DOI:
10.1039/C9RA08733H
(Paper)
RSC Adv., 2020,
10, 1439-1446
Aqueous-phase detection of antibiotics and nitroaromatic explosives by an alkali-resistant Zn-MOF directed by an ionic liquid†
Received
24th October 2019
, Accepted 15th December 2019
First published on 8th January 2020
Abstract
An alkali-resistant Zn-MOF directed by [BMI]Br ionic liquid, (BMI)2[Zn3(ptptc)2] (1), based on a π-electron-rich terphenyl-tetracarboxylic acid, has been synthesized under the combination of hydro/solvothermal and ionothermal condition (BMI = 1-butyl-3-methylimidazolium, H4ptptc = p-terphenyl-3,3′′,5,5′′-tetracarboxylic acid). In 1, the trinuclear Zn(II) clusters are linked by the organic moieties of the ptptc ligands, resulting in a 3D anionic framework structure with highly disordered [BMI]+ cations filled in the pores. 1 exhibits good chemical stability in water and NaOH solutions (pH range of 7–12), which allow it to detect antibiotics and nitroaromatic explosives in an aquatic system. 1 represents high fluorescence quenching efficiency toward NFs (furazolidone, FZD; nitrofurazone, NZF; nitrofurantoin, NFT), NMs (ronidazole, RDZ; metronidazole, MDZ; dimetridazole, DTZ; ornidazole, ODZ) and nitrophenol (2-nitrophenol, 2-NP; 3-nitrophenol, 3-NP; 4-nitrophenol, 4-NP; 2,4,6-trinitrophenol, TNP) in water solution, respectively.
Introduction
Antibiotics and nitroaromatic explosives, widely used in the clinic and in industry, have been noticed as undesirable organic pollutants in wastewater as well as drinking water, threatening the security of the environment and society.1,2 The abuse of antibiotics, particularly nitro-based antibiotics such as nitrofurans (NFs) and nitroimidazole (NMs), releases antibiotic residues in the environment, which are harmful to the health of humans and wildlife, due to their degradation products and toxicity.1 On the other hand, nitroaromatic explosives such as nitrophenols and 2-nitrotoluene (2-NT), 2,4-dinitrotoluene (2,4-DNT), are primary ingredients of industrial explosives and environmentally deleterious substances.2–4 Among all nitroaromatics, nitrophenols pose significant health risks on account of their physiologically active and potentially carcinogenic.3,4 2-nitrophenol (2-NP) and 4-nitrophenol (4-NP) have been listed as “Priority Pollutants” on the US Environmental Protection Agency's (USEPA's) list.4 Therefore, the efficient detection of antibiotics and explosives in water is a pressing challenge targeted at appropriate environmental monitoring.
Since the first work reported in 2009 by Li,5 luminescent metal–organic frameworks (LMOFs), based on the electron transfer and/or energy transfer mechanisms, have been demonstrated as promising alternatives to detect trace amounts of various nitroaromatics explosives.6–11 Also, Zhou and Li have recently reported two new chemically stable fluorescent Zr(IV)-based MOFs as sensing materials to detect antibiotics for the first time.12 LMOF sensor materials have advantages in terms of distinct luminescence spectrum, portability, short response times, high selectivity and sensitivity, applicability in both the solid and solution phase.5–15 However, due to the poor stability of LMOFs in water or in acidic/basic medium, most of them were checked in organic solvents instead of water.5–14 The kind of metal, organic ligand, guest as well as the topology of structure all play key roles in the physical or chemical properties of MOFs, such as water stability, ionic character, hydrophobicity, polarizability, pore apertures etc, which are crucial for achieving the targeted sensing application.10,11,16,17 Generally, implantation of π-conjugated organic group into the shaped ligand further extended to the framework, resulting in the smaller HOMO–LUMO energy gap, is an efficient way to enhance targeted property of MOFs and beneficial for sensing performance.10,11
Ionic liquids (ILs), as a type of ionic compounds, have received increasing attention as green solvent for the syntheses of MOFs due to their peculiar physical properties.18–23 In comparison with the traditional hydro/solvothermal procedure by using only water and/or organic solvents, the specific ionic environment of ILs would lead to the formation of novel MOFs that can not (or not easily) be obtained in molecular solvents. Relevant researches showed that the cation and the anion species can individually or cooperatively influence the resulting structures, due to the highly structured liquid with relatively long-range correlations. ILs could serve as a template directing agent even though neither the cation nor anion being occluded into the ultimate structure, facilitate the formation of condensed structure, obviously improve crystal quality and yield, and stimulate the structure conversion from a heterochiral coaxially nested double-helical column to a cationic spiral staircase.23 Our group have reported three types of lanthanide MOFs, all of which show excellent water-stability that probably can be attributed to the condensed structure directed by ILs, protecting these MOFs from the ingress of water.23b,23c,23e
We are particularly interested in using ILs to mediate novel MOFs with hydrolytic stability for water related applications.23b,23c,23e Herein, we report an alkali-resistant 3D porous Zn-MOF directed by [BMI]Br IL, (BMI)2[Zn3(ptptc)2] (1) (BMI = 1-butyl-3-methylimidazolium, H4ptptc = p-terphenyl-3,3′′,5,5′′-tetracarboxylic acid), for aqueous-phase detection of antibiotics and nitroaromatic explosives. H4ptptc has been extensively employed for the constructions of MOFs.24–26 As shown in Scheme 1, three types of Zn-MOFs based on H4ptptc ligand have been fabricated in molecular solvents, with different topologies involving diamond (dia), lonsdaleite (lon) and nbo.24 These three 4-connected topology frameworks based binuclear Zn clusters show poor stability in water.24 However, trinuclear Zn(II) clusters were formed in 1, leading to the condensed (4,8)-connected CaF2 topology with excellent hydrolytic stability. 1 exhibits high thermal stability and good chemical stability in water and NaOH solutions (pH range of 7–12), which allow it to detect antibiotics and nitroaromatic explosives in an aquatic system. It has been found that 1 represents high fluorescence quenching efficiency toward NFs (furazolidone, FZD; nitrofurazone, NZF; nitrofurantoin, NFT), NMs (ronidazole, RDZ; metronidazole, MDZ; dimetridazole, DTZ; ornidazole, ODZ) and nitrophenol (2-nitrophenol, 2-NP; 3-nitrophenol, 3-NP; 4-nitrophenol, 4-NP; 2,4,6-trinitrophenol, TNP) in water solution, respectively.
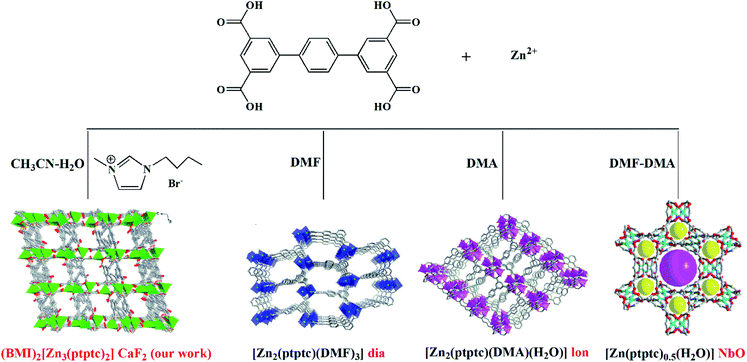 |
| Scheme 1 The synthetic routes for Zn-MOFs based on H4ptptc. | |
Experimental section
Materials and physical measurements
All reagents were of analytical grade and obtained from commercial sources without further purification. Elemental analysis for C and H were performed on a PerkinElmer 240 elemental analyzer. Thermal analyses were performed on a SDT 2960 thermal analyzer from room temperature to 800 °C at a heating rate of 20 °C min−1 under nitrogen flow. Powder X-ray diffraction (PXRD) data were collected on a Rigaku D/Max-2500PC diffractometer with Cu Kα radiation (λ = 1.5406 Å) over the 2θ range of 5–50° with a scan speed of 5° min−1 at room temperature. Room temperature PL spectra and time-resolved lifetime were conducted on an Edinburgh FLS1000 fluorescence spectrometer equipped with a xenon arc lamp (Xe900), nanosecond flash-lamp (nF900) and a microsecond flashlamp with time-resolved single photon counting-multi-channel scaling (MCS) mode. Fluorescence spectra for the aqueous solutions of each analyte were recorded on a Hitachi 850 fluorescence spectrophotometer.
Synthesis of (BMI)2[Zn3(ptptc)2] (1)
First step. ZnCl2 (0.2 mmol) and H4ptptc (0.10 mmol) were soaked in 1 mL [BMI]Br solutions in a glass vial (10 mL), and heated in an isotherm oven more than 100 °C until starting materials dissolved.
Second step. 4 mL mixed solvent of acetonitrile (CH3CN) and H2O (V/V = 1
:
1) was added, and then the homogeneous phase was transferred to a 25 mL Teflon-lined stainless steel vessel. The vessel was sealed and heated to 160 °C for 72 h and then cooled to room temperature at a rate of 5 °C h−1. Colorless block crystals of 1 were obtained in high yields (∼90%). Elemental analysis calcd (%) for 1 C60H50N4O16Zn3 (1279.21): C 56.34, H 3.94, N 4.38. Found: C 55.87, H 3.76, N 4.18.
X-ray crystallography
Single crystal X-ray diffraction analysis of 1 was carried out on an Oxford Diffraction Super Nova area-detector diffractometer using mirror optics monochromated Mo Kα radiation (λ = 0.71073 Å) at room temperature. Using Olex2,27 the structure was solved with the ShelXT28 structure solution program using Intrinsic Phasing and refined with the ShelXL29 refinement package using least squares minimisation. The hydrogen atoms were assigned with common isotropic displacement factors and included in the final refinement by use of geometrical restrains. The [BMI]+ cations were disordered and could not be located. The diffuse electron densities in the structure were therefore treated using the SQUEEZE routine in the program PLATON. The crystallographic data and selected bond lengths and angles for 1 are listed in Tables S1 and S2 in ESI.† Crystallographic data for the structural analyses have been deposited with the Cambridge Crystallographic Data Center. CCDC numbers for 1 is 1959055.
Experiments of detecting of antibiotics and nitroaromatic explosives in the aqueous phase
The fluorescence property of 1 was investigated in water emulsions at room temperature. The fine grinding MOF powder (2 mg) was dispersed in saturated aqueous solutions or a certain concentration (2 mL) of a series of antibiotics (0.1 mM) and nitroaromatics (1 mM), treated by ultrasonication for 30 min and then aged for 3 days to form a stable emulsion before the fluorescence studies. Twelve kinds of antibiotics, nitrofurazone (NZF), dimetridazole (DTZ), ornidazole (ODZ), nitrofurantoin (NFT), ronidazole (RDZ), chloramphenicol (CAP), sulfamethazine (SMZ), metronidazole (MDZ), furazolidone (FZD), sulfadiazine (SDZ), penicillin (PCL), and florfenicol (FFC), were detected. The nitroaromatics used in this study were 2-nitrophenol (2-NP), 3-nitrophenol (3-NP), 4-nitrophenol (4-NP), 2,4,6-trinitrophenol (TNP), nitrobenzene (NB), 2-nitrotoluene (2-NT), 2,4-dinitrotoluene (2,4-DNT), and 1,3-dintrobenzene (1,3-DNB). In fluorescence titration setup, 2 mg of 1 was dispersed in 2 mL aqueous solution, treated by ultrasonication for 30 min and then aged for 3 days for the next experiment. The fluorescence was measured in situ after incremental addition of freshly prepared 1 mM or saturated aqueous solutions of each analyte. The mixed solution was stirred at a constant rate during experiment to maintain its homogeneity. All the experiments were performed in triplicate and consistent results are reported. The quenching efficiency (%) was estimated using the formula (F0 − F)/F0 × 100%, where F0 and F are the maximum fluorescent intensity of 1 before and after the addition of analyte, respectively.
Results and discussion
Synthesis and crystal structure
The reaction of H4ptptc with ZnCl2 in a mixture of acetonitrile (CH3CN)/H2O/[BMI]Br (2/2/1) at 160 °C yields a novel type of Zn-MOF. The mineralizing agent [BMI]Br aids the solubility of the starting materials, and is required for high yields. Systematic exploration of several molecular solvents shows that 1 is only formed in CH3CN–H2O mixture. Other solvents, such as DMF or H2O or CH3CN, or DMF–H2O mixed solvent were also attempted, but only fine powder products were obtained. Besides, when using Zn(NO3)2 or ZnSO4 or other zinc(II) salts, only much unspecified impurities were obtained. In a typical synthesis procedure, we firstly dissolve ZnCl2 and H4ptptc in [BMI]Br, followed by adding CH3CN–H2O into the reaction system, and perform the reaction at 160 °C for 3 days. This facile procedure can produce crystals of 1 with a high yield and high crystal quality.
Single crystal X-ray analysis indicates that 1 crystallizes in triclinic crystal system P
space group. The asymmetry unit of 1 is composed of three crystallographically independent Zn(II) ions, two ptptc ligands and two free [BMI]+ cations. As shown in Fig. 1a, Zn1 coordinates to six oxygen atoms from six carboxylate groups of six different ptptc ligands to complete a distorted octahedral coordination geometry. Zn2 and Zn3 both connect with four oxygen atoms from four carboxylate groups of four different ptptc ligands, resulting in a distorted tetrahedral coordination sphere. Two types ptptc ligands both act as μ7-bridging, linking seven Zn(II) ions through two μ2-η1:η1, one μ2-η0:η2, and one μ1-η0:η1 coordination modes for four carboxylate groups, respectively. Zn1, Zn2 and Zn3 ions are joined together by eight carboxylate groups of eight ptptc ligands to form a trinuclear [Zn3(CO2)8] cluster, in which the Zn⋯Zn separations through carboxylate groups are 3.293 and 3.279 Å for Zn1⋯ Zn2 (−x, 1 − y, −z), Zn1⋯Zn3 (1 + x, 1 + y, z), respectively. These trinuclear [Zn3(CO2)8] clusters are extended by terphenyl groups of ptptc ligands generating a 3D anionic framework (Fig. 1b). The structure of complex 1 has channels of 7.0 × 8.1 Å dimensions with a void space running along [010] direction, which are occupied by highly disordered [BMI]+ cations as counter charges. Furthermore, topological analysis is carried out to get insight of the structure of 1. If [Zn3(CO2)8] cluster is considered as a eight-connected node, and ptptc ligand is regarded as a four-connected node, as shown in Fig. S1 in ESI,† the structure can be viewed as a metal–organic replica of fluorite with (4,8)-connected topology (CaF2).30
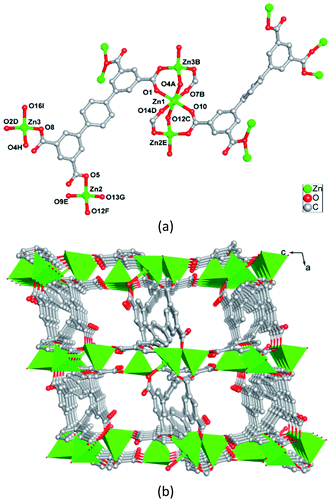 |
| Fig. 1 (a) Representation of the Zn(II) coordination environments in 1. Symmetry codes (A) 1 + X, +Y, +Z; (B) 1 + X, 1 + Y, +Z; (C)−1 + X, +Y, +Z; (D) −1 + X, −1 + Y, +Z; (E) −X, 1 − Y, −Z; (F) 1 − X, 1 − Y, −Z; (G) 1 − X, 2 − Y, −Z; (H) +X, −1 + Y, +Z; (I) 1 − X, 2 − Y, 1 − Z. (b) View of 3D coordination framework of 1. | |
The role of ILs
Unique physical and solvent properties of ILs may create special crystallization environments and provide structure-directing agents, which lead to quite different networks from those obtained by traditional hydro/solvothermal methods. Our past studies proved that ILs seem to be promising for the syntheses of novel MOFs that are inaccessible using traditional synthetic routes.23 As discussed above, [BMI]Br not only serves as solvent and structure-directing agent, but also enters the resulting structure as charge compensating agent.
H4ptptc possesses delocalized π-electron system which not only endows the MOFs with luminescent properties but also encourage π–π stacking to increase the framework stability.24–26 In addition, four carboxylic acid groups and the rigid terphenyl in H4ptptc can afford varied coordination modes to yield diverse topology structures.24–26 As shown in Scheme 1, there have been three types of Zn-MOFs based on H4ptptc ligand: [Zn2(ptptc)(DMF)3]·4H2O·5.5DMF (2), [Zn2(ptptc)(DMA)(H2O)]·2.5H2O·3.5DMA (3) and [Zn(ptptc)0.5(H2O)]·DMF·DMA (4), which are emanated from the different molecular solvents (DMF, DMA and mixture of DMF/DMA (1
:
1), respectively).24 The three types of Zn-MOFs present a permanent porosity with considerable potential void volume (about 39%–68% of the total crystal volume). Besides, the yields of the three Zn-MOFs are only about 30%. However, in our experiments, the crystalline product of Zn(II) ions and ptptc ligands turned out to be a condensed structure (BMI)2[Zn3(ptptc)2] (1), with good qualities and high yields (∼90%). The major advantage of [BMI]Br can probably tune the solvent properties to match those required for the synthesis of condensed structure.
Besides, different synthesis methods also lead to different topologies. The structures of the above mentioned three Zn-MOFs (2, 3 and 4) and 1 based on H4ptptc ligand are dependent on the different solvents (molecular solvent or ionic liquid). 2, 3 and 4 all exhibit 4-connected networks (considering binuclear Zn clusters and ptptc ligand both as the 4-connected nodes) with different topologies involving diamond (dia), lonsdaleite (lon) and nbo. However, trinuclear Zn(II) clusters were formed in 1 under the combination of ionothermal and hydro/solvothermal condition (our work). Each trinuclear Zn(II) cluster connects eight ptptc ligands and each ptptc ligand connects four trinuclear Zn(II) clusters, leading to the (4,8)-connected CaF2 topology. Interestingly, the distortion extents of ptptc ligands are found to be dependent on their topologies. Owing to being compressed, the ptptc ligands are seriously distorted in 1, with the larger dihedral angles between the intermediate benzene ring and adjacent two benzene rings in the ptptc ligand. In 1, the dihedral angles among the benzene rings in the two types ptptc ligands are 45.90° and 53.24°, 40.286° and 29.86°, respectively. While the dihedral angles in 2, 3 and 4 are about 30° or 0°, respectively.
Thermal and pH-stability
Considering practical applications, the stabilities of 1 to heat and pH were investigated. Thermogravimetric (TG) analysis shows that the framework of 1 has high thermal stability and start to decompose around 400 °C (Fig. S3 in ESI†). 1 also exhibits good chemical stability and PXRD results have suggested that 1 could remain stable in the pH range of 7–12 for three days (Fig. 2). The presence of a strong peak around 25° in alkali solutions may be due to variation in the preferred orientation of the powder samples during collection of the experimental PXRD data. However, a sharp impurity peak at about 2θ = 6°could be observed in acidic water with pH values of 2 and 6 (Fig. S2 in ESI†).
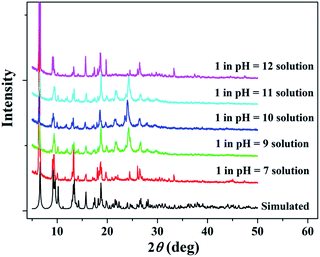 |
| Fig. 2 PXRD patterns for simulated and experimental 1 sample soaked in aqueous solutions over the pH range from 7 to 12. | |
Fluorescent properties
Luminescence spectroscopy as well as time-resolved luminescence lifetime in solid state were investigated. 1 displays an emission band at 403 nm, which is blue-shifted relative to the 417 nm band of H4ptptc ligand (Fig. S4 and S5 in ESI†). The fluorescence decay curve of 1 is fitted as a single exponential function with the lifetime of 7.79 ns for peak of 403 nm (Fig. S5 in ESI†). By the excitation of microsecond flash lamp (100 Hz), 1 displays a broad emission with two main peaks at 668 and 710 nm (Fig. S6 in ESI†). Time-resolved measurements revealed long lifetime of 400.48 and 359.26 μs, which can be assigned to phosphorescence emission (Fig. S6 in ESI†). The long lifetime of phosphorescence emission is due to the strong coordination bonds and dense stacking structure of 1 which provide a rigid matrix reducing the nonradiative loss of triplet excitons.31 We also examined the fluorescent properties of 1 dispersed in water and common organic solvent. As shown in Fig. S7 in the ESI,† the fluorescent emissions of 1 have slight solvent dependence, and 1 shows stronger emissions in H2O and CH3CN, respectively, and the emission spectra are close to those of solid state sample. The strong emission and stability of 1 in H2O suspension indicate potential to be utilized as antibiotics and nitroaromatic explosives sensor in the aqueous phase.
Detection of antibiotics and nitroaromatics in the aqueous phase
The high water stability together with good dispersibility and strong emissions of the micrometre-sized fine particles of 1 in water suspension, prompt us to explore its application in monitoring antibiotics and nitroaromatics in water. The fine grinding sample 1 was dispersed in 0.1 mM aqueous solutions of the selected twelve kinds of antibiotics. 1 has high quenching efficiencies toward NZF and DTZ, but very poor toward PCL and FFC (Fig. 3a, b and S8 in ESI†). Fig. 3b shows the percentage of fluorescence quenching in terms of adding a certain amount of different antibiotics at room temperature. NZF and DTZ give rise to the highest quenching efficiencies of 97 and 90%, respectively. ODZ, NFT, RDZ, CAP, SMZ, and MDZ also lead to relative high quenching efficiencies (>50%), whereas quenching efficiencies are low for the remaining antibiotics. To examine sensing sensitivity towards these antibiotics in more detail, fluorescence-quenching titrations were performed with gradually adding a certain amount of the analytes to suspensions of 1. For NZF, DTZ ODZ, NFT, RDZ, and FZD, the fluorescence quenching efficiency increased drastically with the analyte amount even in the low concentration range (Fig. 3c, S9–S20 in ESI†). The quenching efficiency can be quantitatively explained by the Stern–Volmer equation: (F0/F) = Ksv[Q] + 1, where Ksv is the quenching constant (ppm−1), [Q] is the concentration of the analyte, F0 and F are the fluorescent intensities before and after addition of the analyte, respectively. As indicated in Fig. S9–S20 in ESI,† the SV plots for the above-mentioned six kinds of antibiotics are nearly linear at low concentrations, and subsequently deviate from linearity, bending upwards at higher concentrations. Such phenomena of nonlinear SV plots might be attributed to self-absorption, a combination of static and dynamic quenching, or an energy-transfer process between the analytes and the MOFs.32 Linear SV plots are observed for all the other antibiotics over a wide concentration range. The higher Ksv values are 1.7 × 10−1 ppm−1 for NZF and 1.6 × 10−1 ppm−1 for DTZ, respectively, as verified by linear fitting of the SV plots at a low concentration range (Table S3 in ESI†). These Ksv values are comparable to those of Zr(IV)-based MOFs reported by Zhou and Li.12
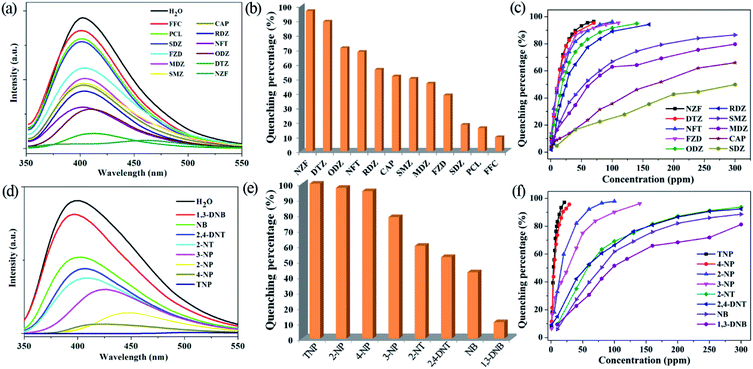 |
| Fig. 3 The emission spectra for 1 dispersed in 0.1 mM aqueous solutions of the selected antibiotics (a) and 1 mM aqueous solutions of the selected nitroaromatics explosives (d). Quenching efficiency of the fluorescent intensity for 1 dispersed in 0.1 mM aqueous solutions of the selected antibiotics (b) and 1 mM aqueous solutions of the selected nitroaromatics explosives (e). Quenching efficiencies of 1 dispersed in aqueous solutions at different concentrations of the selected antibiotics (c) and nitroaromatics explosives (f). | |
To date, a number of MOFs have been employed for various nitroaromatics explosives detection, but most of them work in organic solvent systems, not in water, due to the poor water stabilities of MOFs.5–14 The fine grinding sample 1 was dispersed in 1 mM or saturated aqueous solutions of a series of nitroaromatics. Significant fluorescence quenching of 1 occurs upon the addition of the selected four nitrophenol compounds (TNP, 2-NP, 4-NP and 3-NP), while 1,3-DNB shows a very low quenching effect (Fig. 3d, e and S21 in ESI†). As depicted in Fig. 3e, more than 95% of quenching efficiency could be achieved for TNP, 2-NP and 4-NP. In addition, 2-NT, 2,4-DNT and NB also lead to relative high quenching efficiencies (about 40–60%). The quenching efficiencies of 1 for the eight nitroaromatics follow the order of TNP > 2-NP > 4-NP > 3-NP > 2-NT > 2,4-DNT > NB > 1,3-DNB. In fluorescence titration, 1 shows fast detection and high fluorescence quenching for the nitrophenol compounds on incremental addition of 20 ppm for TNP, 30 ppm for 4-NP, 90 ppm for 2-NP and 140 ppm for 3-NP, respectively (Fig. 3f and S22–S25 in ESI†). Similar to those in detecting antibiotics, the SV plots for the four nitrophenol compounds are also nearly linear at low concentrations and subsequently deviated from linearity and bend upwards at higher concentrations (Fig. S22–S25 in ESI†). Whereas, the other nitroaromatics give linear SV plots over a wide concentration range (Fig. S26–S29, in the ESI†). 1 shows the higher Ksv values of 5.3 × 10−1 ppm−1 for TNP and 3.8 × 10−1 ppm−1 for 4-NP, respectively (Table S3 in ESI†). In addition, the Ksv value for TNP in 1 is significantly larger than that of our reported lanthanide MOFs, demonstrating a super quenching ability of 1.23b,23c,23e
The fluorescence quenching mechanism of nitro compounds
Generally, photoinduced electron transfer and/or energy transfer are regarded as the fluorescence quenching mechanism.5–14 Molecular orbital calculation can also be used to describe the valence-band (VB) and conduction-band (CB) energy levels of MOFs.5,6 In most cases, the CB energy levels of MOFs are higher than the lowest unoccupied molecular orbitals (LUMOs) of nitro compounds, which are low-lying π*-type orbitals stabilized by the NO2 group through conjugation. This facilitates electron transfer from the electron-rich framework to the LUMO of the electron-deficient nitro compounds upon photoexcitation, leading to fluorescence quenching.5–14 Fig. 4 shows the HOMO and LUMO orbital energies of antibiotics and nitroaromatics.8a,12,13b The lower the LUMO energy of the nitro compounds, the more easily electron transfer occurs and the higher quenching efficiency can be observed. In fluorescence titration processes, the maximum quenching efficiency observed for the four nitrophenol compounds (TNP, 2-NP, 4-NP and 3-NP), NZF and NFT are in good agreement with the LUMO energy levels of nitro analytes, but the order of observed quenching efficiency is not fully in accordance with the LUMO energies of other antibiotics and nitroaromatics. Thus, the photoinduced electron transfer is not the only mechanism, and an energy transfer should also exist in the fluorescence quenching processes observed in these systems.
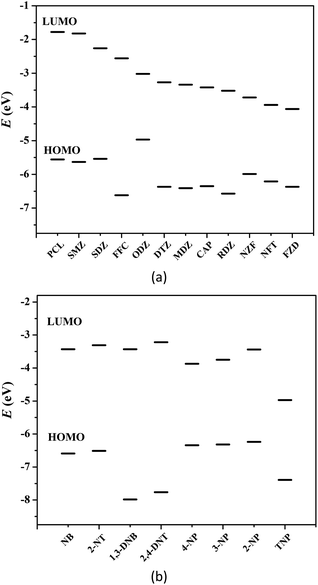 |
| Fig. 4 HOMO and LUMO energy levels for the selected antibiotics (a) and nitroaromatics explosives (b). | |
The nonlinearity of the SV plots for the four nitrophenol compounds, NZF and NFT, i.e., further indicate the existence of the resonance energy transfer from the MOF to these nitro compounds. A necessary condition of such energy transfer is the effective overlap between the absorption band of the analyte and the emission band of the MOF.5–14 The UV-vis absorption spectra of antibiotics and nitroaromatics in aqueous solution were measured. As shown in Fig. S30 in ESI,† the greatest spectral overlap between the absorption spectrum of NZF, NFT and FZD and the emission spectrum of 1 can be observed. For nitroaromatics, TNP has the greatest overlap, followed by 4-NP, 3-NP and 2-NP, while negligible spectral overlap for other nitroaromatics 1,3-DNB, NB, 2,4-DNT and 2-NT (Fig. S31 in ESI†). These results indicate that the long-range energy transfer may also play a key role in the fluorescence quenching process.5–14 As a result, 1 exhibits higher fluorescence quenching response towards the four nitrophenol compounds, NZF, NFT and FZD, owing to the coexistence of electron transfer and energy transfer.
Conclusions
In summary, an alkali-resistant Zn-MOF based on a π-electron-rich terphenyl-tetracarboxylic acid, (BMI)2[Zn3(ptptc)2] (1), has been synthesized under the combination of hydro/solvothermal and ionothermal condition. [BMI]Br not only serves as solvent to dissolve both organic and inorganic precursors and improve crystal yield compared to other ptptc-based Zn-MOFs, but also acts as structure-directing agent to mediate novel MOF. 1 exhibits good chemical stability in water and alkaline solution, which allow it to be used in an aquatic system. 1 shows remarkable sensitivity for nitro-explosives in a wide scope, particularly for phenol hydroxyl-containing nitro-explosives. Besides, 1 also displays a highly quenching response to antibiotics at fairly low responsive concentration. The orbital energy theoretical calculation and UV-vis absorption experiments indicate that quenching mechanism might be attributed to photo-induced electron transfer and fluorescence resonance energy transfer from the excited MOF to the electron deficient analytes adsorbed on the particles surface of 1.
Conflicts of interest
There are no conflicts to declare.
Acknowledgements
This work was supported by the National Natural Science Foundation of China (No. U1504202, 21971100 and 21771097), Project of Central Plains Science and Technology Innovation Leading Talents of Henan Province, and the Natural Science Foundation of Henan province (No. 182300410237).
Notes and references
-
(a) Q.-Q. Zhang, G.-G. Ying, C.-G. Pan, Y.-S. Liu and J.-L. Zhao, Environ. Sci. Technol., 2015, 49, 6772–6782 CrossRef CAS PubMed;
(b) H. Wang, N. Wang, J. Qian, L. Hu, P. Huang, M. Su, X. Yu, C. Fu, F. Jiang, Q. Zhao, Y. Zhou, H. Lin, G. He, Y. Chen and Q. Jiang, Environ. Sci. Technol., 2017, 51, 3518–3525 CrossRef CAS.
-
(a) M. E. Germain and M. J. Knapp, Chem. Soc. Rev., 2009, 38, 2543–2555 RSC;
(b) Y. Salinas, R. Martínez-Máñez, M. D. Marcos, F. Sancenón, A. M. Costero, M. Parra and S. Gil, Chem. Soc. Rev., 2012, 41, 1261–1296 RSC.
-
(a) K. Karim and S. K. Gupta, Water Res., 2003, 37, 2953–2959 CrossRef CAS PubMed;
(b) Z. She, M. Gao, C. Jin, Y. Chen and J. Yu, Process Biochem., 2005, 40, 3017–3024 CrossRef CAS.
- V. Uberoi and S. K. Bhattacharya, Water Environ. Res., 1997, 69, 146–156 CrossRef CAS.
- A. Lan, K. Li, H. Wu, D. H. Olson, T. J. Emge, W. Ki, M. Hong and J. Li, Angew. Chem., Int. Ed., 2009, 48, 2334–2338 CrossRef CAS PubMed.
-
(a) S. Pramanik, C. Zheng, X. Zhang, T. J. Emge and J. Li, J. Am. Chem. Soc., 2011, 133, 4153–4155 CrossRef CAS PubMed;
(b) Z. Hu, K. Tan, W. P. Lustig, H. Wang, Y. Zhao, C. Zheng, D. Banerjee, T. J. Emge, Y. J. Chabal and J. Li, Chem. Sci., 2014, 5, 4873–4877 RSC.
-
(a) D. Tian, Y. Li, R.-Y. Chen, Z. Chang, G.-Y. Wang and X.-H. Bu, J. Mater. Chem. A, 2014, 2, 1465–1470 RSC;
(b) X. J. Liu, X. Wang, J. L. Xu, D. A. Tian, R. Y. Chen, J. Xu and X. H. Bu, Dalton Trans., 2017, 46, 4893–4897 RSC.
-
(a) S. S. Nagarkar, B. Joarder, A. K. Chaudhari, S. Mukherjee and S. K. Ghosh, Angew. Chem., Int. Ed., 2013, 52, 2881–2885 CrossRef CAS;
(b) A. Karmakar, A. Kumar, A. K. Chaudhari, P. Samanta, A. V. Desai, R. Krishna and S. K. Ghosh, Chem.–Eur. J., 2016, 22, 4931–4937 CrossRef CAS.
-
(a) L. H. Cao, F. Shi, W. M. Zhang, S. Q. Zang and T. C. W. Mak, Chem.–Eur. J., 2015, 21, 15705–15712 CrossRef CAS;
(b) G. X. Wen, M. L. Han, X. Q. Wu, Y. P. Wu, W. W. Dong, J. Zhao, D. S. Li and L. F. Ma, Dalton Trans., 2016, 45, 15492–15499 RSC;
(c) H. R. Fu, X. X. Wu, L. F. Ma, F. Wang and J. Zhang, ACS Appl. Mater. Interfaces, 2018, 10, 18012–18020 CrossRef CAS;
(d) L.-H. Xie, X.-M. Liu, T. He and J.-R. Li, Chem, 2018, 4, 1911–1927 CrossRef CAS;
(e) H. He, Q.-Q. Zhu, F. Sun and G. Zhu, Cryst. Growth Des., 2018, 18, 5573–5581 CrossRef CAS.
-
(a) Y. Cui, Y. Yue, G. Qian and B. Chen, Chem. Rev., 2012, 112, 1126–1162 CrossRef CAS PubMed;
(b) L. E. Kreno, K. Leong, O. K. Farha, M. Allendorf, R. P. Van Duyne and J. T. Hupp, Chem. Rev., 2012, 112, 1105–1125 CrossRef CAS PubMed;
(c) D. Banerjee, Z. Hu and J. Li, Dalton Trans., 2014, 43, 10668–10685 RSC;
(d) Y. Cui, B. Chen and G. Qian, Coord. Chem. Rev., 2014, 273–274, 76–86 CrossRef CAS;
(e) Z. Hu, B. J. Deibert and J. Li, Chem. Soc. Rev., 2014, 43, 5815–5840 RSC.
-
(a) W. P. Lustig, S. Mukherjee, N. D. Rudd, A. V. Desai, J. Li and S. K. Ghosh, Chem. Soc. Rev., 2017, 46, 3242–3285 RSC;
(b) Y. Zhang, S. Yuan, G. Day, X. Wang, X. Yang and H.-C. Zhou, Coord. Chem. Rev., 2018, 354, 28–45 CrossRef CAS;
(c) J. He, J. Xu, J. Yin, N. Li and X.-H. Bu, Sci. China Mater., 2019, 62, 1655–1678 CrossRef CAS;
(d) P. L. Wang, L. H. Xie, E. A. Joseph, J. R. Li, X. O. Su and H. C. Zhou, Chem. Rev., 2019, 119, 10638–10690 CrossRef CAS PubMed.
- B. Wang, X. L. Lv, D. W. Feng, L. H. Xie, J. Zhang, M. Li, Y. B. Xie, J. R. Li and H. C. Zhou, J. Am. Chem. Soc., 2016, 138, 6204–6216 CrossRef CAS PubMed.
-
(a) M.-L. Han, G.-X. Wen, W.-W. Dong, Z.-H. Zhou, Y.-P. Wu, J. Zhao, D.-S. Li, L.-F. Ma and X. Bu, J. Mater. Chem. C, 2017, 5, 8469–8474 RSC;
(b) H. R. Fu, L. B. Yan, N. T. Wu, L. F. Ma and S. Q. Zang, J. Mater. Chem. A, 2018, 6, 9183–9191 RSC;
(c) H. R. Fu, Y. Zhao, T. Xie, M. L. Han, L. F. Ma and S. Q. Zang, J. Mater. Chem. C, 2018, 6, 6440–6448 RSC;
(d) X. Liu, B. Liu, G. Li and Y. Liu, J. Mater. Chem. A, 2018, 6, 17177–17185 RSC;
(e) Z.-H. Zhou, W.-W. Dong, Y.-P. Wu, J. Zhao, D.-S. Li, T. Wu and X.-h. Bu, Inorg. Chem., 2018, 57, 3833–3839 CrossRef CAS PubMed.
-
(a) Z.-S. Qin, W.-W. Dong, J. Zhao, Y.-P. Wu, Q. Zhang and D.-S. Li, Inorg. Chem. Front., 2018, 5, 120–126 RSC;
(b) Y. Zhou, Q. Yang, D. Zhang, N. Gan, Q. Li and J. Cuan, Sens. Actuators, B, 2018, 262, 137–143 CrossRef CAS;
(c) Z. Zhou, M.-L. Han, H.-R. Fu, L.-F. Ma, F. Luo and D.-S. Li, Dalton Trans., 2018, 47, 5359–5365 RSC;
(d) J.-H. Wei, J.-W. Yi, M.-L. Han, B. Li, S. Liu, Y.-P. Wu, L.-F. Ma and D.-S. Li, Chem.–Asian J., 2019, 14, 3694–3701 CrossRef CAS PubMed.
-
(a) R.-W. Huang, Y.-S. Wei, X.-Y. Dong, X.-H. Wu, C.-X. Du, S.-Q. Zang and T. C. W. Mak, Nat. Chem., 2017, 9, 689–697 CrossRef CAS PubMed;
(b) Y.-P. Wu, G.-W. Xu, W.-W. Dong, J. Zhao, D.-S. Li, J. Zhang and X. Bu, Inorg. Chem., 2017, 56, 1402–1411 CrossRef CAS;
(c) M. M. Xu, X. J. Kong, T. He, X. Q. Wu, L. H. Xie and J. R. Li, Inorg. Chem., 2018, 57, 14260–14268 CrossRef CAS;
(d) K. Fan, S.-S. Bao, W.-X. Nie, C.-H. Liao and L.-M. Zheng, Inorg. Chem., 2018, 57, 1079–1089 CrossRef CAS;
(e) B. J. C. Wong, D.-m. Xu, S.-S. Bao, L.-M. Zheng and J. Lei, ACS Appl. Mater. Interfaces, 2019, 11, 12986–12992 CrossRef PubMed.
-
(a) H. L. Jiang, D. Feng, K. Wang, Z. Y. Gu, Z. Wei, Y. P. Chen and H. C. Zhou, J. Am. Chem. Soc., 2013, 135, 13934–13938 CrossRef CAS PubMed;
(b) S. M. T. Abtab, D. Alezi, P. M. Bhatt, A. Shkurenko, Y. Belmabkhout, H. Aggarwal, L. J. Weselinski, N. Alsadun, U. Samin, M. N. Hedhili and M. Eddaoudi, Chem, 2018, 4, 94–105 CrossRef;
(c) E. A. Kapustin, Chem, 2018, 4, 16–17 CrossRef CAS;
(d) L. N. McHugh, M. J. McPherson, L. J. McCormick, S. A. Morris, P. S. Wheatley, S. J. Teat, D. McKay, D. M. Dawson, C. E. F. Sansome, S. E. Ashbrook, C. A. Stone, M. W. Smith and R. E. Morris, Nat. Chem., 2018, 10, 1096–1102 CrossRef CAS PubMed;
(e) J.-W. Ye, X.-Y. Li, H.-L. Zhou and J.-P. Zhang, Sci. China: Chem., 2018, 62, 341–346 CrossRef.
-
(a) J. Cui, Y. Li, Z. Guo and H. Zheng, Chem. Commun., 2013, 49, 555–557 RSC;
(b) H.-R. Fu, F. Wang and J. Zhang, Dalton Trans., 2015, 44, 2893–2896 RSC;
(c) Y.-N. Gong, T. Ouyang, C.-T. He and T.-B. Lu, Chem. Sci., 2016, 7, 1070–1075 RSC;
(d) Y. L. Hu, M. L. Ding, X. Q. Liu, L. B. Sun and H. L. Jiang, Chem. Commun., 2016, 52, 5734–5737 RSC.
-
(a) E. Ahmed and M. Ruck, Dalton Trans., 2011, 40, 9347–9357 RSC;
(b) D. Freudenmann, S. Wolf, M. Wolff and C. Feldmann, Angew. Chem., Int. Ed., 2011, 50, 11050–11060 CrossRef CAS;
(c) J. Zhang, L. Peng and B. Han, Soft Matter, 2014, 10, 5861–5868 RSC;
(d) B. Zhang, J. Zhang and B. Han, Chem.–Asian J., 2016, 11, 2610–2619 CrossRef CAS PubMed.
-
(a) K. Jin, X. Huang, L. Pang, J. Li, A. Appel and S. Wherland, Chem. Commun., 2002, 2872–2873 RSC;
(b) D. N. Dybtsev, H. Chun and K. Kim, Chem. Commun., 2004, 1594–1595 RSC;
(c) J. Zhang, S. Chen and X. Bu, Angew. Chem., Int. Ed., 2008, 47, 5434–5437 CrossRef CAS;
(d) S. M. Chen, J. Zhang, T. Wu, P. Y. Feng and X. H. Bu, J. Am. Chem. Soc., 2009, 131, 16027–16029 CrossRef CAS;
(e) J. Zhang, T. Wu, S. Chen, P. Feng and X. Bu, Angew. Chem., Int. Ed., 2009, 48, 3486–3490 CrossRef CAS.
-
(a) H. Ren, T. Ben, E. Wang, X. Jing, M. Xue, B. Liu, Y. Cui, S. Qiu and G. Zhu, Chem. Commun., 2010, 46, 291–293 RSC;
(b) W. X. Chen, H. R. Xu, G. L. Zhuang, L. S. Long, R. B. Huang and L. S. Zheng, Chem. Commun., 2011, 47, 11933–11935 RSC;
(c) Y. Kang, S. Chen, F. Wang, J. Zhang and X. Bu, Chem. Commun., 2011, 47, 4950–4952 RSC;
(d) Q.-Y. Liu, Y.-L. Wang, N. Zhang, Y.-L. Jiang, J.-J. Wei and F. Luo, Cryst. Growth Des., 2011, 11, 3717–3720 CrossRef CAS;
(e) D.-Y. Du, J.-S. Qin, C.-X. Sun, X.-L. Wang, S.-R. Zhang, P. Shen, S.-L. Li, Z.-M. Su and Y.-Q. Lan, J. Mater. Chem., 2012, 22, 19673 RSC;
(f) J. Liu, F. Zhang, X. Zou, G. Yu, N. Zhao, S. Fan and G. Zhu, Chem. Commun., 2013, 49, 7430–7432 RSC.
-
(a) J. Liu, X. Zou, C. Liu, K. Cai, N. Zhao, W. Zheng and G. Zhu, CrystEngComm, 2016, 18, 525–528 RSC;
(b) Y. Li, Z. Yang, Y. Wang, Z. Bai, T. Zheng, X. Dai, S. Liu, D. Gui, W. Liu, M. Chen, L. Chen, J. Diwu, L. Zhu, R. Zhou, Z. Chai, T. E. Albrecht-Schmitt and S. Wang, Nat. Commun., 2017, 8, 1354 CrossRef PubMed;
(c) X. X. Sang, J. L. Zhang, J. F. Xiang, J. Cui, L. R. Zheng, J. Zhang, Z. H. Wu, Z. H. Li, G. Mo, Y. Xu, J. L. Song, C. C. Liu, X. N. Tan, T. Luo, B. X. Zhang and B. X. Han, Nat. Commun., 2017, 8, 7 CrossRef;
(d) T. Zheng, Z. Yang, D. Gui, Z. Liu, X. Wang, X. Dai, S. Liu, L. Zhang, Y. Gao, L. Chen, D. Sheng, Y. Wang, J. Diwu, J. Wang, R. Zhou, Z. Chai, T. E. Albrecht-Schmitt and S. Wang, Nat. Commun., 2017, 8, 15369 CrossRef CAS.
-
(a) W.-W. Xiong, J.-R. Li, B. Hu, B. Tan, R.-F. Li and X.-Y. Huang, Chem. Sci., 2012, 3, 1200 RSC;
(b) Z. P. Wang, J. Y. Wang, J. R. Li, M. L. Feng, G. D. Zou and X. Y. Huang, Chem. Commun., 2015, 51, 3094–3097 RSC.
-
(a) J. H. Qin, Y. Jia, H. Li, B. Zhao, D. Wu, S. Zang, H. Hou and Y. Fan, Inorg. Chem., 2014, 53, 685–687 CrossRef CAS PubMed;
(b) J. H. Qin, B. Ma, X.-F. Liu, H.-L. Lu, X.-Y. Dong, S.-Q. Zang and H. Hou, J. Mater. Chem. A, 2015, 3, 12690–12697 RSC;
(c) J. H. Qin, B. Ma, X. F. Liu, H. L. Lu, X. Y. Dong, S. Q. Zang and H. Hou, Dalton Trans., 2015, 44, 14594–14603 RSC;
(d) J. H. Qin, H. R. Wang, Q. Pan, S. Q. Zang, H. Hou and Y. Fan, Dalton Trans., 2015, 44, 17639–17651 RSC;
(e) J. H. Qin, H. R. Wang, M. L. Han, X. H. Chang and L. F. Ma, Dalton Trans., 2017, 46, 15434–15442 RSC;
(f) J. H. Qin, Y. D. Huang and F. F. Li, Inorg. Nano-Met. Chem., 2019, 49, 7–11 CrossRef CAS;
(g) J. H. Qin, Y. D. Huang, Y. Zhao, X. G. Yang, F. F. Li, C. Wang and L. F. Ma, Inorg. Chem., 2019, 58, 15013–15016 CrossRef CAS.
-
(a) J. Yang, L. Zhang, X. Wang, R. Wang, F. Dai and D. Sun, RSC Adv., 2015, 5, 62982–62988 RSC;
(b) J.-C. Jin, J. Wu, Y.-X. He, B.-H. Li, J.-Q. Liu, R. Prasad, A. Kumar and S. R. Batten, CrystEngComm, 2017, 19, 6464–6472 RSC.
-
(a) R.-X. Yao, X. Cui, X.-X. Jia, F.-Q. Zhang and X.-M. Zhang, Inorg. Chem., 2016, 55, 9270–9275 CrossRef CAS;
(b) L.-L. Gao, Q.-N. Zhao, M.-M. Li, L.-M. Fan, X.-Y. Niu, X.-Q. Wang and T.-P. Hu, CrystEngComm, 2017, 19, 6651–6659 RSC.
-
(a) Y.-L. Gai, F.-L. Jiang, L. Chen, Y. Bu, K.-Z. Su, S. A. Al-Thabaiti and M.-C. Hong, Inorg. Chem., 2013, 52, 7658–7665 CrossRef CAS;
(b) J. Lu, C. Perez-Krap, F. Trousselet, Y. Yan, N. H. Alsmail, B. Karadeniz, N. M. Jacques, W. Lewis, A. J. Blake, F.-X. Coudert, R. Cao and M. Schroeder, Cryst. Growth Des., 2018, 18, 2555–2562 CrossRef CAS.
- O. V. Dolomanov, L. J. Bourhis, R. J. Gildea, J. A. K. Howard and H. Puschmann, J. Appl. Crystallogr., 2009, 42, 339–341 CrossRef CAS.
- G. Sheldrick, Acta Crystallogr., Sect. A: Found. Adv., 2015, 71, 3–8 CrossRef.
- G. Sheldrick, Acta Crystallogr., Sect. C: Struct. Chem., 2015, 71, 3–8 Search PubMed.
- R. Q. Zou, R. Q. Zhong, M. Du, T. Kiyobayashi and Q. Xu, Chem. Commun., 2007, 2467–2469 RSC.
-
(a) X. Liu, R. Li, L. Ma, X. Feng and Y. Ding, New J. Chem., 2016, 40, 619–625 RSC;
(b) X. Yang and D. Yan, Chem. Sci., 2016, 7, 4519–4526 RSC;
(c) A. Y. Ni, Y. Mu, J. Pan, S. D. Han, M. M. Shang and G. M. Wang, Chem. Commun., 2018, 54, 3712–3714 RSC;
(d) Y. Zhao, X.-G. Yang, X.-M. Lu, C.-D. Yang, N.-N. Fan, Z.-T. Yang, L.-Y. Wang and L.-F. Ma, Inorg. Chem., 2019, 58, 6215–6221 CrossRef CAS PubMed;
(e) X. G. Yang, X. M. Lu, Z. M. Zhai, Y. Zhao, X. Y. Liu, L. F. Ma and S. Q. Zang, Chem. Commun., 2019, 55, 11099–11102 RSC;
(f) X. G. Yang, Z. M. Zhai, X. M. Lu, Y. Zhao, X. H. Chang and L. F. Ma, Dalton Trans., 2019, 48, 10785–10789 RSC.
-
(a) S. M. Aly, M. R. Parida, E. Alarousu and O. F. Mohammed, Chem. Commun., 2014, 50, 10452–10455 RSC;
(b) A. a. O. El-Ballouli, E. Alarousu, M. Bernardi, S. M. Aly, A. P. Lagrow, O. M. Bakr and O. F. Mohammed, J. Am. Chem. Soc., 2014, 136, 6952–6959 CrossRef CAS PubMed.
Footnote |
† Electronic supplementary information (ESI) available: Sturcture figure, TG plot, fluorescence spectra, phosphorescence spectra, details of detecting of the selected antibiotics and nitroaromatic explosives, crystal data, selected bond lengths and angles, summary of quenching constants, HOMO and LUMO energies calculated for the analytes. CCDC 1959055. For ESI and crystallographic data in CIF or other electronic format see DOI: 10.1039/c9ra08733h |
|
This journal is © The Royal Society of Chemistry 2020 |