-R. Lu, Adv. Drug Delivery Rev., 2017, 113, 24–48 CrossRef CAS PubMed.
- F. R. Balkwill, M. Capasso and T. Hagemann, J. Cell Sci., 2012, 125, 5591–5596 CrossRef CAS PubMed.
- H. Wu, Z. Ding, D. Hu, F. Sun, C. Dai, J. Xie and X. Hu, J. Pathol., 2012, 227, 189–199 CrossRef CAS PubMed.
- F. A. Gallagher, M. I. Kettunen, S. E. Day, D.-E. Hu, J. H. Ardenkjær-Larsen, P. R. Jensen, M. Karlsson, K. Golman, M. H. Lerche and K. M. Brindle, Nature, 2008, 453, 940–943 CrossRef CAS PubMed.
- Y. Li, K. Xiao, J. Luo, W. Xiao, J. Lee and K. S. Lam, Cancer Res., 2011, 71(8) DOI:10.1158/1538-7445.AM2011-4439.
- X.-D. Xu, Y.-J. Cheng, J. Wu, H. Cheng, S.-X. Cheng, R.-X. Zhuo and X.-Z. Zhang, Biomaterials, 2016, 76, 238–249 CrossRef CAS PubMed.
- J. Pouysségur, F. Dayan and N. M. Mazure, Nature, 2006, 441, 437–443 CrossRef PubMed.
- D. M. Gilkes, G. L. Semenza and D. Wirtz, Nat. Rev. Cancer, 2014, 14, 430–439 CrossRef CAS PubMed.
- M. R. Horsman, L. S. Mortensen, J. B. Petersen, M. Busk and J. Overgaard, Nat. Rev. Clin. Oncol., 2012, 9, 674 CrossRef CAS PubMed.
- T. P. Szatrowski and C. F. Nathan, Cancer Res., 1991, 51, 794–798 CAS.
- C. M. Doskey, V. Buranasudja, B. A. Wagner, J. G. Wilkes, J. Du, J. J. Cullen and G. R. Buettner, Redox Biol., 2016, 10, 274–284 CrossRef CAS PubMed.
- S. Park, X. You and J. A. Imlay, Proc. Natl. Acad. Sci. U. S. A., 2005, 102, 9317–9322 CrossRef CAS PubMed.
- G. L. Semenza, Nat. Rev. Cancer, 2003, 3, 721–732 CrossRef CAS PubMed.
- Y. Kuang, K. Baakrishnan, V. Gandhi and X. Peng, J. Am. Chem. Soc., 2011, 133, 19278–19281 CrossRef CAS PubMed.
- S. Park, J. Yoon, S. Bae, M. Park, C. Kang, Q. Ke, D. Lee and P. M. Kang, Biomaterials, 2014, 35, 5944–5953 CrossRef CAS PubMed.
- G. Angelovski, Angew. Chem., Int. Ed., 2016, 55, 7038–7046 CrossRef CAS PubMed.
- D. Ni, W. Bu, E. B. Ehlerding, W. Cai and J. Shi, Chem. Soc. Rev., 2017, 46, 7438–7468 RSC.
- B. Ding, P. Zheng, P. a. Ma and J. Lin, Adv. Mater., 2020, 32, 1905823 CrossRef CAS PubMed.
- Y. Chen, D. Ye, M. Wu, H. Chen, L. Zhang, J. Shi and L. Wang, Adv. Mater., 2014, 26, 7019–7026 CrossRef CAS.
- P. Mi, D. Kokuryo, H. Cabral, H. Wu, Y. Terada, T. Saga, I. Aoki, N. Nishiyama and K. Kataoka, Nat. Nanotechnol., 2016, 11, 724 CrossRef CAS.
- B. Li, Z. Gu, N. Kurniawan, W. Chen and Z. P. Xu, Adv. Mater., 2017, 29, 1700373 CrossRef PubMed.
- L. Yu, Y. Chen, M. Wu, X. Cai, H. Yao, L. Zhang, H. Chen and J. Shi, J. Am. Chem. Soc., 2016, 138, 9881–9894 CrossRef CAS PubMed.
- Y. Li, X. Zhao, X. Liu, K. Cheng, X. Han, Y. Zhang, H. Min, G. Liu, J. Xu, J. Shi, H. Qin, H. Fan, L. Ren and G. Nie, Adv. Mater., 2020, 32, 1906799 CrossRef CAS PubMed.
- F. Liu, X. He, H. Chen, J. Zhang, H. Zhang and Z. Wang, Nat. Commun., 2015, 6, 8003 CrossRef CAS PubMed.
- J. Yu, F. Zhao, W. Gao, X. Yang, Y. Ju, L. Zhao, W. Guo, J. Xie, X.-j. Liang and X. Tao, ACS Nano, 2019, 13, 10002–10014 CrossRef CAS PubMed.
- J. Yu, C. Yang, J. Li, Y. Ding, L. Zhang, M. Z. Yousaf, J. Lin, R. Pang, L. Wei and L. Xu, Adv. Mater., 2014, 26, 4114–4120 CrossRef CAS PubMed.
- R. Wang, M. Zhao, D. Deng, X. Ye, F. Zhang, H. Chen and J. Kong, J. Mater. Chem. B, 2018, 6, 4592–4601 RSC.
- R. Deng, X. Xie, M. Vendrell, Y.-T. Chang and X. Liu, J. Am. Chem. Soc., 2011, 133, 20168–20171 CrossRef CAS PubMed.
- Y. Li, Y. Wu, J. Chen, J. Wan, C. Xiao, J. Guan, X. Song, S. Li, M. Zhang, H. Cui, T. Li, X. Yang, Z. Li and X. Yang, Nano Lett., 2019, 19, 5806–5817 CrossRef CAS.
- M. Huo, L. Wang, Y. Chen and J. Shi, Nat. Commun., 2017, 8, 1–12 CrossRef CAS PubMed.
- Y. Hu, T. Lv, Y. Ma, J. Xu, Y. Zhang, Y. Hou, Z. Huang and Y. Ding, Nano Lett., 2019, 19, 2731–2738 CrossRef CAS PubMed.
- Q. Chen, Y. Luo, W. Du, Z. Liu, S. Zhang, J. Yang, H. Yao, T. Liu, M. Ma and H. Chen, ACS Appl. Mater. Interfaces, 2019, 11, 18133–18144 CrossRef CAS.
- H. Zhao, Y. Wang, Y. Wang, T. Cao and G. Zhao, Appl. Catal., B, 2012, 125, 120–127 CrossRef CAS.
- E. Brillas, M. A. Baños, S. Camps, C. Arias, P.-L. Cabot, J. A. Garrido and R. M. Rodríguez, New J. Chem., 2004, 28, 314–322 RSC.
- T. Soltani and B.-K. Lee, Chem. Eng. J., 2017, 313, 1258–1268 CrossRef CAS.
- B. Ma, S. Wang, F. Liu, S. Zhang, J. Duan, Z. Li, Y. Kong, Y. Sang, H. Liu and W. Bu, J. Am. Chem. Soc., 2018, 141, 849–857 CrossRef.
- L.-S. Lin, T. Huang, J. Song, X.-Y. Ou, Z. Wang, H. Deng, R. Tian, Y. Liu, J.-F. Wang and Y. Liu, J. Am. Chem. Soc., 2019, 141, 9937–9945 CrossRef CAS PubMed.
- R. Hu, Y. Fang, M. Huo, H. Yao, C. Wang, Y. Chen and R. Wu, Biomaterials, 2019, 206, 101–114 CrossRef CAS PubMed.
- S. Gao, Y. Jin, K. Ge, Z. Li, H. Liu, X. Dai, Y. Zhang, S. Chen, X. Liang and J. Zhang, Adv. Sci., 2019, 6, 1902137 CrossRef CAS PubMed.
- L. S. Lin, J. Song, L. Song, K. Ke, Y. Liu, Z. Zhou, Z. Shen, J. Li, Z. Yang and W. Tang, Angew. Chem., Int. Ed., 2018, 57, 4902–4906 CrossRef CAS PubMed.
- X. Wang, X. Zhong, L. Bai, J. Xu, F. Gong, Z. Dong, Z. Yang, Z. Zeng, Z. Liu and L. Cheng, J. Am. Chem. Soc., 2020, 142, 6527–6537 CrossRef CAS PubMed.
- Y. Sang, F. Cao, W. Li, L. Zhang and X. Qu, J. Am. Chem. Soc., 2020, 142, 5177–5183 CrossRef CAS PubMed.
- M. Zhang, R. Song, Y. Liu, Z. Yi, X. Meng, J. Zhang, Z. Tang, Z. Yao, Y. Liu and X. Liu, Chem, 2019, 5, 2171–2182 CAS.
- Z. Dong, L. Feng, Y. Hao, Q. Li, M. Chen, Z. Yang, H. Zhao and Z. Liu, Chem, 2020, 6, 1391–1407 CAS.
|
This journal is © The Royal Society of Chemistry 2020 |