Characterization of a commercially-available, low-pressure UV lamp as a disinfection system for decontamination of common nosocomial pathogens on N95 filtering facepiece respirator (FFR) material†
Received
27th April 2020
, Accepted 23rd June 2020
First published on 24th June 2020
Abstract
A commercially-available UV surface disinfection system used for hospital room disinfection was characterized for use as a UV-inactivation system for N95 filtering facepiece respirator (FFR) respirators. The output of light was initially characterized and assessed for its ability to penetrate through the individual N95 respirator layers. Following characterization, disinfection performance was tested using a range of model pathogens. In a series of experiments, coupons of respirator material were inoculated with Escherichia coli, Staphylococcus aureus, Geobacillus stearothermophilus spores, Bacillus cereus, Pseudomonas aeruginosa, and influenza A virus. Inoculated coupons were then treated with UV light from a low-pressure UV disinfection system during specific treatment times. Microbial enumeration was performed pre- and post-UV treatment. It was found that respirator coupons inoculated with E. coli, influenza A, P. aeruginosa, B. cereus and G. stearothermophilus resulted in below detection counts when exposed to UV fluences between 410 to 2700 mJ cm−2. In addition, UV light was unable to sufficiently penetrate all layers of respirator material, highlighting the importance of flipping respirators when exposing them to UV light. This work suggests that with further validation, UV light will be a promising touchless treatment option for frontline professionals who need to repurpose N95 respirators for subsequent use.
Water impact
UV disinfection research in the water sector has paved the way for a comprehensive understanding of methods to repurpose personal protection equipment using this technology. Considering UV disinfection through a wider lens has revealed that the use of UV light can be an effective, suitable and touchless treatment option for disinfection and reuse of N95 masks.
|
1.0 Introduction
The application of ultraviolet (UV) light for microbial inactivation purposes has been integral in food, air, and water purification systems. With recent events, UV is now considered as a promising alternative to disinfect and reuse personal protective equipment (PPE) in the global fight against the novel severe acute respiratory syndrome coronavirus 2 (SARS-CoV-2) pandemic. COVID-19, the illness caused by SARS-CoV-2, is transmitted through respiratory droplets from coughs and sneezes of infected individuals.1 The N95 filtering facepiece respirator (FFR), a single-use disposable respirator approved by the National Institute for Occupational Safety and Health (NIOSH), is indicated for health care personnel when performing aerosol-generating medical procedures (AGMP) in patients with potential COVID-19 infections.2 Amid the foreseeable depletion of disposable PPE, there is a growing international shortage of N95 respirators for AGMP, which may in part account for the high incidence of COVID-19 infection in health care workers.3 The urgent need for a safe and effective method for decontaminating N95 respirators that allows them to be returned to service cannot be overstated.
A successful N95 FFR decontamination method must effectively disinfect used respirators in a way that is harmless to end-users while retaining respirator integrity for multiple uses.4 In a Technical Bulletin released in April 2020, 3M, a leading manufacturer of N95 respirators, does not recommend the use of ethylene oxide (due to concerns related to off-gassing), ionizing radiation (due to risk in compromising filter performance), microwave (due to damage of the respirator near metal components, resulting in compromised fit), or the use of high temperature, autoclave, or steam (due to significant filter degradation).5 While the Centers for Disease Control and Prevention (CDC) and NIOSH do not recommend decontamination of FFRs for reuse as standard care, consideration for this alternative is given when FFR shortages exist in times of crisis. In a timely summary of the impact of various FFR treatment strategies on their disinfection efficacy and ability to maintain respirator integrity, CDC lists UV treatment one of the methods showing the most promise.6 A recent systematic review has shown that 43 N95 FFR models that received UV treatment maintained NIOSH certification standards following disinfection.7 In addition to its inherent efficacy and ability to preserve N95 respirator integrity, UV disinfection is a form of “touchless technology” which bears several notable advantages: UV disinfection can be administered without altering a room's ventilation; the treatment does not leave behind any residue; and it provides a broad spectrum of action with rapid disinfection cycles.8 As such, UV inactivation offers a promising approach to decontaminate used N95 respirators in a safe, timely, and effective manner for their essential return to service.
UV disinfection of high-touch surfaces has been investigated in hospital settings and compared to standard operating procedures (SOPs). In a teaching hospital, the effectiveness of UV disinfection versus SOP, in which cleaning staff applied a chlorine-based detergent followed by a chlorine-based disinfectant (active chlorine 2800 mg L−1) was tested in reducing total bacterial count (TBC) and elimination of high-concern microorganisms.8 Of 345 sampling sites across five high-touch surfaces, only 18% of samples tested positive after UVC treatment compared to 63% after the SOP. In hospital isolation units, disinfection via ceiling- and wall-mounted UVC light sources (with dosages ranging from 16 to 1923 mJ cm−2) was compared with standard hospital environmental cleaning (with non-antibacterial soap and water) and chemical disinfection (5% chloramine) to determine the bactericidal effect on contaminated surfaces. Although some shadowed areas (e.g. bed rail, lockers, and mattresses) required additional chemical disinfection, UVC disinfection significantly reduced the number of bacteria on surfaces exposed to UVC.9
UV disinfection occurs when the nitrogenous bases of nucleic acids strongly absorb UV radiation and are damaged by resulting photochemical reactions.10 Gram-negative bacteria are most susceptible to UV treatment, followed by Gram-positive, fungi, and bacterial spores.11 In one study involving comparisons of UV254 sensitivities among a wide range of viruses, sensitivity ranged from 0.11 to 25 mJ cm−2 and depended on the size and type of nucleic acid (i.e. base composition and whether the virus genome comprised double-stranded DNA, single-stranded DNA, double-stranded RNA, or single-stranded RNA).12 In the absence of data on the UV susceptibility of SARS-CoV-2, Derraik et al. (2020)13 described several studies in which UV treatment was effective for disinfection of SARS-CoV-1, a related virus from the same species. However, the efficacy of the applied dose was influenced by several factors, including inoculum size, culture medium, and shape and type of material.13 For the purpose of disinfecting used N95 respirators, a sterilization technique effective for a wide range of infectious agents is required, as the respirators may be contaminated by a variety of pathogens from not only infected patients, but also from the individual wearing the respirator. A fluence of at least 1000 mJ cm−2 has been reported for achieving disinfection of a used N95 respirator.14–16
Many jurisdictions have issued policy statements concerning safe approaches to repurpose N95 respirators. Relevant to our study, Health Canada released a notice on 08 April 2020 outlining minimum requirements for decontamination processes.17 Specifically, for tests to reduce pathogen burden, bacterial sporicidal testing (e.g. Geobacillus stearothermophilus spores) and viral inactivation testing (e.g. SARS-CoV-2, SARS-CoV-1, H1N1, influenza A/PR/8/34) with a 4
log reduction must be included. Biosafety level-2 (BSL-2) bacteria or viruses, agents having moderate potential hazard to personnel and the environment, as surrogates may also be considered. Health Canada also described additional recommendations to ensure the performance of the respirators is maintained (e.g. particle filtration efficacy, breathability, and valve leak (where applicable), and respirator fit testing). Moreover, this notice from Health Canada reports that the US Center for Disease Control (CDC) is encouraging the development of UV disinfection as one of the most promising methods of FFR decontamination.
In addition, the U.S. Food and Drug Administration (US FDA) has issued a guidance document outlining important regulatory considerations for reprocessing this valuable form of PPE.18 In this document, the US FDA discusses the hierarchy for decontamination and bioburden reduction systems for surgical masks and/or respirators that require different tiers of evidence levels. Moreover, a variety of microorganisms with required log inactivation (>6
log or >3
log, depending on the target tier) and the type of repurposed mask (single or multiple users) are also considered. As the worldwide pandemic evolves, it is that likely that new regulations and guidelines will appear regarding PPE disinfection and reuse protocols.
The overall objective of this study was to characterize and test the performance of a commercially-available UV reactor that is used for hospital room disinfection in surface disinfection of N95 respirator material. To meet this objective, seven specific aims were outlined, in accordance with the Health Canada requirements: (i) characterization of the commercial UV disinfection system by measuring irradiance and calculating fluence at different unit configurations; (ii) investigation of UV light penetration through different N95 respirator layers; (iii) disinfection performance for inoculum applied onto individual respirator layers using Escherichia coli; (iv) disinfection of influenza A virus; (v) disinfection of Geobacillus stearothermophilus spores; (vi) disinfection performance for inoculum embedded within respirator layers using Staphylococcus aureus; and (vii) disinfection of Bacillus cereus and Pseudomonas aeruginosa, two common hospital pathogens.
2.0 Materials and methods
2.1 UV disinfection system
The UV source chosen for this study was a commercially available UV disinfection system (MoonBeam3 by Diversey™), which was previously acquired by the Nova Scotia Health Authority (NSHA) for disinfecting hospital rooms and enclosures. This UV disinfection system is an EPA- and -FDA certified portable device with three 95 W, low-pressure, individually adjustable, articulating arms that can be positioned at almost any angle to direct UV light. N95 respirators (3M 1870+ N95) were obtained from the NSHA as well. For disinfection experiments, N95 respirator coupons were made by cutting the respirators into multiple 3 cm2 sections.
2.2 Set-up and characterization of the UV disinfection system
The first specific aim of this study was to characterize a commercially available UV disinfection system and establish a light-tight room that was suitable to house the reactor. This UV disinfection system was the source of UV radiation for all experiments. The system is designed for terminal room disinfection and for workspaces where patients who are carriers of severely transmissible and harmful pathogens have been treated. The system is controlled by a central tower that is placed outside of the area of disinfection. The lamps on the unit are programmable by a control panel located on the light tower. The device can be programmed to emit light for 90, 180, 300, and 600 second disinfection cycles.
The bulbs equipped to the system are low-pressure, 253.7 nm peak emittance mercury–halogen lamps. The light source was characterized using a spectroradiometer (Ocean Optics USB4000) with an integration range between 240 and 300 nm. The spectroradiometer, equipped with a 3648-element linear silicon CCD array detector, was placed in the room with the light source and was connected via USB to a laptop situated outside of the room, which allowed monitoring of the lamp without risk of UV exposure to research personnel. The output of the LP UV lamp was measured each day of experimentation to monitor the function and consistency of the lamp. No noticeable degradation of the output of light was observed during experimentation. The spectroradiometer was also placed in the reactor room during experimentation to ensure that the light source was functioning throughout the specified disinfection cycle that was in use. The reactor was placed in a 220 × 190 cm room with false walls composed of an outer surface of reflective aluminum foil to ensure the working space was light-tight and the geometry of the room was kept consistent.
Irradiance was measured in 10 cm increments ranging from 28 cm (minimum lamp to surface distance) to 78 cm (maximum lamp to surface distance) to account for changes in fluence across the surface of a full respirator. The change in UV intensity in relation to bulb height has been included in ESI† Table S1. The shortest and greatest distances between the UV lamp and treatment surface were measured as approximately 28 and 78 cm, respectively. These two different lamp elevation arrangements were further characterized to understand the minimum and maximum functional output of the lamp and can be seen in Fig. 1. The 78 cm distance was used for all experiments as the fluences that were achieved fell within the relevant irradiances (at least 1000 mJ cm−2) that have been observed by other studies.14–16
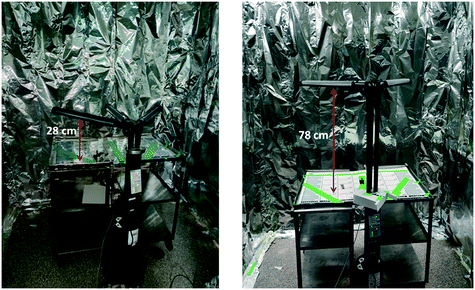 |
| Fig. 1 Configuration and characterization of the UV disinfection system using a spectroradiometer at the UV treatment room. The commercial UV system (MoonBeam3, Diversey™) with initial set-up in UV treatment room for measuring irradiance at minimum height (28 cm), left, and maximum height (78 cm), right. | |
The average irradiances were determined by measuring the light output at nine points across the array of lamp arms. Each lamp arm had three sampling locations, one point at the bulb terminal nearest the reactor hub, one point in the middle of the bulb (lengthwise), and one final point at the end of the bulb. The average irradiance of nine measurements for each arm height was used to calculate an estimated UV fluence (fluence = irradiance × time) for each disinfection cycle. These experimentally determined values were used to calculate the fluence for each disinfection cycle following methods outlined by Bolton and Linden.19 The fluence for each UV disinfection cycle (90, 180, 300, and 600 seconds) was calculated by multiplying the average irradiance by each disinfection cycle time.
2.3 Investigation of UV light penetration through different N95 respirator layers
The next task for this work was to identify the minimum acceptable performance of the UV reactor (quantity, time, and fluence) to achieve a high protective respirator disinfection factor. First, the UV light penetration through the different respirator layers was investigated. A 3M 1870+, N95 respirator was the main model of respirator used for this work. All three layers of this respirator model are constructed of hydrophobic polyurethane (Fig. 2). The outermost layer (L1) is a textured cloth-like material. The middle layer (L2) is only present on the main panel of the respirator and is more rigid than the other two layers, having a plastic-like feel. The innermost layer (L3) is the thickest layer of the respirator, measuring approximately 3 mm, and has a felted texture. Upon observation and accordingly with previous literature,20 it was determined that L3 was comprised of many layers that are not readily separable and any attempts to further separate the layer damaged the integrity of the filter. In this study, L3 was considered as a single respirator layer.
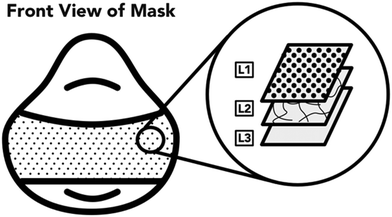 |
| Fig. 2 Description of the hydrophobic polyurethane layers of the 3M 1870/1870+ N95 respirators. | |
A coupon of individual (L1, L2, or L3) or combinations (L1 + L2 + L3, L1 + L2, L1 + L3, L2 + L3, L3 + L2, or L3 + L2 + L1) of respirator layers was clipped to the surface of the spectroradiometer detector. The detector was then exposed to light from UV disinfection system and the irradiance was recorded. The spectroradiometer was placed in the center of the UV lamp array with the UV lamps located at the same distance from the treated surface as all other experiments (78 cm).
2.4 Disinfection performance for inoculum applied onto individual respirator layers using Escherichia coli
E. coli DH5a was grown from frozen stock overnight at 37 °C and 180 RPM in Luria–Bertani (LB) broth. The following day, an inoculum of the overnight culture was added to fresh LB and grown under the same conditions for approximately 4 hours. E. coli was then transferred to a centrifuge tube and centrifuged for 10 min at 3000 RPM until a pellet was formed. LB broth was decanted, and the pellet was resuspended in phosphate buffered saline (PBS) by vortexing for 1 min.
Two replicates of each respirator coupon layer (L1, L2, and L3, as described in section 2.3) were inoculated by placing nine 5 μL droplets of E. coli (1 × 107 CFU mL−1) suspended in PBS on top of each layer using a 20 μL micropipette. Two replicates for positive control per UV fluence (six in total) and three replicates as negative control were used for this experiment. Following UV exposure, the respirator samples were placed in 20 mL PBS, vortexed for 1 minute at 3000 RPM for resuspension, and enumerated via track dilution plate technique using a 10 μl sample on LB agar and overnight incubation at 37 °C.21,22 The experiment was performed at the three lowest disinfection cycles (90, 180, and 300 seconds). A diagram of the experimental set-up is illustrated in Fig. 3.
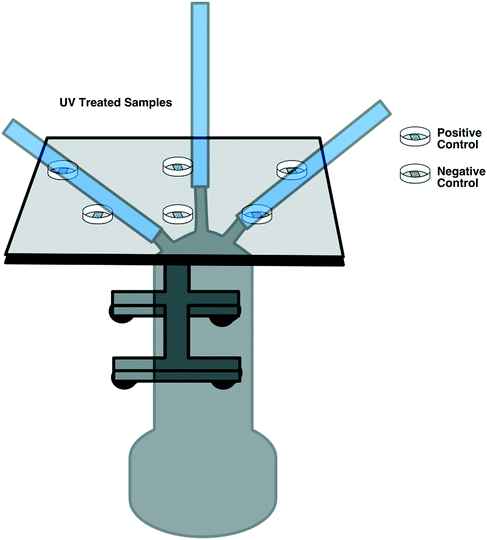 |
| Fig. 3 Diagram of experimental plan of E. coli validation of the UV disinfection system. Petri dishes beneath the UV light source contained single N95 respirator coupons inoculated with test microorganisms receiving UV treatment. The positive control sample represents a respirator coupon inoculated with test microorganisms that does not receive UV treatment. The blank negative control sample represents a respirator coupon without any inoculation that receives no UV treatment. | |
2.5 Surface disinfection of influenza A virus on respirator material
To assess surface UV disinfection of influenza A virus, N95 respirator coupons were inoculated in with 2.25 × 105 plaque forming units (PFU) of the laboratory-adapted influenza A virus A/PuertoRico/8/34/(H1N1) bearing two amino acid substitutions in the M2 ion channel protein (T27V, N31S) that renders the virus sensitive to amantadine. For UV-treated samples and positive controls, triplicate respirator coupons were inoculated. For each replicate, nine 5 μL droplets of influenza A virus were placed on top of a single, randomly-selected respirator layer (i.e. L1, L2, or L3). Preliminary experiments revealed that results were not impacted by the respirator layer tested (data not shown); therefore, randomly-selected respirator layers were chosen for experiments. Pieces of 2.5 × 2.5 cm respirator coupons were inoculated in a 3 × 3 grid of 5 μL droplets similar to the illustration in Fig. 4 (arrangement C). The inoculated single-layer coupons were then placed in under the center of each of the arms of the UV disinfection system. The experiment was performed at all four disinfection cycles (90, 180, 300, and 600 seconds).
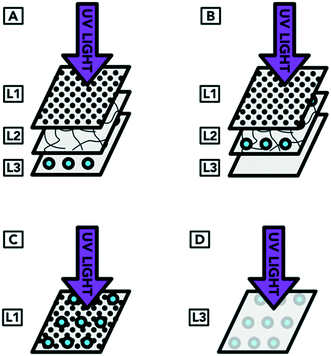 |
| Fig. 4 Diagram of experimental plan of validation of the UV disinfection system with S. aureus embedded within the layers of the N95 respirator. Arrangement A represents droplets containing the targeted organism reaching the innermost layer of an N95 respirator. Arrangement B represents droplets containing the targeted organism that have reached the middle layer of an N95 respirator. Arrangement C represents droplets containing the targeted organism landing on the outside of a worn respirator. Arrangement D represents a contaminated respirator that was treated but had not been flipped to expose all surfaces to UV radiation. | |
Following UV exposure, coupons were briefly vortexed in 5 mL of infection media (Dulbecco's modified Eagle's medium (DMEM); Thermo Fisher Scientific, Ottawa, ON, Canada) supplemented with 0.5% endotoxin-free bovine serum albumin (Sigma Aldrich Canada), 100 U mL−1 penicillin + 100 μg mL−1 streptomycin + 20 μg mL−1 glutamine (Pen/Strep/Gln; Wisent Bioproducts, St-Bruno, QC, Canada) and stored at 4 °C for three days prior to initiating the plaque assay.23 Madin–Darby canine kidney (MDCK) cells were maintained in DMEM supplemented with 10% fetal bovine serum (FBS, Thermo Fisher Scientific, Grand Island, NY, USA) and Pen/Strep/Gln at 37 °C in a 5% CO2 atmosphere. MDCK cells were seeded in 24-well cluster dishes and incubated overnight prior to infection with serial 10-fold dilutions of the UV-treated (or control) viral inoculum in infection media. Cells were rocked periodically for one hour, washed twice with PBS, and overlaid with infection medium containing 1.2% Avicel RC 591 (FMC Corporation, Philadelphia, PA, USA). After 48 h, cell monolayers were washed twice with PBS, fixed with 5% paraformaldehyde for 30 minutes and stained with 1% crystal violet for 5 min, prior to rinsing with water to reveal plaques.
2.6 Surface disinfection of Geobacillus stearothermophilus spores on respirator material
G. stearothermophilus spores were obtained from the biological indicator of 3M™ Attest™ Monitoring Starter Kit. Three UV disinfection cycles (180, 300, and 600 seconds) were tested for treating G. stearothermophilus spores on a single layer (i.e. L1, L2, or L3, randomly-selected) inoculated with nine 5 μL droplets of the inoculum. A single respirator coupon for each a positive control and a UV-treated sample was prepared. Following UV exposure, the respirator coupons were placed in 20 mL PBS and vortexed for one minute at 3000 RPM for resuspension. Samples were enumerated via track dilution plate technique using a 10 μL sample on tryptic soy agar (TSA) and tryptic soy broth (TSB), followed by incubation at 55 °C for 24 hours.
2.7 Disinfection performance for inoculum embedded within respirator layers using Staphylococcus aureus
Experiments for assessing the impact of light penetration through the layers of the respirator were performed by embedding S. aureus within the layers of the respirator. Although S. aureus is most likely found on the skin of a respirator user and is not a product of aerosolization, it was selected for this light penetration experiment because it was readily available. Moreover, the electrostatically-charged layers of the respirator material prevents both penetration and release of droplet nuclei,24 treatment of S. aureus embedded within the N95 respirator layers was used only to test the ability of UV light to disinfect a model organism through each of the individual respirator layers rather than to reflect likely respirator contamination scenarios.
S. aureus was grown overnight at 37 °C and 180 RPM on TSB, sub-cultured and incubated and washed in similar conditions as E. coli (explained above). For the UV-treated samples, duplicate coupons per arrangement type were prepared. Positive controls were prepared in duplicate: two for arrangement A and two for arrangement D. One negative control was prepared using L2. The experiment was carried out at two disinfection cycles (180 and 600 seconds). Respirator coupons were inoculated by placing nine 5 μL droplets of S. aureus (1 × 108 CFU mL−1) suspended in TSB on top of each layer using a 20 μL micropipette. Treated respirator samples were placed in 20 mL PBS, vortexed for one minute at 3000 RPM for resuspension, and enumerated via spot plate technique using 10 μl of sample on to sheep blood agar (SBA plates, BBL™ Trypticase™ soy agar with 5% sheep blood (TSA II)) and overnight incubation at 37 °C.
The inoculation was carried out in four arrangements. In arrangement A, droplets containing the organism were applied to the surface of L3 (which represents the inoculum reaching the innermost layer of an N95 respirator). The respirator layers were then reassembled so that the inoculum was covered by L2 and L1 (which was the original arrangement of layers of a complete respirator). In arrangement B, the droplets were applied to the surface of L2 (representing droplets containing the targeted organism that have reached the middle layer of an N95 respirator). When the respirator was reformed, the inoculum on the surface of L2 was covered by L1. Arrangement C represents droplets containing the targeted organism landing on the outside of a worn respirator. In this case, the UV light was not shielded by any respirator layer covering the organism. Arrangement D represented a contaminated respirator from the inside of L3 (i.e. from the individual wearing the respirator) that was treated but had not been flipped to expose all surfaces to UV radiation. As preliminary experiments showed that L3 alone blocked more than 95% of the UV light, only L3 (without the covering layers) was exposed to UV light for arrangement D. The inoculation procedure showing the different layers of the respirator is illustrated in Fig. 4.
2.8 Disinfection of Bacillus cereus and Pseudomonas aeruginosa
N95 respirator coupons comprised of L3 were artificially contaminated with two common hospital pathogens, Bacillus cereus, a Gram-positive spore forming bacilli, and Pseudomonas aeruginosa, a frequent cause of nosocomial infections. High and low concentrations (approximately 104 and 102 CFU mL−1) of B. cereus were tested for disinfection at four UV disinfection cycles. To more faithfully recapitulate a real respirator contamination and treatment scenario, bacteria were sprayed on both sides of 2.5 × 5 cm sections of respirator material. For treated samples, triplicates were prepared for each microorganism and parameter (UV fluence and initial concentration of microorganism); positive controls were prepared in duplicate, and a single negative control sample for each concentration was prepared (Table 1). Both sides of the respirator sections were treated with UV. Following UV treatment, each respirator coupons was aseptically placed into thioglycolate broth and briefly vortexed. Thioglycolate broths (TGB) were incubated at 35 °C and examined daily for turbidity. A terminal subculture was carried out after 72 hours of incubation in TGB. Viable colony counts were determined by making serial dilutions of the inoculum suspension, plating out 1 and 10 μL aliquots of each dilution on 5% SBA, and incubating for 24 hours at 35 °C.
Table 1 Experimental plan for validation of the UV disinfection system for inactivation of common nosocomial pathogens on N95 filtering facepiece respirator (FFR) material
Microorganism |
Inoculation technique |
Sample type |
UV cycle (s) |
Respirator layer/arrangement |
Replicates (n) |
Initial concentration |
Enumeration technique |
RSL = randomly-selected layer.
Initial concentration of G. stearothermophilus spores stock from commercial kit is unknown.
|
E. coli DH5a |
Surface |
Positive control |
None |
L1, L2 and L3 |
L3 × 3, L2 × 1, L1 × 2 |
1 × 108 CFU mL−1 |
Track dilution plate technique using 10 μl of sample on to LB agar plate, overnight incubation, 37 °C |
Negative control |
None |
L1, L2 and L3 |
1 per layer |
Treated |
90 |
L1, L2 and L3 |
2 per layer |
180 |
L1, L2 and L3 |
2 per layer |
300 |
L1, L2 and L3 |
2 per layer |
600 |
L1, L2 and L3 |
2 per layer |
S. aureus (ATCC 25923) |
Embedded |
Positive control |
None |
A and D |
2 |
1 × 108 CFU mL−1 |
Track dilution plate technique using 10 μl of sample on to SBA agar plate, overnight incubation, 37 °C |
2 |
Negative control |
None |
RSLa |
1 |
Treated |
180 |
A, B, C, D |
2 per layer |
600 |
A, B, C, D |
2 per layer |
G. stearothermophilus spores from 3M™ Attest™ Monitoring Starter Kit |
Surface |
Positive control |
None |
RSL |
1 |
NAb |
Cultured in TSA plates and TSB |
Treated |
180 |
RSL |
1 |
300 |
RSL |
1 |
600 |
RSL |
1 |
Influenza A virus (A/PR/8/34(H1N1)) |
Surface |
Positive control |
None |
RSL |
3 |
5 × 106 PFU mL−1 |
Plaque assays |
Treated |
90 |
RSL |
3 |
180 |
RSL |
3 |
300 |
RSL |
3 |
600 |
RSL |
3 |
Bacillus cereus (clinical isolate) |
Sprayed |
Positive control |
None |
L3 |
2 |
1 × 102 CFU mL−1 |
72 hours incubation in thioglycolate at 35 °C, examined daily for turbidity |
Negative control |
None |
L3 |
1 |
Treated |
90 |
L3 |
2 |
180 |
L3 |
2 |
300 |
L3 |
2 |
600 |
L3 |
2 |
Positive control |
None |
L3 |
2 |
1 × 104 CFU mL−1 |
Negative control |
None |
L3 |
1 |
Treated |
90 |
L3 |
2 |
180 |
L3 |
2 |
300 |
L3 |
2 |
600 |
L3 |
2 |
P. aeruginosa (ATCC 27853) |
Sprayed |
Positive control |
None |
L3 |
2 |
1 × 102 CFU mL−1 |
72 hours incubation in thioglycolate at 35 °C, examined daily for turbidity |
Negative control |
None |
L3 |
1 |
Treated |
90 |
L3 |
2 |
180 |
L3 |
2 |
300 |
L3 |
2 |
600 |
L3 |
2 |
Positive control |
None |
L3 |
2 |
1 × 104 CFU mL−1 |
Negative control |
None |
L3 |
1 |
Treated |
90 |
L3 |
2 |
180 |
L3 |
2 |
300 |
L3 |
2 |
600 |
L3 |
2 |
2.9 Summary of experiments
A summary of all disinfection experiments for each microorganism is detailed in Table 1. The type of inoculation is listed as “surface” (applied to the respirator layer surface in 5 μL droplets by micropipette), “embedded” within the layers of the respirator, or “sprayed” on the respirator material in a mist. Sample type refers to positive controls (inoculated samples that did not receive UV treatment), negative controls (samples receive neither inoculation nor UV treatment) or treated (inoculated samples that receive UV treatment). The UV cycle refers to one of the four disinfection cycles (90, 180, 300, or 600 seconds) of the UV reactor. Each coupon was treated at a specific fluence (0, 412, 824, 1373, or 2746 mJ cm−2, which correspond to disinfection cycle times of 0, 90, 180, 300, and 600 seconds, respectively), then flipped for exposure on the other side for the same amount of fluence of the respective cycle time.
Respirator layer arrangement refers to which of the three respirator layers was treated (L1, L2, L3, or randomly-selected layer (RSL)) and their subsequent arrangement prior to UV treatment. It should be noted that most experiments involved inoculation and treatment of a single respirator layer while those involving S. aureus described inoculation of a single respirator layer which was then combined with one or two other respirator layers prior to UV treatment. Furthermore, experiments began within an hour of respirator coupon inoculation. The number of replicates per sample type is listed, as well as initial concentration of the microorganism applied to the respirator coupons and the technique used for enumeration.
3.0 Results and discussion
3.1 Characterization of the commercial UV disinfection system
Average irradiances for the commercial UV disinfection system (i.e. MoonBeam3) were determined as 112 W m−2 at the closest distance (28 cm) and 45.8 W m−2 at the furthest distance (78 cm) relative to the exposure surface. The spectroradiometer sensor was 10 cm closer to the light source compared to the surface of exposure and this difference in height was considered when calculating UV fluence. The maximum UV intensity of the lamp did not vary based on the chosen disinfection cycle of UV exposure. Using average irradiance, fluence values were calculated in accordance with the method outlined by Bolton and Linden for each disinfection cycle of the UV reactor (Table 2).
Table 2 Estimated fluences for each of the disinfection cycles of the UV reactor
Lamp height (cm) |
Cycle time (s) |
Fluence (mJ cm−2) |
Irradiance (W m−2) |
28 |
90 |
1010 |
112 |
180 |
2019 |
300 |
3365 |
600 |
6730 |
78 |
90 |
412 |
45.8 |
180 |
824 |
300 |
1373 |
600 |
2746 |
3.2 UV light penetration through different N95 respirator layers
Investigation of UV light penetration through the different layers of the N95 respirator was carried out using the UV disinfection system at a distance of 78 cm from the samples (corresponds to a 68 cm irradiance reading from the spectroradiometer sensor) at a fluence rate of 45.8 W m−2. L1, L2 and L3 block 62, 34, and 95% of UV light, respectively. Together, all three layers block 100% of UV light when shone from the outside of the respirator and 99% when UV light was delivered from the inside of the respirator (Table 3). The light penetration data highlight the importance of flipping respirators when exposing them to UV light. UV light was unable to sufficiently penetrate all layers of respirator material and therefore would not provide a fluence capable of disinfection.
Table 3 UV light penetration through different respirator layers: L1 (outer layer), L2 (middle flexible layer), and L3 (inner layer, user interface). L2 and L3 comprise filtering medium
Respirator material layers tested |
Layer configurationa |
Fluence rate (W m−2) |
Proportion of blocked light (%) |
Order of the layers denotes the direction of light penetration.
|
Individual layer |
No layer |
42.6 |
— |
L1 |
16.3 |
62 |
L2 |
28.3 |
34 |
L3 |
2.0 |
95 |
Combined layers |
No layer |
48.4 |
— |
L1 + L2 + L3 |
0.0 |
100 |
L1 + L2 |
8.0 |
84 |
L1 + L3 |
0.3 |
99 |
L2 + L3 |
0.3 |
99 |
L3 + L2 |
0.7 |
99 |
L3 + L2 + L1 |
0.6 |
99 |
3.3 Disinfection performance for inoculum applied onto individual respirator layers using Escherichia coli
The results of the first round of disinfection performance tests of the UV disinfection system using E. coli are shown in Fig. 5 (additional information can be found in ESI† Table S2). The average CFU mL−1 that was recovered from the positive control samples was 3.9 × 105 ± 1.7 × 105. All treated samples resulted in counts below the detection limit for E. coli after UV treatment at all disinfection cycles used, regardless of which layer was inoculated. This indicated that the type of respirator layer inoculated did not impact disinfection performance. Overall, there was consistently no E. coli growth in any of the UV treated N95 respirator samples in the three tested disinfection cycles of the UV reactor (0 CFU/10 μL). Therefore, at least 5
log inactivation was achieved in this experiment with the quantification method used. These results are consistent with previous findings that the UV fluence required for a 4
log inactivation of E. coli was between 5 and 11 mJ cm−2.25
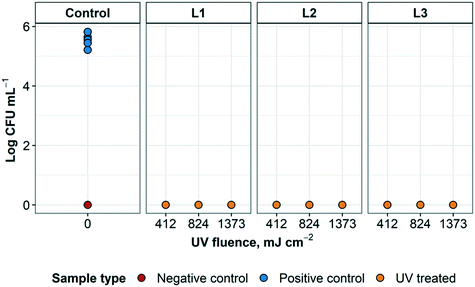 |
| Fig. 5 Standard plate count results of N95 respirator sample layers L1, L2, and L3 inoculated with E. coli following UV treatment at three disinfection cycles: 90, 180 and 300 seconds. UV treated and positive control, n = 2. Negative control, n = 1. | |
3.4 Disinfection of influenza A virus
UV treatment was effective in inactivating influenza A virus at each exposure time. It was not possible to observe a dose inactivation curve because the lowest UV fluence treated (412 mJ cm−2) was sufficient to inactivate all virus to below detection levels; at higher fluences, treated samples remained below the detection limit, as expected (ESI† Fig. S1). These results indicate >5
log reduction of influenza A virus infectivity, as shown in Fig. 6.
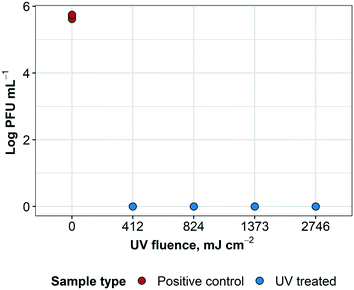 |
| Fig. 6 Results of UV treatment of influenza A virus on N95 respirator material coupons. A randomly-selected layer was inoculated with influenza A virus and treated with the UV radiation at four disinfection cycles: 90, 180, 300 and 600 seconds in triplicate samples. UV treated and positive control samples, n = 3. Two measurements per sample. | |
The results obtained in this study aligned with results found in previous literature with similar viruses. For example, Darnell et al. (2004) found >6
log reduction of SARS-CoV-1 infectivity at a fluence of approximately 1445 mJ cm−2 over an exposure time of 6 minutes.26 Darnell et al. (2004)26 found no further decrease of infectivity when the virus was exposed to higher UV radiation. Heimbuch et al. (2011) applied influenza A/PR/8/34 virus aerosols and droplets onto FFRs and exposed them to a fluence of 1800 mJ cm−2.27 This resulted in >4
log reduction of viable influenza virus against both the droplet and aerosol challenges for all treated samples, as well as all UV treated samples below detection limits. This is comparable to findings from Lore et al. (2010) which involved aerosolized influenza (A/H5N1) on FFRs exposed to a fluence of 1980 mJ cm−2.28 All samples resulted in >4.5
log inactivation of the virus. For these previous studies and our investigation into a commercial system, it was found the measured log inactivation (>4
log and >4.5
log) was a result of the high starting concentration of the influenza virus and the virus was reduced to values below the detection limit for all UV treated samples.
3.5 Disinfection of Geobacillus stearothermophilus spores
Results reveal that the UV treatment was effective at inactivating the spores for each exposure time. Treated samples and controls were cultured in plates and liquid broth for confirmation (ESI† Fig. S2). The control plate supported growth as expected and the liquid (broth) cultures were also consistent with the plate results. Positive controls resulted in a recovered concentration of G. stearothermophilus of 3.2 × 104 CFU mL−1. UV treated samples resulted in no bacterial growth and therefore at least 3.5
log inactivation of G. stearothermophilus at 412 mJ cm−2, as well as higher UV fluences, as expected. UV fluence (Fig. 7). These results are consistent with previous findings that greater than 4
log inactivation of G. stearothermophilus in PBS was achieved at a fluence of about 1 J mL−1, which converts to approximately 1000 mJ cm−2 (ref. 29) (1 J mL−1 ≈ 1.019 mJ cm−2, according to Keyser et al.30).
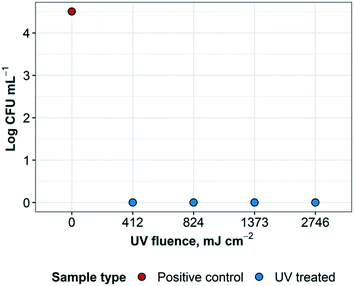 |
| Fig. 7 Results of UV treatment of G. stearothermophilus spores on N95 respirator material coupons. A randomly-selected layer was inoculated with G. stearothermophilus spores and treated at four disinfection cycles (90, 180, 300 and 600 seconds) of UV radiation. UV treated and positive control, n = 1. | |
3.6 Disinfection performance for inoculum embedded within respirator layers using Staphylococcus aureus
The results of the disinfection of S. aureus embedded within the layers of the N95 respirator using the UV disinfection system are shown in Fig. 8 (additional information can be found in ESI† Table S3). For type A arrangement, in which L1 and L2 were blocking 83.57% of the UV light applied to the inoculum, 2.4
log inactivation was achieved at 824 mJ cm−2 and no bacterial growth (0 CFU/10 μL) at 2746 mJ cm−2. In this case, when accounting for the amount of light being blocked by the respirator layers, only 118.9 and 396.2 mJ cm−2 were reaching the inoculum at 180 and 600 s disinfection cycles, respectively. For type B arrangement, in which it was only possible to have a single sample (without replicates), 2.3 and 3.2
log inactivation was achieved at 824 and 2746 mJ cm−2, respectively. Accounting for 61% of the light being blocked, only 321.3 and 1070.9 mJ cm−2 were reaching the inoculum at 180 and 600 s UV disinfection cycles, respectively. For type C arrangement, where UV light was not blocked, 2.3 and 2.5
log inactivation were achieved with 824 and 2746 mJ cm−2 applied, respectively. There were no differences in disinfection between the control samples and those from the type D layer arrangement in which the inoculum was beneath layer 3. This was in accordance with the large amount of UV light blocked by L3 (95.3%).
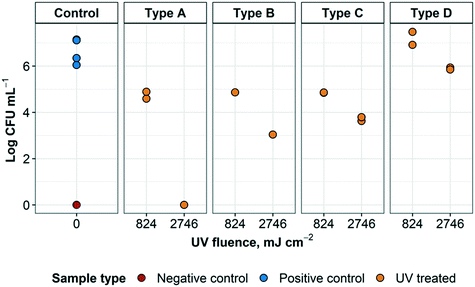 |
| Fig. 8 UV treatment of S. aureus embedded within N95 respirator layers. The different sample types refer to the arrangement of respirator layers and where the inoculum was placed, as detailed in Fig. 6. Arrangement A represents droplets containing the targeted organism reaching the innermost layer of an N95 respirator. Arrangement B represents droplets containing the targeted organism that have reached the middle layer of an N95 respirator. Arrangement C represents droplets containing the targeted organism landing on the outside of a worn respirator. Arrangement D represents a contaminated respirator that was treated but had not been flipped to expose all surfaces to UV radiation. For each arrangement, n = 2 for positive controls, except for arrangement B (n = 1). For negative controls, n = 1. | |
Some of these results are expected; however, it was interesting to see that a higher log inactivation was achieved on type A and type B arrangements, where L1 and/or L1 and L2 were blocking the light from the inoculum, rather than arrangement type C, in which the light was not blocked at all. Conversely, only UV disinfection of arrangement type D displayed almost zero log inactivation. Some of these unpredicted results may be attributed to a number of reasons (e.g. dilution error, issues with inoculation, etc.) and further experimentation would be required to substantiate or challenge these findings. Nevertheless, it was promising to see that it was possible to achieve 2.3 and 2.4
log inactivation at a UV fluence of 824 mJ cm−2 and between 2.5 and up to >6
log inactivation at a UV fluence of 2746 mJ cm−2. Previous studies have demonstrated that the UV fluence required to achieve a 4 and 5
log inactivation of Staphylococcus spp. (including S. aureus) in greywater were 13.0 and 20.9 mJ cm−2, respectively.31
3.7 Disinfection of Bacillus cereus and Pseudomonas aeruginosa
After 72 hours, no growth of any type was detected in the TGB for any UV-treated specimen (except for one sample contaminated post-processing with coagulase negative Staphylococci). This was later confirmed with sample plating (data not shown) for all four treatment variations (Fig. 9). All negative controls remained negative for the 72 h incubation. For positive controls, concentrations of 7 × 102 and 2 × 104 CFU mL−1 for B. cereus and 5.9 × 102 and 9.0 × 103 for P. aeruginosa were recovered from low and high initial concentrations, respectively. For this experiment, >2.8 and >4.3
log inactivation was observed for B. cereus and >2.7 and >3.9
log inactivation was achieved for P. aeruginosa, respectively. It was not possible to further quantify log inactivation because quantification limit of the enumeration method was reached.
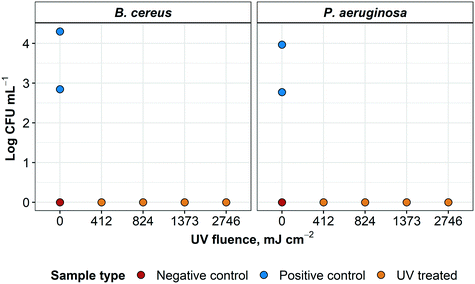 |
| Fig. 9 Results of terminal subculture of UV treated N95 respirator material coupons inoculated with of B. cereus and P. aeruginosa. L3 pieces of respirators were inoculated and treated at four disinfection cycles (90, 180, 300 and 600 seconds) of UV radiation. Treated samples, positive and negative controls were done in triplicate (n = 3). The UV fluence indicated for each treatment group was applied to each side of the respirator coupons. | |
All four treatment modalities were successful in sterilizing L3 coupons of N95 respirators grossly contaminated with either B. cereus or P. aeruginosa. Surface disinfection of these healthcare-associated microorganisms inoculated on L3 coupons was successfully achieved using a commercially-available UV LP lamp. These results were consistent with previous findings that a 4
log inactivation of P. aeruginosa required a UV fluence of 1100 mJ cm−2.25 Although B. cereus is not often used in decontamination studies,32 inactivation of this microorganism in dried fishery products is expected to require 600 to 900 mJ cm−2.33
In addition, the bacterial deposition method used for P. aeruginosa and B. cereus (spray) did not impact the level of disinfection when compared to E. coli, influenza virus, G. stearothermophilus experiments where coupons were inoculated using droplets, since all of the UV treated samples resulted in zero counts. This is in accordance with a study by Heimbuch et al. (2011), where six different respirator models were studied and the difference in respirator material or the inoculation method did not appear to impact UV disinfection efficacy.27
3.8 Assessing additional risks of donning a UV-disinfected N95 respirator
The “N95” filter rating from NIOSH indicates that N95 FFRs demonstrate a minimum efficiency level of 95%;34 in terms of risk to the user, this means that up to 5% of particles may penetrate the layers of a brand new N95 respirator (ESI† Fig. S3). The material of the N95 respirator is comprised of three types of layers: a hydrophobic outer layer, middle electrostatically-charged layers, and an inner biocompatible layer for user comfort.35 The function of the hydrophobic outer layer is to prevent droplets (i.e. from coughs, sneezes, or AGPs) from penetrating deeper into the electrostatically-charged layers. However, droplet nuclei may penetrate the outer hydrophobic layer and become trapped in the electrostatic layers of the material.
In a 2012 study by Fisher et al. examining reaerosolization of MS2 bacteriophage from N95 FFRs during simulated coughing, less than 0.0001% of captured droplets and less than 0.21% of droplet nuclei were released from the respirator material.24 The authors stated that the risk of user exposure due to reaerosolization associated with extended use were considered negligible for most respiratory viruses, as air drag forces produced by inhalation or coughing are not sufficient to overcome adhesion forces that hold trapped particles within the filter fibers. Additionally, in an online seminar by the International Ultraviolet Association entitled, “Expert Perspectives on UV as a Tool for N95 Decontamination”, it was stated that the risk of wearing a decontaminated respirator was essentially the same as that of using a brand new respirator.35 It has been noted in the literature that N95 respirators and other PPE that are visibly soiled are not suitable candidates for reuse, as the presence of organic matter may impede disinfection.13
The very nature of N95 respirator material prevents penetration of 95% of particles past the protective hydrophobic and electrostatic layers, as well as subsequent release of particles that end up trapped within the layers of the respirator. Although this study shows that UV light penetration through the layers of the respirator is minimal, so, too, is the penetration of pathogen-laden droplets. As such, disinfection experiments involving embedded bacteria and viruses are not considered practically-relevant representations of respirator disinfection as this type of inoculation does not accurately represent particle penetration into the layers of the respirator.
3.9 Future validation of a method for UV-disinfection of N95 respirators
This study provides evidence to support the potential application of UV disinfection as a suitable and touchless treatment option for healthcare professionals and their strategy against the spread and proliferation of SARS CoV-2. The data presented in this study serves as proof-of-concept for UV-disinfection of a range of microorganisms inoculated onto N95 respirator material. To validate an N95 respirator disinfection method, a series of rigorous validation studies must be carried out (including repeated experiments with intact N95 respirators, airflow testing and fit testing of the respirators, and number of viable disinfection cycles) with a sufficient number of replicates required to meet quality assurance/quality control criteria.
A validated UV disinfection method for N95 respirator reuse must be capable of reaching acceptable levels of inactivation for model organisms. At the time of writing this paper, there does not seem to be a unanimous agreement in the literature on an acceptable level of inactivation. Although a recent US FDA document suggests 6
log inactivation for certain microorganisms,18 a starting concentration of at least 107 CFU mL−1 would be required to demonstrate this level of inactivation. In this study, even though spores (G. stearothermophilus), Gram positive bacteria (B. cereus), Gram negative bacteria (E. coli, P. aeruginosa), and enveloped virus (influenza A) resulted in below detection limits after UV disinfection, the 6
log inactivation recommended by the US FDA could not be verified. This limitation is related to the initial concentration of target microorganisms rather than the capacity of UV disinfection. To clearly demonstrate a >6
log inactivation of any microorganism, the initial concentration should be around 107 CFU mL−1, which is unlikely to be representative of the real bioburden in a used N95 FFR. Furthermore, using an initial concentration of 107 CFU mL−1 would result in turbid inoculum, which could potentially interfere with UV light exposure, distribution, and penetration, due to possible shielding of bacteria. On the other hand, a >4
log inactivation of influenza A virus was achieved, which is aligned with the current Health Canada guidelines regarding viral inactivation testing.36
SARS-CoV-2 has been characterized as an enveloped, positively charged, single-stranded ribonucleic acid (RNA) virus (60 to 140 nm) of the beta coronavirus genus.37,38 Based on its characteristics, it has been estimated that a fluence of 0.28 mJ cm−2 will inactivate 99% of the viral load (D1).39 This value is in accordance with the previously predicted range of 0.25 to 0.39 mJ cm−2 as the D37 (one lethal hit per virus) for the entire coronavirus family.12 In addition, Favero (2001) stated that lipid or medium-sized viruses are the least resistant microorganisms to disinfection, followed by vegetative bacteria, fungi, non-lipid or small viruses, mycobacteria, and bacterial spores.40 Considering the amount of UV light that reaches the inner layers of the respirator material when treated with 1000 mJ cm−2 from both sides is at least 37.3 mJ cm−2 (ref. 35) (two orders of magnitude higher than the estimated fluence required to kill a member of the coronavirus family), it is deemed unlikely that SARS-CoV-2 would survive UV treatment at the fluence delivered in these experiments. However, more studies about SARS-CoV-2 UV spectral sensitivity and kinetics are required to draw more definitive conclusions.
4.0 Conclusions
A commercially available UV disinfection system intended for patient room disinfection was fully characterized for application in N95 respirator material disinfection. Coupons of N95 FFR material were inoculated with different microorganisms and configurations in a series of experiments, with each experiment focusing on a different challenge organism type. Microbial enumeration was performed pre and post UV treatment with promising results. UV light was unable to sufficiently penetrate all layers of respirator material, highlighting the importance of turning respirators over when exposing them to UV light. This observation indicates that the respirator layers themselves may inhibit full disinfection of microorganisms trapped within the respirator material. However, due to the unique protective properties of the material of an intact N95 respirator, assessing disinfection of embedded microorganisms inoculated onto physically separated respirator layers (as demonstrated with S. aureus in this study) is not a purposeful objective that portrays a real-life contamination scenario. Based on this important consideration and anticipation of further method validation, UV treatment at the fluences tested in this work was found to be a promising technology in the surface decontamination process for respirator reuse.
Conflicts of interest
There are no conflicts to declare.
Acknowledgements
The authors acknowledge the coordination and technical support from many individuals in the Nova Scotia Health Authority. In addition, the authors acknowledge funding support from the NSERC COVID-19 Alliance Grant and the NSERC/Halifax Water Industrial Research Chair program. The authors would also like to extend appreciation to Dr. Richard Simons (AquiSense Technologies) for technical advice and support during the project. As well, the following researchers from the Centre for Water Resources Studies at Dalhousie University assisted with technical reviews during the study: Dr. Benjamin Trueman, Lindsay Anderson, Kyle Rauch, David Shoults and Jessica Bennett.
References
-
S. Pawar, A. Stanam, M. Chaudhari and D. Rayudu, Effects of temperature on COVID-19 transmission, medRxiv, 2020, Mar 30, DOI:10.1101/2020.03.29.20044461.
-
World Health Organization, Infection prevention and control during health care when novel coronavirus (nCoV) infection is suspected - Interim guidance [Internet], 2020, [cited 2020 Apr 26]. Available from:https://www.who.int/publications-detail/infection-prevention-and-control-during-health-care-when-novel-coronavirus-(ncov)-infection-is-suspected-20200125 Search PubMed.
-
A. Kumar, S. B. Kasloff, A. Leung, T. Cutts, J. E. Strong and K. Hills, et al.N95 Mask Decontamination using Standard Hospital Sterilization Technologies [Internet], Winnipeg, Manitoba, 2020, [cited 2020 Apr 6]. Available from: https://news.umanitoba.ca/n95-mask-decontamination-using-standard-hospital-sterilization-technologies/ Search PubMed.
-
A. Price and L. Chu, COVID-19 Evidence Service | Addressing COVID-19 Face Mask Shortages [v1.1] [Internet], Standford Medicine, 2020, Mar [cited 2020 Apr 6]. Available from: https://m.box.com/shared_item/https%3A%2F%2Fstanfordmedicine.box.com%2Fv%2Fcovid19-PPE-1-1 Search PubMed.
-
3M Company, Technical Bulletin: Decontamination Methods for 3M N95 Respirators [Internet], 3M, St. Paul, MN, 2020, Ar, p. 2, Available from: https://multimedia.3m.com/mws/media/1824869O/decontamination-methods-for-3m-n95-respirators-technical-bulletin.pdf Search PubMed.
-
CDC, Decontamination and Reuse of Filtering Facepiece Respirators [Internet], Centers for Disease Control and Prevention, 2020, [cited 2020 Apr 26]. Available from: https://www.cdc.gov/coronavirus/2019-ncov/hcp/ppe-strategy/decontamination-reuse-respirators.html Search PubMed.
-
K. O'Hearn, S. Gertsman, M. Sampson, R. Webster, A. Tsampalieros and R. Ng, et al.Decontaminating N95 masks with Ultraviolet Germicidal Irradiation (UVGI) does not impair mask efficacy and safety: A Systematic Review [Internet], Open Science Framework, 2020, Mar [cited 2020 Apr 6]. Available from: DOI:10.31219/osf.io/29z6u.
- B. Casini, B. Tuvo, M. L. Cristina, A. M. Spagnolo, M. Totaro and A. Baggiani,
et al., Evaluation of an Ultraviolet C (UVC) Light-Emitting Device for Disinfection of High Touch Surfaces in Hospital Critical Areas, Int. J. Environ. Res. Public Health, 2019, 16(19), 3572 CrossRef CAS PubMed.
- B. M. Andersen, H. Bånrud, E. Bøe, O. Bjordal and F. Drangsholt, Comparison of UV C Light and Chemicals for Disinfection of Surfaces in Hospital Isolation Units, Infect. Control Hosp. Epidemiol., 2006, 27(7), 729–734 CrossRef CAS PubMed.
- Z. Qiao, Y. Ye, P. H. Chang, D. Thirunarayanan and K. R. Wigginton, Nucleic Acid Photolysis by UV 254 and the Impact of Virus Encapsidation, Environ. Sci. Technol., 2018, 52(18), 10408–10415 CrossRef CAS PubMed.
- C. Otto, S. Zahn, F. Rost, P. Zahn, D. Jaros and H. Rohm, Physical Methods for Cleaning and Disinfection of Surfaces, Food Eng. Rev., 2011, 3(3), 171–188 CrossRef CAS.
- C. D. Lytle and J.-L. Sagripanti, Predicted Inactivation of Viruses of Relevance to Biodefense by Solar Radiation, J. Virol., 2005, 79(22), 14244–14252 CrossRef CAS PubMed.
-
J. G. B. Derraik, W. A. Anderson, E. A. Connelly and Y. C. Anderson, Rapid evidence summary on SARS-CoV-2 survivorship and disinfection, and a reusable PPE protocol using a double-hit process [Internet], Public and Global Health, 2020, Apr [cited 2020 Apr 24]. Available from: DOI:10.1101/2020.04.02.20051409.
-
C. Carolina Ontiveros and A. William, UV Disinfection of N95 Filtering Facepiece Respirators : A Brief Review UV Disinfection of N95 Filtering Facepiece Respirators : A Brief Review [Internet], 2020, Available from: https://www.researchgate.net/publication/340133321_UV_Disinfection_of_N95_Filtering_Facepiece_Respirators_A_Brief_Review#fullTextFileContent Search PubMed.
- B. K. Heimbuch, W. H. Wallace, K. Kinney, A. E. Lumley, C.-Y. Wu and M.-H. Woo,
et al., A pandemic influenza preparedness study: Use of energetic methods to decontaminate filtering facepiece respirators contaminated with H1N1 aerosols and droplets, Am. J. Infect. Control, 2011, 39(1), e1–e9 CrossRef PubMed.
- D. Mills, D. A. Harnish, C. Lawrence, M. Sandoval-Powers and B. K. Heimbuch, Ultraviolet germicidal irradiation of influenza-contaminated N95 filtering facepiece respirators, Am. J. Infect. Control, 2018, 46(7), e49–e55 CrossRef PubMed.
-
Health Canada, Notice – Important Regulatory Considerations for the Reprocessing of Single Use N95 Respirators (masks) during the COVID-19 Response [Internet], aem. 2020, [cited 2020 Apr 17], Available from: https://www.canada.ca/en/health-canada/services/drugs-health-products/medical-devices/activities/announcements/covid19-notice-reprocessing-n95-respirators.htmlrecommen Search PubMed.
-
U.S. Food and Drug Administration, Recommendations for Sponsors Requesting EUAs for Decontamination and Bioburden Reduction Systems for Surgical Masks and Respirators During the Coronavirus Disease 2019 (COVID-19) Public Health Emergency: Guidance for Industry and Food and Drug Administration Staff [Internet], 2020, Available from: https://www.fda.gov/regulatory-information/search-fda-guidance-documents/recommendations-sponsors-requesting-euas-decontamination-and-bioburden-reduction-systems-face-masks Search PubMed.
- J. R. Bolton and K. G. Linden, Standardization of Methods for Fluence (UV Dose) Determination in Bench-Scale UV Experiments, J. Environ. Eng., 2003, 129(3), 209–215 CrossRef CAS.
- E. M. Fisher and R. E. Shaffer, A method to determine the available UV-C dose for the decontamination of filtering facepiece respirators: UV-C decontamination of respirators, J. Appl. Microbiol., 2011, 110(1), 287–295 CrossRef CAS PubMed.
- B. D. Jett, K. L. Hatter, M. M. Huycke and M. S. Gilmore, Simplified Agar Plate Method for Quantifying Viable Bacteria, BioTechniques, 1997, 23(4), 648–650 CrossRef CAS PubMed.
- G. R. Siragusa, Statistical Validation of the Track-Dilution Plating Method from Ground Beef and Carcass Surface Samples1, J. Rapid Methods Autom. Microbiol., 1999, 7(3), 155–161 CrossRef.
- A. J. Eisfeld, G. Neumann and Y. Kawaoka, Influenza A virus isolation, culture and identification, Nat. Protoc., 2014, 9(11), 2663–2681 CrossRef CAS PubMed.
- E. M. Fisher, A. W. Richardson, S. D. Harpest, K. C. Hofacre and R. E. Shaffer, Reaerosolization of MS2 Bacteriophage from an N95 Filtering Facepiece Respirator by Simulated Coughing, Ann. Occup. Hyg., 2012, 56(3), 315–325 CrossRef PubMed.
- T. P. Coohill and J.-L. Sagripanti, Overview of the Inactivation by 254 nm Ultraviolet Radiation of Bacteria with Particular Relevance to Biodefense, Photochem. Photobiol., 2008, 84(5), 1084–1090 CAS.
- M. E. R. Darnell, K. Subbarao, S. M. Feinstone and D. R. Taylor, Inactivation of the coronavirus that induces severe acute respiratory syndrome, SARS-CoV, J. Virol. Methods, 2004, 121(1), 85–91 CrossRef CAS PubMed.
- B. K. Heimbuch, W. H. Wallace, K. Kinney, A. E. Lumley, C.-Y. Wu and M.-H. Woo,
et al., A pandemic influenza preparedness study: Use of energetic methods to decontaminate filtering facepiece respirators contaminated with H1N1 aerosols and droplets, Am. J. Infect. Control, 2011, 39(1), e1–e9 CrossRef PubMed.
- M. B. Lore, B. K. Heimbuch, T. L. Brown, J. D. Wander and S. H. Hinrichs, Effectiveness of Three Decontamination Treatments against Influenza Virus Applied to Filtering Facepiece Respirators, Ann. Occup. Hyg., 2011, 56(1), 92–101 Search PubMed.
- L. Reverter-Carrión, J. N. Sauceda-Gálvez, I. Codina-Torrella, M. M. Hernández-Herrero, R. Gervilla and A. X. Roig-Sagués, Inactivation study of Bacillus subtilis, Geobacillus stearothermophilus, Alicyclobacillus acidoterrestris and Aspergillus niger spores under Ultra-High Pressure Homogenization, UV-C light and their combination, Innov. Food Sci. Emerg. Technol., 2018, 48, 258–264 CrossRef.
- M. Keyser, I. A. Műller, F. P. Cilliers, W. Nel and P. A. Gouws, Ultraviolet radiation as a non-thermal treatment for the inactivation of microorganisms in fruit juice, Innov. Food Sci. Emerg. Technol., 2008, 9(3), 348–354 CrossRef CAS.
- D. C. Shoults and N. J. Ashbolt, Total staphylococci as performance surrogate for greywater treatment, Environ. Sci. Pollut. Res., 2018, 25(33), 32894–32900 CrossRef CAS PubMed.
- J. P. Wood and A. C. Adrion, Review of Decontamination Techniques for the Inactivation of Bacillus anthracis and Other Spore-Forming Bacteria Associated with Building or Outdoor Materials, Environ. Sci. Technol., 2019, 53(8), 4045–4062 CrossRef CAS PubMed.
- E.-S. Lee, S. Y. Park and S.-D. Ha, Effect of UV-C light on the microbial and sensory quality of seasoned dried seafood, Food Sci. Technol. Int., 2016, 22(3), 213–220 CrossRef PubMed.
-
I. M. Hutten, Handbook of Nonwoven Filter Media, Elsevier, 2007, p. 510 Search PubMed.
-
International Ultraviolet Association, Expert Perspectives on UV as a Tool for N95 Decontamination Webinar [Internet], 2020, May 14 [cited 2020 Jun 1]. Available from: http://www.iuva.org/Expert-Perspectives-on-UV-as-a-Tool-for-N95-Decontamination-Webinar/ Search PubMed.
-
Health Canada, Important Regulatory Considerations for the Reprocessing of Single Use N95 Respirators during the COVID-19 Response: Notice [Internet], aem, 2020, [cited 2020 Jun 21]. Available from: https://www.canada.ca/en/health-canada/services/drugs-health-products/medical-devices/activities/announcements/covid19-notice-reprocessing-n95-respirators.html Search PubMed.
- Y. Tian, L. Rong, W. Nian and Y. He, Review article: gastrointestinal features in COVID-19 and the possibility of faecal transmission, Aliment. Pharmacol. Ther., 2020, 51(9), 843–851 CrossRef CAS PubMed.
- N. Zhu, D. Zhang, W. Wang, X. Li, B. Yang and J. Song,
et al., A Novel Coronavirus from Patients with Pneumonia in China, 2019, N. Engl. J. Med., 2020, 382(8), 727–733 CrossRef CAS PubMed.
-
J.-L. Sagripanti and C. D. Lytle, Estimated Inactivation of Coronaviruses by Solar Radiation With Special Reference to COVID-19. Photochemistry and Photobiology [Internet], [cited 2020, Jun 11], Available from: DOI:10.1111/php.13293.
-
M. S. Favero and W. W. Bond, Chemical Disinfection of Medical and Surgical Materials. In: Disinfection, Sterilization, and Preservation, 5th edn, Lippincott Williams & Wilkins, Philadelphia, PA, 2001, pp. 881–918 Search PubMed.
Footnote |
† Electronic supplementary information (ESI) available. See DOI: 10.1039/d0ew00404a |
|
This journal is © The Royal Society of Chemistry 2020 |
Click here to see how this site uses Cookies. View our privacy policy here.