DOI:
10.1039/C8NH00287H
(Communication)
Nanoscale Horiz., 2019,
4, 495-515
3D quantum theranosomes: a new direction for label-free theranostics†
Received
5th September 2018
, Accepted 19th November 2018
First published on 19th November 2018
Abstract
Quantum-scale materials offer great potential in the field of cancer theranostics. At present, quantum materials are severely limited due to 0D & 1D materials lacking biocompatibility, resulting in coated materials with labelled tags for fluorescence excitation. In addition, the application of magnetic quantum materials has not been reported to date for cancer theranostics. In this current research study, we introduce the concept of applying nickel-based magnetic 3D quantum theranosomes for label-free broadband fluorescence enhancement and cancer therapy. To begin with, we present two (primary and secondary) distinct quantum theranosomes for cancer detection and differentiation (HeLa & MDAMB-231) from mammalian fibroblast cells. The primary theranosomes exhibit a metal enhanced fluorescence (MEF) property through localized surface plasmon resonance to act as cancer detectors, whereas the secondary theranosomes act as cancer differentiators through the fluorescence quenching of HeLa cancer cells. Apart from the above, the synthesized magnetic quantum theranosomes introduced therapeutic functionality wherein the theranosomes mimicked a tumor microenvironment by selectively accelerating the proliferation of mammalian fibroblasts cells while at the same time inducing cancer therapy. These quantum theranosomes were synthesized using femtosecond pulse laser ablation and self-assembled to form an interconnected 3D structure. The 3D architecture and the physicochemical properties of the laser synthesized quantum theranosomes closely resembled a tumor microenvironment. Furthermore, we anticipate that our current recorded findings can shed further light upon these unique magnetic quantum theranosomes as potential contenders towards opening an entirely new direction in the field of cancer theranostics.
Conceptual insights
We introduce a unique concept of applying nickel-based magnetic 3D quantum theranosomes for a label-free broadband fluorescence excitation probe and as a device for cancer therapy to achieve a potential new direction in the field of cancer theranostics. An inherently non-luminescent nickel substrate was transformed to create two distinct 3D quantum theranosomes. They exhibited distinct optical (broadband fluorescence excitation) and magnetic properties, overcoming the major drawbacks (detection and differentiation of cancer cells from mammalian fibroblast cells) of currently available quantum materials, and can thus be successfully exploited for label-free cancer diagnosis. These theranosomes were synthesized via a single-step top-down multi-photon ionization mechanism facilitated by an ultra-short pulsed laser and self-assembled in a 3D structural fashion to mimic the tumor microenvironment. In addition, these theranosomes induced a selective cancer therapeutic property through increased cytoskeletal rearrangement with regards to cancer cells, by constricting their proliferation and migration, but at the same time increased fibroblast cell proliferation. Moreover, these theranosomes increased reactive oxygen species and revealed a distinct cell apoptosis pathway (annexin/PI) with flow cytometry analysis. This new and novel ground-breaking approach will pave the way for next-generation cancer theranostics, which interests a broad spectrum of audiences specializing in the fields of cell–material interaction and cancer treatment.
|
Introduction
Theranostics is the integration of a dual function system that offers both cancer diagnostic imaging and concurrent therapy merged into a single operating platform. Cancer theranostics have now gained momentous importance in recent years by offering targeted delivery through conjugates facilitating both chemotherapy and bioimaging through novel strategies in oncological research.1 Recent cancer theranostics require non-invasive imaging techniques to track the progress of the therapeutic cells. Current optical imaging (fluorescence, bioluminescence or photo acoustics) is one such technique, offering remarkably high sensitivity amongst a variety of cell tracking techniques. It is worth mentioning at this stage that all the above-mentioned techniques require the use of multiple specific labels or tags to accomplish the desired imaging using nanoscale materials.2 Nevertheless, there is no reported work on label-free fluorescence bioimaging being adopted or already implemented for cancer theranostics.
Currently, to achieve cancer therapy and imaging, a variety of functional nanomaterials have been developed. For instance, nickel, iron and cobalt based nanoparticles have been investigated primarily for their drug loading and transport capacity.3–5 They are equipped to transport contrast agents and dyes for image-based diagnosis, and cancer treatment is achieved through photodynamic, thermal or pH variation.6,7 Thus, the nanoparticles are used as a platform for drug delivery and induce cancer therapy through external targeting. For instance, iron oxide nanoparticles coated with the chemotherapeutic agent mitoxantrone targeted VX-2 squamous cell carcinoma.8 Similarly, superparamagnetic iron oxide nanoparticles with a size range from 50 to 200 nm coated with cetuximab agent were used to target squamous head and neck cancers.9 The iron oxide nanoparticles helped to achieve magnetic targeting. However, the nonpreferential heating and the need for external magnetic or electric field to excite the cytotoxic and apoptotic effect on cancer cells pose significant side effects by interacting with healthy cells.
Primarily, to achieve label-free fluorescence bioimaging, one worthwhile approach is the move towards quantum-scale materials, thereby opening a new direction in cancer theranostics. Quantum dot (QD) particles with fluorescence excitation are a highly desirable feature for cancer theranostics. These quantum-scale (QS) materials have been functionalized externally to be used as fluorescence probes in biolabeling and bioimaging applications due to their unique high quantum yield, molar excitation co-efficient level, and optical and electronic properties.10–12 These unique properties exhibited by the quantum-scale materials are due to the quantum confinement effect observed in metals and semiconductor materials that are of 2–8 nm in size. These materials in turn share dimensional similarities to proteins and nucleic acids in the desired new biological environment.13,14 Nevertheless, conventional fluorescence cell imaging utilizing fluorophores suffers major drawbacks due to non-specific fluorophore accumulation and short lifetime (ns to μs) with a progressive photobleaching process and they possess very limited contrast features that hinder their use in theranostic applications.15 Hence, there is now an inherent need for an apt diagnostic method to overcome all the above-mentioned limitations offered by these fluorescent molecules for cancer theranostics.
In general, all quantum-scale materials exhibit many attractive characteristic properties, such as size-dependent tunable fluorescence, high brightness, single wavelength excitation and large surface to volume ratios. In addition to the above, they also resist photobleaching and chemical degradation compared with available conventional dyes.16 But the use of these QDs for theranostics has been severely restricted due to their innate cytotoxicity, particle size dependency for excitation, single wavelength excitation and the need for bioconjugation labels for in vitro application to induce fluorescence excitation.17,18 Thus, there is an overwhelming need for quantum-scale materials which address all the aforementioned drawbacks of QDs to achieve a potential label-free cancer theranostic agent.
Some successful attempts were reported addressing some of the above-mentioned drawbacks using coated QDs and noble-metal doped QDs for fluorescence bioimaging applications.19–21 These are often nanoscale polymeric coatings or tripeptide coatings over QDs to help in improving biocompatibility and thereby overcoming toxicity. Similarly, QDs are limited to specific wavelength excitation due to particle sizes and thus different particle sizes synthesized using a variety of synthesis techniques are combined to achieve the desired broadband fluorescence excitation. Furthermore, these QDs are limited to 0D and 1D materials and so do not possess the physiochemical characteristics to mimic a tumor microenvironment for effective deployment in cancer theranostic applications, thereby severely limiting their use. Therefore, to the best of our knowledge, there exists no reported work on the use of 3D label-free magnetic quantum probes with broadband fluorescence excitation for cancer theranostics.
Moreover, to satisfy the use of cancer fluorescence bioimaging probes for cancer theranostic applications, there is a need for a viable transport mechanism. This can essentially be fulfilled using magnetic materials to achieve the desired site-specific reaction at the targeted site through a variety of external targeting approaches: for instance Magnetic Resonance Imaging (MRI) and thermal imaging.22,23 Both these methods, however, are not only time consuming but the Enhanced Permeability and Retention (EPR) effect has severely limited their use in fluorescence bioimaging applications.24 Some successful attempts were demonstrated along the same lines using magnetic QDs as a viable alternative to fluorescent dyes for bioimaging applications. These were achieved using magnetic materials like nickel, gadolinium and iron by a variety of techniques, such as magnetic doping, functionalized ligands and antibody coatings for labelled multimodal imaging.5,25,26 While the magnetic materials addressed the targeting aspect, the issue of biocompatibility of these materials has not yet been fully evaluated for bioimaging applications. For instance, Sudhasree et al. and Abudayyak et al. highlighted that nickel nanoparticles synthesized through chemical synthesis were more cytotoxic compared with nanoparticles synthesized through a physical route.27,28 In-addition, to be successfully deployed for cancer theranostics, these magnetic probes must also necessarily induce cancer therapy. Nevertheless, to this date, there has been no reported work published regarding the use of magnetic quantum materials for label-free cancer theranostics.
In this research study, we report the development of nickel-based magnetic quantum theranosomes for cancer theranostics. Two (primary and secondary) theranosomes were synthesized in this research to achieve label-free broadband fluorescence excitation and for cancer therapy. The primary (quantum-Ni) theranosomes acted as a cancer detector by exhibiting a fluorescence intensity only upon interacting with cancer (MDAMB-231 and HeLa) cells compared with NIH3T3 fibroblast cells. This fluorescence enhancement is achieved through localized surface plasmon resonance, giving rise to metal enhanced fluorescence excitation. The secondary (quantum-NiO) theranosomes acted as a cancer differentiator, distinguishing between the interacting cancer cells by selectively demonstrating increased fluorescence intensity in MDAMB-231 cancer cells compared with HeLa cancer cells. The secondary theranosomes, chiefly composed of metal oxide, induced energy-dependent fluorescence enhancement through theranosome localization, thereby inducing selective fluorescence enhancement. This selective fluorescence excitation (ON) and quenching (OFF) effect brought about by the synthesized theranosomes automatically empowered them to be the right choice to act as label-free fluorescence bioimaging probes. In addition, both the theranosomes also induced selective cancer therapy while simultaneously encouraging fibroblast cell proliferation, as seen from cell viability and flow cytometry analysis (ROS and annexin/PI) conducted exclusively for validation purposes. These theranosomes were synthesized using a simple top-down multiphoton ionization mechanism and self-assembling them in a 3D fashion. The ability to fine tune the ionization mechanism was realized in creating the two distinct theranosomes primary (quantum-Ni) and secondary (quantum-NiO) with varying material chemistry and properties. The 3D arrangement of the quantum theranosomes combined with tunable physiochemical properties offered itself as a potential candidate to create label-free fluorescence imaging and cancer cell therapy without any adverse side-effects to mammalian fibroblast cells, thereby demonstrating biocompatibility by mimicking a tumor microenvironment. The results accomplished and data collated in our research study here pave the way for the application of these magnetic quantum theranosomes to act and serve as ideal candidates for the next generation of label-free cancer theranostics.
Materials and methods
Materials
A nickel 200 sheet purchased from Onlinemetals.com (USA) with 99% nickel content was cut into (3 × 10 × 15) mm (D × W × L) to be used to conduct the experiments. SiC grit sandpaper (200 to 1200) was used to induce gradual mechanical polishing over the nickel surface, which was later ultrasonically cleaned using 100% ethanol and acetone for 15 minute duration each to remove all debris particles embedded during the surface polishing process. All the polished samples were then washed again with de-ionized (DI) water and finally air dried to conduct further experiments.
Laser ionization to synthesize theranosomes
A tunable Yb diode pumped fiber laser from the Impulse series (Clark – MXR) with a central wavelength of 1030 nm with an average power of 16 watts was utilized for the fabrication of the desired quantum theranosomes. This ultra-short pulsed laser system utilizes varying laser pulse repetition rates between 200 KHz and 25 MHz to synthesize the desired quantum theranosomes. The air-dried nickel substrates were then mounted on an X–Y–Z precision stage which was normal to the laser beam direction. A laser beam with a 10 μm spot diameter was then focused onto the nickel substrate. To create an even distribution of quantum theranosomes on the nickel substrate, a piezo-electric raster scanning system with EzCAD software was used. For the ionization of quantum theranosomes, a laser pulse repetition rate of 4 MHz to 26 MHz along with a laser pulse width of 214 fs was used under a gaseous medium of N2 species for the formation of the ion-plume. The gases were injected directly into the interaction zone through several individual nozzles, continuously maintaining a flow rate of 1 bar pressure which evenly surrounded the total core within the interaction zone.
Synthesis of theranosomes by multiphoton ionization mechanism of solid nickel substrate
The synthesis of magnetic quantum theranosomes over the nickel substrate by ultra-short pulsed laser interaction is depicted in a schematic in Fig. 1. A computer-controlled scanner allowed for the precise two-dimensional (2D) movement of an ultra-short laser beam over the nickel substrate in the X–Y direction to synthesize 3D quantum theranosomes. This 3D quantum theranosome generation by ultra-short laser pulses in the presence of an inert nitrogen atmospheric medium is a complex gas dynamic and non-linear process, involving a very specific and set sequence of events: surface energy absorption of laser pulses, plasma plume ignition & ionization, rapid condensation and ejection of species to the atmosphere.
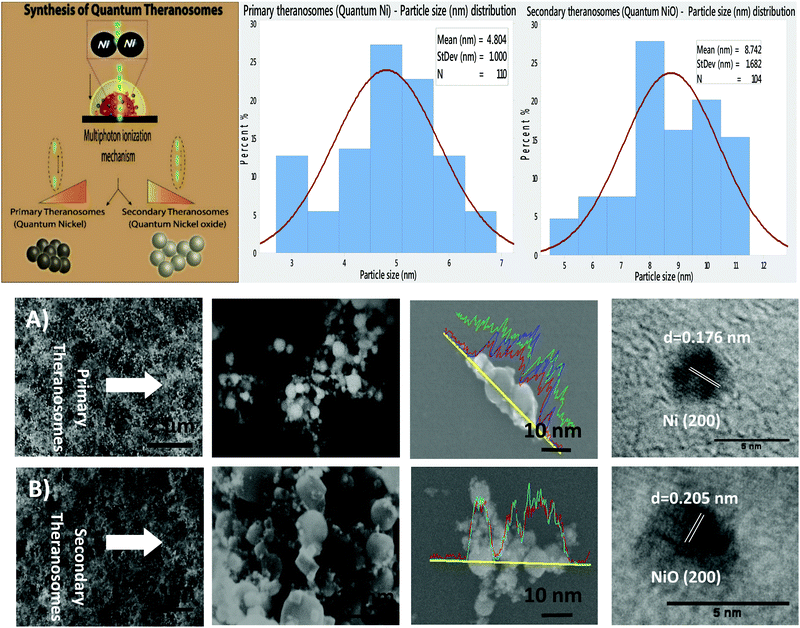 |
| Fig. 1 Characterization of primary (quantum-Ni) and secondary (quantum-NiO) theranosomes. The FESEM shows the self-assembled 3D quantum structures. The HR-SEM insets show individual particle morphologies, which are composed of primarily spherical and cubic particles. HR-TEM displays lattice spacing ‘d’ indicating the presence of nickel and nickel oxide along with particle size distribution. | |
Primary theranosome synthesis
To synthesize primary theranosomes, a 26 MHz laser pulse repetition rate is employed. This yields an energy of 1.64 joules per unit square centimeter which is focused onto the nickel substrate, resulting in the formation of ionized nickel species within the interaction plume, which later cools under an ambient atmosphere to form quantum-scale primary theranosomes. During this process, only a small focal volume within the ionization plume absorbs the incident energy and transforms the material, thereby minimizing thermal stress and damage. Control over the state of this expanding ionization plume is based on a combination of various parameters, such as laser pulse energy (μJ), pulse interaction time (fs) and the peak power (MW) of incoming laser pulses. Here, the energy deposited by ultrashort laser pulses far exceeds the inter-atomic binding energy level and the nickel atom under excitation becomes totally free from its bonds only to interact further with the incoming ultra-short laser pulses. These super heated ions cool down to form primary quantum theransomes composed entirely of nickel. This is verified by XRD, and HRTEM material analysis.
Secondary theranosome synthesis
To synthesize secondary theranosomes, a 4 MHz laser pulse rate is deployed where the energy per unit square centrimeter is dramatically increased to 5.14 joules. This initiates a kinetic reaction within the expanding ionization plume which remains in a gaseous-ionic state encompassing both non-interacting atoms ejected from the material surface to interact further with oncoming laser pulses. Since the laser pulse interaction time is an order of magnitude lower than the material heat diffusion time, this induces a superheated ion formation reaction creating ionised species which transform the material from one crystalline structure to an another. This very swift transformation, from the initial cold solidified state to an ionic state of the substrate when under excitation, causes a time-dependent ionization and material recombination process. The final ejection of the ionised speciecs from the plasma plume forces the superheated ions to cool down as they are propelled further away from the interaction surface only to recombine with the oxygen medium present in the ambient atmosphere, thereby condensing to form 3D self-assembled secondary quantum theranosome particles. From material analysis, the secondary theranosomes are composed entirely of nickel oxide. The growth rate of every individual particle varies with both lattice plane orientation and temperature gradient, thereby enabling effective control over both the particle morphology and particle size.
Theranosome morphological and physicochemical characterization
The synthesized quantum theranosome specimens were imaged using a high-resolution scanning electron microscope (HRSEM). An HRSEM/EDX attachment from Oxford Instruments was used to analyze the elemental composition of the synthesized theranosomes. In order to quantify the particle size distribution, every theranosome particle in an observed image was counted individually. This process was then repeated three times to demonstrate repeatability. The image analysis software ImageJ downloaded from the National Institute of Health (USA) was used. The graphs were plotted using Minitab 18. To quantitatively identify the oxide composition of the synthesized theranosomes X-ray diffraction (Phillips powder XRD) was employed with Cu K-α radiation (λ = 1.54054 Å) at 40 kV and 40 mA with a 2θ scanning speed of 0.010° min−1. Subsequently Reitveld analysis was also carried out on the acquired spectrum. Thereafter, a Bruker Senterra Dispersive Raman Microscope fitted with a 532 nm laser system was used to analyze the theranosomes. A 50× magnification objective was used to focus the laser onto the sample surface with an appropriate laser irradiation power of 5 mW. A standard collection time of 10 s with three iterations was employed and all the necessary parameters were chosen by an earlier trial and error method conducted to reduce the spectrum noise. All the spectrums collected were baseline corrected and normalized to laser power.
Optical characterization
A colloidal suspension of the synthesized theranosomes was made using a Phosphate Buffer Solution (PBS) and a Hitachi UV-3100 UV-vis-NIR spectrophotometer was used to analyse the UV-vis-NIR absorption and reflection spectrums of the synthesized primary and secondary theranosomes.
In vitro cell culture
HeLa cells, human cervical cancer cell line, were obtained from ATCC (American Type Culture Collection, ATCC No. CCl-2) and were cultured in DMEM-F12 medium with phenol red containing heat-inactivated fetal bovine serum and 1% penicillin–streptomycin antibiotics at 37 °C in a 5% CO2 atmosphere. NIH3T3 fibroblast cells and MDAMB-231 were also obtained from ATCC were cultured in DMEM medium containing 10% fetal bovine serum with 1% penicillin–streptomycin antibiotics at 37 °C in 5% CO2. The theranosome samples were washed with both alcohol and DI water and thereafter kept under UV light for a duration of 15 minutes. The substrates were then placed in separate Petri-dishes with HeLa, MDAMB-231 and NIH3T3 cells seeded individually to attain a density of 105 cells per mL, totalling 4 mL quantity by volume per dish. The Petri dishes were then placed in an incubator for 24 and 48 hours duration.
SEM sample fixation
After the set incubation period, the samples were fixed for SEM imaging in 2% glutaraldehyde over a 0.1 M sodium cacodylate buffer of pH 7.3 for an hour. Later, the samples were immersed in 0.1 M cacodylate buffer with 0.2 M sucrose for about 20 minutes. The samples were then dehydrated in an increasing concentration level of alcohol for 20 minutes duration each time. The samples were then critical point dried and gold sputtered to achieve improved imaging. SEM imaging was conducted using a Hitachi SU1500 instrument at 10 kV with a magnification range between 500 and 15
000 times. Three separate images were chosen for each of the conditions to conduct further quantitative analysis for measuring cell analysis using ImageJ software.
Label-free fluorescence bioimaging
The samples were prepared in a very similar way to the in vitro cell culture process and after the set incubation period of 24 hours, the samples were washed once using 1% PBS and thereafter fixed using 4% methanol-free paraformaldehyde. The samples were then air-dried and made ready for bioimaging using an epi-fluorescent Nikon E-400 microscope with a 3-filter setting (DAPI, FITC and Texas Red). The data were recovered with a DS-5M-UI color digital camera (Nikon, Canada) and collated.
Differential fluorescence staining of cells
The samples were seeded with appropriate cells and incubated for 24 hours and 48 hours, similar to the SEM sample investigation. After the set incubation period, all the samples were fixed in 4% methanol-free paraformaldehyde followed by an incubation in 1% skimmed milk to prevent non-specific binding. To stain the actin cytoskeleton, the samples were incubated with Alexa fluor 488 (Life Technologies) followed by DAPI (4′,6′-diamidino-2-phenylindole, Life Technologies) to stain the nucleus. The samples were then air-dried and later imaged using an epi-fluorescent Nikon E-400 microscope. The data were recovered with a DS-5M-UI color digital camera (Nikon, Canada) and collated.
Assessment of necrosis and apoptosis using annexin V–PI staining
Apoptosis in HeLa, MDAMB-231 and NIH3T3 cells was measured by using the annexin V–propidium iodide (PI) and apoptosis detection kit (BD Biosciences, San Diego, USA). All the cells were exposed to primary (quantum-Ni) and secondary (quantum-NiO) theranosomes in a 6-well plate for durations of 24 and 48 hours. After the said exposure, cells were first trypsinized and later centrifuged at 1000 rpm to form pellets which were all washed with PBS once and again re-suspended in 1 mL of binding buffer, then incubated with 0.2 mL of annexin V–FITC for 10 min, followed by staining with 0.2 mL of PI. Thereafter, the samples were analyzed with a Fortessa X-20 flow cytometer from BD Bioscience (St. Michael's Hospital), and at least a minimum of 10
000 cells were counted for each sample. The cell population of interest was gated based on the forward and side-scatter properties. Both the vertical and horizontal lines were designed based on the auto-fluorescence of untreated control cells. The different labeling patterns adopted in the annexin V/PI analysis were to identify the different cell populations wherein both the FITC negative and the PI negative were designated as viable cells; FITC positive and PI negative cells were earmarked as early apoptotic cells; FITC positive and PI positive cells were marked as late apoptotic cells; and FITC negative and PI positive cells as necrotic cells. The complete data analysis was performed using BD FACS Diva software.
Reactive oxygen species (ROS) determination using flow cytometry and fluorescence microscopy
Cells were all treated with QS theranosomes for a duration of 24 and 48 hours. The cells were first harvested by a trypsinization process and thereafter centrifuged at 1000 rpm to form pellets. The pellets were resuspended in a DCFDA working solution for a duration of 30 min at 37 °C. After washing with PBS, the pellets were once again resuspended in PBS to be immediately analyzed using flow cytometry (Fortessa X-20, BD Bioscience). A minimum of 10
000 events were recorded and the data was processed using BD FACS Diva software. Similarly, cells were treated with primary and secondary theranosomes for 24 and 48 hours and cell intracellular ROS generation was determined by a fluorescence microscopy probe DCFH-DA. The green fluorescence intensity of DCF was found to be enhanced in the theranosome treated cells compared with the control cells.
Transmission electron microscopy – bio TEM
Theranosome-treated cells (MDAMB-231, HeLa and NIH3T3) which had been incubated for 24 hours were trypsinized to detach the cells from the Petri-dish. They were then centrifuged to form pellets (1 × 106 cells per pellet). During this process, some theranosome particles get removed from the cells and then settle. The obtained pellet was fixed in 2% glutaraldehyde in 0.1 M sodium cacodylate buffer and stored at 4 °C until sectioning. To prepare the TEM grids, cells were rinsed in buffer and fixed in 1% osmium tetroxide in buffer, dehydrated in a graded ethanol series followed by propylene oxide, and embedded in EMBed812 resin. Cells were then stained with uranyl acetate and lead citrate. 100 nm thick sections were then cut using an RMC MT6000 ultramicrotome and viewed in an FEI Tecnai 20 TEM microscope. TEM preparation and imaging were performed at the Nanoscale Biomedical Imaging Facility of the Hospital for Sick Children Research Institute, Toronto, Canada.
Cell viability assessment
Cell viability was assessed by the MTT assay (Sigma Aldrich) protocol. MTT is a colorimetric assay which depicts cells by measuring the intensity of the purple color. The NIH3T3 fibroblast and MDAMB-231 cancer cells were seeded at a concentration of 1 × 104 cells per well in 100 μL of culture medium in 96-well plates. Cell culture continued for 24 hours and then cell viability was determined following the instructions provided by the manufacturer. The amount of colored reaction obtained was proportional to the number of viable cells. The mean absorbance of non-treated cells was used as a reference value to calculate 100% cell viability.
Statistics
All the experiments were carried out in triplicate and all data points collected were standard mean with ± standard error unless otherwise mentioned. The error bars included indicate standard deviation. On the whole, an overall 95% confidence interval for the mean was achieved.
Results and discussion
Morphological and material chemistry investigation of theranosomes
The surface morphology of the self-amassed 3D quantum scale theranosomes synthesized under nitrogen atmospheric conditions indicated in Fig. 1 was observed using FE-SEM and HR-SEM. A web consisting of both coalesced cubic and spherical-like structures was noticed. These coalesced particles were further aggregated to form an intertwined web-like structure which was arranged over multiple layers to form the 3D quantum theranosomes. The morphology of the synthesized theranosomes under a nitrogen-filled atmosphere was analyzed and was observed to have a wider size distribution of particles, as can be seen from the graphs in Fig. 1. Based on the above observation, the synthesized particles within the observed structure was then further characterized into primary (quantum-Ni) and secondary (quantum-NiO) theranosomes. The energy dispersive X-ray analysis using HRSEM on individual theranosome particles detected elemental nickel, oxygen and carbon. This is evident from the line EDX images presented in Fig. 1, where the primary theranosome particles had a higher ratio of nickel to oxygen. The oxygen detected is from the surface oxidation of particles from the theranosomes during imaging and the carbon sensed is from the TEM grid. The line EDX data on the secondary theranosome had an increased ratio of oxygen to nickel, supporting theranosome formation. To study the morphological particle distribution range of these synthesized theranosomes, an HR-TEM analysis was carried out. The distribution graphs from Fig. 1 show a particle size histogram performed over several observed images. The derived results fit into a single log-normal distribution, which describes the most prominent features of the histogram. The mean distribution sizes of the primary and secondary theranosome particles were 4.7 nm and 8.8 nm respectively. The narrow particle size distribution attained in this study is due to the precise control over the multiphoton photon ionization process achieved through a combination of laser pulse energy and laser pulse-to-pulse separation time. The surface characterization of these synthesized theranosomes was performed using Raman, XRD and XPS to determine the change in the chemical composition and the different oxidation states of primary (quantum-Ni) and secondary (quantum-NiO) theranosomes.
For all the above conditions, we observed that the Raman bands from Ni and NiO were in the spectral region above 400 cm−1. The Raman peaks noted in Fig. 2(A) and (B) were primarily vibration modes due to the first and second order Raman scattering caused by phonons in Ni and NiO, whereas the band above 1200 cm−1 originates due to the scattering by two magnons in Ni and NiO. In general, magnons are observed in magnetic materials due to electron spin wave excitations. The inelastic scattering observed with magnons can also occur in magnetic materials due to spin–orbit coupling.29 The phonon and magnon modes were analyzed quantitatively by deconvoluting the Raman spectra using Gaussian functions. The intense Raman band observed in Fig. 2(A) at ∼509 cm−1 was assigned to the first-order one phonon (1P) LO mode. The Raman band observed near 500 cm−1 could also be of magnetic origin. In the present work, the Raman bands positioned at ∼509 cm−1 could also be directly related to quantum-scale particles (nanocrystallites) as particle size does influence magnetic behavior.30 Also, there was a total absence of the first-order TO mode under all conditions. We then assigned the 1075 cm−1 band to the second-order LO phonon modes.
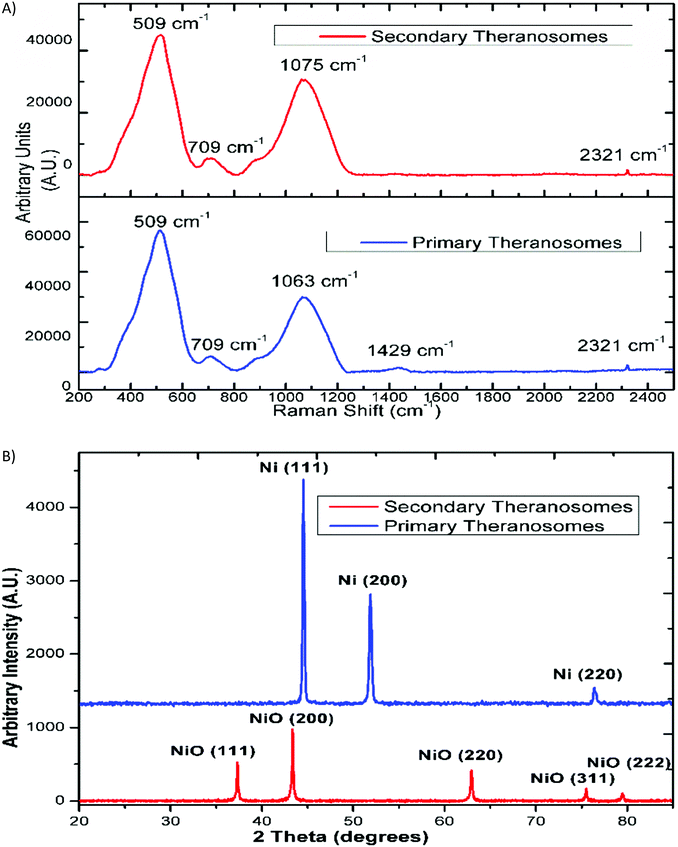 |
| Fig. 2 (A) Raman spectra of primary (quantum-Ni) and secondary (quantum-NiO) theranosomes. (B) X-Ray diffraction and Reitveld analysis of the synthesized theranosomes to qualitatively identify the weight percentage of observed phases. | |
The relative intensity of the LO phonon mode was found to be enhanced with primary theranosomes (quantum-Ni) compared with secondary theranosomes (quantum-NiO), relating to a change in particle size variation, as cited in the literature.30 In addition, the decreasing peak size for the secondary theranosomes indicates a high level of nickel vacancy in the synthesized theranosomes. Furthermore, the second-order (2TO) transverse optical mode of NiO was observed for all theranosomes at ∼709 cm−1, as can be seen from Fig. 2(A). Moreover, the two magnon modes (2M) at ∼1400 cm−1 intensity were observed only for primary theranosomes (quantum-Ni). From the literature and previous studies, it was presumed that the 2M vibrations seen in nickel-based nanomaterials are primarily due to an antiferromagnetic super-exchange interaction in nickel ions and that in the linear atomic chain Ni2+–O2−–Ni2+.31,32 The near-complete absence of 2M excitations seen in Fig. 2(A) for secondary theranosomes (quantum-NiO) was consistent with the enhancement in LO mode conditions. This directly refers to the even bond breakage of Ni2+–O2−–Ni2+. Furthermore, the 3 MO mode at ∼2300 cm−1 was present in all conditions. In addition, the observed first-order Raman band was derived from symmetry-breaking imperfections and defects (like Ni vacancies or disorder in crystal lattices); hence it can be suggested that its FWHM can be used as an indicator for the presence of these defects in the NiO samples.
X-Ray diffraction and Reitveld analysis
Additionally, X-ray diffraction (XRD) analysis of the synthesized quantum-scale theranosomes, as in Fig. 2(B), shows significant line broadening, which is characteristic of nickel and nickel oxide. The reflection peaks for nickel were indexed to the face-centered cubic (fcc) structure of nickel. Three diffraction peaks at 2θ = 44.5, 51.9, 76.5 degrees correspond to the Miller index (111), (200) and (220) facets of primary (quantum-Ni) theranosomes. The peaks at 2θ = 37.28, 43.28, 62.88, 75.28, 79.48 degrees correspond to the diffraction from (111), (200), (220), (311), (222) facets of secondary (quantum-NiO) theranosomes.33,34 The above results were also confirmed with HR-TEM lattice images of primary and secondary theranosomes, as can be seen from the insets in Fig. 1. Reitveld fitting was conducted on the acquired spectrum to derive a qualitative weight percentage approximation for each identified phase in the synthesized theranosomes. Furthermore, XPS was deployed as a qualitative technique to establish and resolve the chemical and surface atomic composition of the synthesized theranosomes.
X-ray photoelectron spectroscopy (XPS) analysis of synthesized theranosomes
The survey scan results presented in Fig. 3(A) and (B) exhibit the main core-level peaks for O1s and Ni2p centered at binding energies (BEs) of 532, and 855 eV, respectively. The binding energy (BE) scale was corrected with reference to the spurious C1s peak (282.9 eV) attributed to specimen charging and surface contamination effects. Detailed energy analysis of the Ni2p (Fig. 3(A)) spectrum shows its characteristic spin–orbit splitting of the nickel ion into 2p3/2 and 2p1/2 peaks (at 856.38 and 874.08 eV), respectively, with an energy separation of 17.7 eV. The corresponding satellite peaks observed at higher energies of 862 eV and 880 eV relate to the 2p3/2 and 2p1/2 energy region. These satellite peaks at higher energies than the “2p” principal peaks in this energy region have been ascribed to a charge transfer multielectron transition.35 These features corroborate the suggestion of the surface oxidation of the primary (quantum-Ni) theranosomes, even though their low-intensity profile is indicative of a smaller extent of the oxidation phase that it had undergone. In addition, the intensity of the nickel peaks noted was significantly higher for primary (quantum-Ni) theranosomes compared with secondary (quantum-NiO) theranosomes. Furthermore, the individual scans showed the presence of trace oxygen content and a strong O1s core-level spectrum signal from the primary (quantum-Ni) theransomes.36 However, only a strong O1s core peak was apparent from the secondary (quantum-NiO) theranosomes as observed (in Fig. 3(A) and (B)).
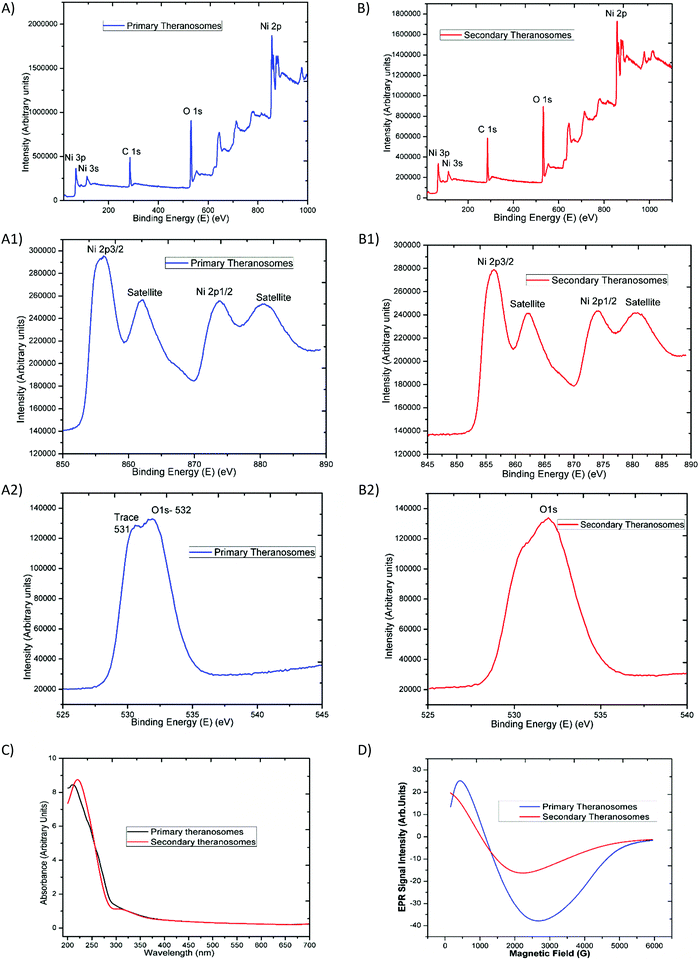 |
| Fig. 3 XPS survey and individual spectrums exhibiting the strong presence of nickel and oxygen for (A) primary theranosomes and (B) secondary theranosomes; (C) UV-Vis spectra of theranosomes exhibiting increased photon absorption; (D) room temperature electron paramagnetic resonance curves for different theranosomes. | |
Optical (UV-Vis) and magnetic (resonance properties using EPR) characterization
The synthesized quantum theranosomes ranging from 200 to 700 nm were first investigated with ultraviolet-visible absorption at room temperature, and exhibited a significant high photon absorption, as shown in Fig. 3(C). It was evident from the synthesized theranosomes that the absorption level gradually decreases with an increase in wavelength from 250 to 700 nm. The absorption band was observed at 212 nm for primary (quantum-Ni) theranosomes and at 220 nm for secondary (quantum-NiO) theranosomes. The bulk nickel nanomaterial absorption band was centered around 230 nm, as noted and cited from the available literature.37 The observed red shift in the absorption bands in our present investigation was due to the quantum size confinement effect with (quantum-Ni) primary theranosomes particles shifting more than the (quantum-NiO) secondary theranosomes, thereby confirming the presence of nickel and nickel oxide particles.38,39 In addition, metallic nickel has no band gap and the observed UV-absorption spectrum of primary theranosomes was due to the oxidation reaction of nickel with PBS.40
Furthermore, to investigate the magnetic properties, Electron Paramagnetic Resonance (EPR) spectroscopy was used to study the synthesized material states with unpaired electrons. It was observed that the primary (quantum-Ni) and secondary (quantum-NiO) theranosomes in Fig. 3(D) exhibit a narrow resonant peak at low magnetic field. The relative peak positions of the absorption lines depend on the particle size. I.e., the peak position shifts to higher fields, indicating that the material is in a ferromagnetic state. It can now be well acknowledged that the EPR signal of NiO disappears because of the presence of strong exchange fields. On the other hand, a particle size of less than 100 nm can also exhibit paramagnetism at room temperature due to the presence of Ni+ ions already present in NiO. Ulmane et al. and Gandhi et al. undertook an extensive study on the size-dependent properties of nickel-based nanomaterials. Based on their observations, the decreasing and complete absence of the 2M mode from the Raman spectrum with increasing LO mode was correlated to the surface disorders of NiO.41,42 In our present study, the formation of an EPR signal in comparison with the magnetic properties could be attributed to the existence of uncompensated spin on the surface of the particles, which provides a sufficient supportable explanation for the presence of these field-dependent magnetic properties.
Quantum theranosome fluorescence enhancement intensity using flow cytometry and fluorescence microscopy assessment
To assess their potential as a bioimaging probe, we examined the quenching and excitation effect of the synthesized quantum theranosomes, which were made into colloidal suspensions using phosphate buffer solution (PBS) and were analyzed using flow cytometry by acquisition of the side-scattering (SSC) and forward-scattering (FSC) signal from Fig. 4. A notable change in SSC and FSC signals was revealed in the suspension compared with a control and the theranosomes showcased increased activity in all the visible channels in both forward- and side-scattering channels. This is backed up by the photoluminescence (PL) spectrum of the synthesized theranosomes presented in Fig. 4(D) and (E). This confirmed the broadband emission in the visible wavelength.39 An increase in fluorescence intensity is observed when the excitation is increased from 400 to 700 nm. In addition to the above, a red shift in excitation was also observed. Moreover, the irradiation of quantum theranosomes in the visible range, as depicted in the fluorescence images, demonstrates higher excitation of these quantum theranosomes when viewed from the bright field, and the single (DAPI, FITC and Texas Red) channel images in Fig. 4(A) and (B) and the ESI,† Image S1.
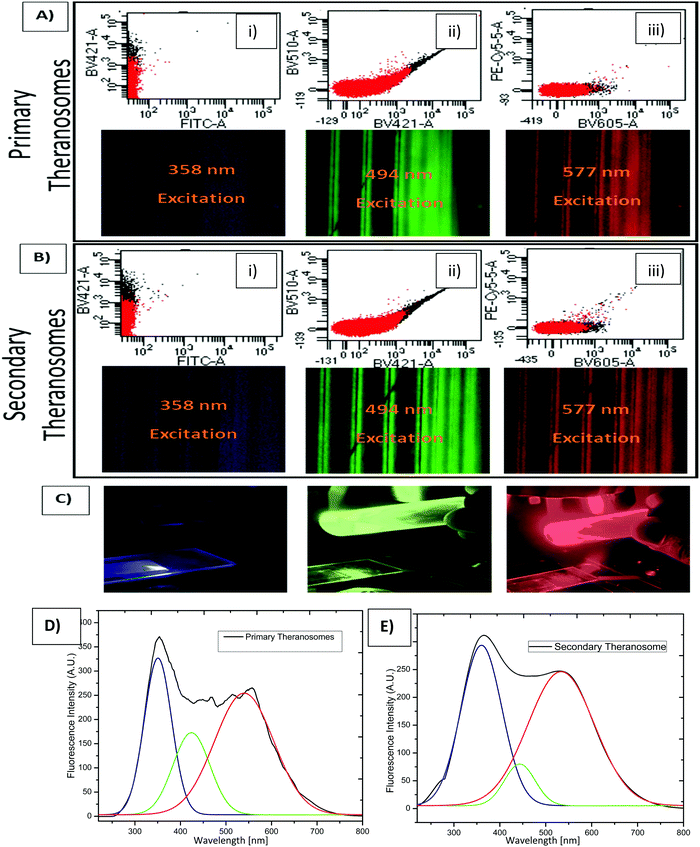 |
| Fig. 4 Emission of theranosomes at excitation wavelengths of multiple channels at 358, 494, 577 nm exhibits the fluorescence characteristics of the theranosomes using flow cytometry and fluorescence microscopy. A comparison of fluorescence intensity at (A) primary theranosomes (i), (ii) and (iii) represent flow cytometry measurement at their respective excitation channels and (B) secondary theranosomes (i), (ii) and (iii) represent flow cytometry measurement at their respective excitation channels. (C) A representative comparison shows the different fluorescence intensity of the synthesized colloidal theranosome particles under corresponding fluorescence excitation channels in fluorescence microscopy. Photoluminescence (PL) synchronous spectrum of (D) primary and (E) secondary theranosomes showed strong PL with respect to the excitation wavelength. The emission was at a broadband visible wavelength which demonstrates the fluorescence excitation of the theranosomes. | |
To evaluate the fluorescence property and showcase the nanomaterial interaction in a biological environment, multiple techniques have been developed, such as confocal Raman mapping, atomic force microscopy, TEM and fluorescence microscopy. TEM being extremely high resolution (∼1 nm) is valuable for providing visualization of both the nanoparticle location in cellular compartments and nanoparticle aggregation.43 Herein, TEM is used to both showcase theranosome aggregation inside the cells and to demonstrate its fluorescence bioimaging property. The traditional TEM bright-field mode is used, allowing nanometer-scale details and visualization of theranosomes in biological milieux. Since the theranosomes are inorganic in nature with high crystallinity and mass, they appear dark in the bright-field mode. Thus, a dark-field mode is also applied to showcase the theranosome location and activity. The synthesized theranosomes, being fluorescent in nature, appear as bright white spots when visualized in dark-field mode.
In vitro fluorescence bioimaging property of the theranosomes
For cellular diagnosis, the fabricated quantum theranosomes were introduced to NIH3T3 fibroblasts, (MDAMB-231) breast cancer cells and cervical cancer cells (HeLa) as model cell lines for examining cell viability. The cell viability (from Fig. 5(A), (B) and 6(A), (B) and their corresponding insets, and ESI,† Fig. S2) indicated that the quantum theranosomes are all biocompatible for conducting the analysis of living cells, thereby confirming their use as a bioimaging probe. To establish that the theranosomes are viable broadband fluorescence bioimaging probes, the synthesized probes must become localized in the cell and be able to distinguish normal cells from cancerous cells. The cytotoxicity of the synthesized theranosomes was assessed in a standard cell culture condition by an MTT assay. The results obtained are presented in ESI,† Fig. S2(B), highlighting the increased viability of fibroblast cells in both primary and secondary theranosomes compared with breast cancerous cells. The results infer that the theranosomes are inducing a cytotoxic response only to cancerous cells. Yallapu et al. suggested increased intracellular uptake or accumulation of drugs and drug-coated nanoparticles in cancer cells is often used as an indicator for a higher therapeutic index.44 Thus, we investigated the uptake of both primary and secondary theranosomes using TEM evaluation. Bio-TEM observations on ultrathin sections of HeLa, MDAMB-231 and NIH3T3 cells after treatment with theranosomes for 24 hours is visualized in Fig. 5(A) and (B). This was used to explore the process of cellular uptake for cancer therapy and the use of theranosomes for fluorescence bioimaging. In general, molecules enter cells through passive diffusion, but nanomaterials enter cells predominantly through an endocytosis mechanism. A significant amount of research had already been conducted to determine and establish how nanoscale materials enter cancerous and fibroblast cells.45–48
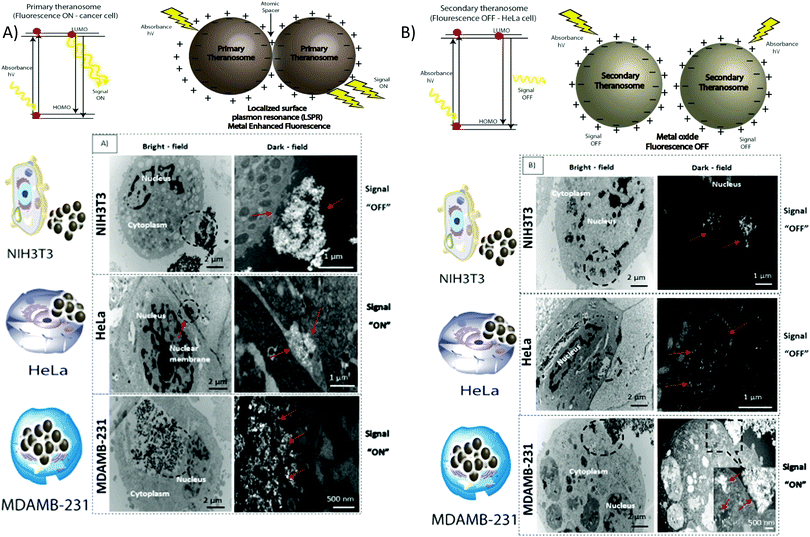 |
| Fig. 5 (A) Representative TEM image of primary theranosomes in HeLa, MDAMB-231 and NI3T3 fibroblast cells. The theranosomes are located within the cellular membrane in both cancer cells when compared with fibroblast cells. The corresponding dark-field images on the right show the location and localization of the theranosomes. (B) Representative TEM image of secondary theranosomes in HeLa, MDAMB-231 and NIH3T3 fibroblast cells. The theranosomes are located within the cellular membrane in both cancer cells when compared with fibroblast cells. The corresponding dark-field images on the right show the location and localization of the theranosomes. | |
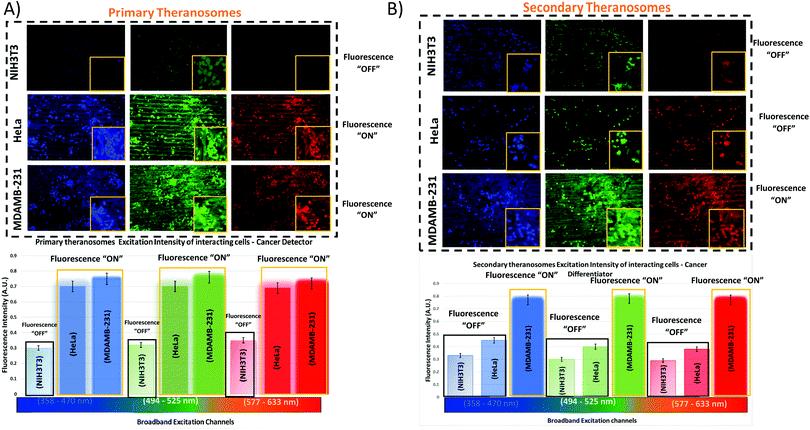 |
| Fig. 6 (A) Primary quantum theranosomes acting as cancer detectors, demonstrating increased fluorescence intensity of cancer (MDAMB-231, HeLa) cells compared with fibroblast (NIH3T3) cells. Standard scales of 10 μm and 50 μm for the insets are deployed. (B) Secondary quantum theranosomes acting as cancer differentiators, demonstrating the increased fluorescence intensity of cancer (MDAMB-231) cells compared with both fibroblast (NIH3T3) and HeLa cancer cells. Standard scales of 10 μm and 50 μm for the insets are deployed. | |
The theranosomes were found internalized near and in almost all cell components of both types of cancerous cells. Representative images of (primary and secondary) theranosomes interacting with both (HeLa and MDAMB-231) cancerous and NIH3T3 fibroblast cells are showcased in Fig. 5, wherein the primary theranosomes are visible in the (HeLa) nuclear membrane and in close proximity to the (MDAMB-231) nucleus. A magnified dark-field TEM view of the theranosome uptake is also presented in Fig. 5(A) which is brightly illuminated with white spots represented by red arrows. Interestingly, a significant portion of the primary theranosomes were located as clusters in the cellular structures, a phenomenon which is completely absent in fibroblast cells. But the secondary theranosomes showed several clustered aggregations irrespective of cell phenotype from the TEM observations. Several research studies have reported that both single and dispersed nanoparticles in general enter cells through endocytosis and tend to aggregate at the cell membrane, which results in receptor clustering.45,49 Numerous pieces of research have also demonstrated a wide array of nanoparticle uptake mechanisms and pathways in cancerous and fibroblast cells to establish their therapeutic efficiency and also drug transport into cancer cells, especially the clathrin-mediated endocytosis (CME) mechanism at the nano-bio interface level.50,51 Since CME plays a vital role in the cellular process to transport nutrients, specific studies have focused on their interactions with cellular membranes involving a unique set of interactions. For instance, Chithrani et al. demonstrated a detailed study detailing the clathrin-dependent endocytosis mechanism of plasmonic gold nanoscale materials in breast cancer cells.52 Similarly, Yallapu et al. showcased higher nanoparticle uptake of iron oxide by MDAMB-231.53 The endocytosis process dynamically modulates several cancer cell functions and is thereby responsible for their proliferation and migration. Thus, it has been inferred that endocytosis in cancer cells is not only unregulated but also makes the process quite different based on cell phenotype when compared with normal cells.50 Similarly, in this work it was found that the fluorescence intensity of NIH3T3 cells compared with cancerous cells was significantly weaker on both primary (quantum-Ni) and secondary (quantum-NiO) theranosomes compared with that of the corresponding native nickel control surface. In-addition, the results also indicated that the fluorescent intensity of both theranosomes was at its lowest on NIH3T3 fibroblast cells under all excitation channels compared with the native control and (HeLa and MDAMB-231) cancer cell lines. This selective fluorescence excitement of theranosomes establishes the viability of using theranosomes for fluorescence bioimaging. Furthermore, the primary (quantum-Ni) theranosome probe showed increased fluorescence intensity when interacting with both types (MDAMB-231 and HeLa) of cancer cell compared with corresponding fibroblasts and the native control surface. Thus, the primary theranosome probe acted as a turn-ON fluorescence probe, increasing fluorescence intensity when made to interact with cancer cells. This is validated by the data presented in Fig. 5(A) and 6(A). The selective fluorescence excitation exhibited by the synthesized primary theranosomes can be explained using the energy transfer reaction observed in plasmonic metallic materials such as gold and silver. These plasmonic materials due to increased accumulation and localization near the cellular membrane introduce localized Surface Plasmon Resonance (LSPR) which in turn brings out Metal Enhanced Fluorescence (MEF) excitation. This observed reaction exhibits increased fluorescence excitation upon interacting with all cancerous cells compared with NIH3T3 fibroblast cells.54 This excitation reaction thus induced in cancer cells essentially acts as a light-up bioprobe, reaching increased fluorescence intensity through theranosome aggregation in cancer cells.55,56
However, the secondary theranosomes exhibit high fluorescence intensity only on MDAMB-231 cells compared with HeLa cells. This selective enhanced fluorescence intensity excitation exhibited by the secondary theranosome probe on MDAMB-231 cells is roughly twice that of its interaction with HeLa cells. The enhanced fluorescence excitation of MDAMB-231 cancer cells by the secondary theranosome probe with simultaneous fluorescence quenching on HeLa cells establishes the above-mentioned secondary theranosome probe as a viable cell-specific fluorescence exciter. The secondary theranosomes, being composed entirely of NiO, acts as typical metal oxide particles. The presence of oxygen within the secondary theranosomes supresses the spontaneous energy transfer reaction which is observed in the primary theranosome through MEF, thus limiting its fluorescence excitation property and thereby requiring more energy per unit area to excite the fluorescence property.57 Furthermore, in general, when metal ions interact with cancer cells the primary uptake pathway is through CME.58 For instance, Chaves et al. highlighted that in magnetic iron oxide nanomaterial, breast cancer cells have a higher particle uptake compared with normal cells of similiar origin.59 Thus, a similar conclusion can be inferred from the experimental data presented in Fig. 5 and 6, where both primary and secondary theranosomes exhibited higher particle uptake of MDAMB-231 cancer cells. When comparing the uptake between HeLa and MDAMB-231 cancer cells in secondary theranosomes, the HeLa cancer cells presented less particle uptake compared with MDAMB-231 cancer cells. This observed change is also due to the phenotye and origin of the HeLa cancer cells. Since each cancer cell phenotype is different, the uptake mechanism associated with it also varies significantly.60 In-addition, HeLa cancer cells follow calveloe-mediated endocytosis and macropinocytosis rather than caltherin-mediated endocytosis.61 Thus the observed mechanism essentially acted as a switch to turn ON and OFF the fluorescence property of the primary and secondary theranosomes. This unique and specific property of the quantum nickel oxide to excite only interacting MDAMB-231 cancer cells and not HeLa cells was an interesting aspect here, as this specific property opens up the use of synthesized secondary theranosomes (quantum-NiO) as an active cancer cell-specific diagnostic agent. This effect was congruent to the higher targeting efficiency exhibited by the secondary theranosomes on MDAMB-231 cancer cells in inducing cell apoptosis compared with HeLa cancer cells, as can be seen from the insets of Fig. 7. Furthermore, the TEM images presented in Fig. 6(B) also showcase less secondary theranosome accumulation but on the other hand more localization compared with primary theranosomes.
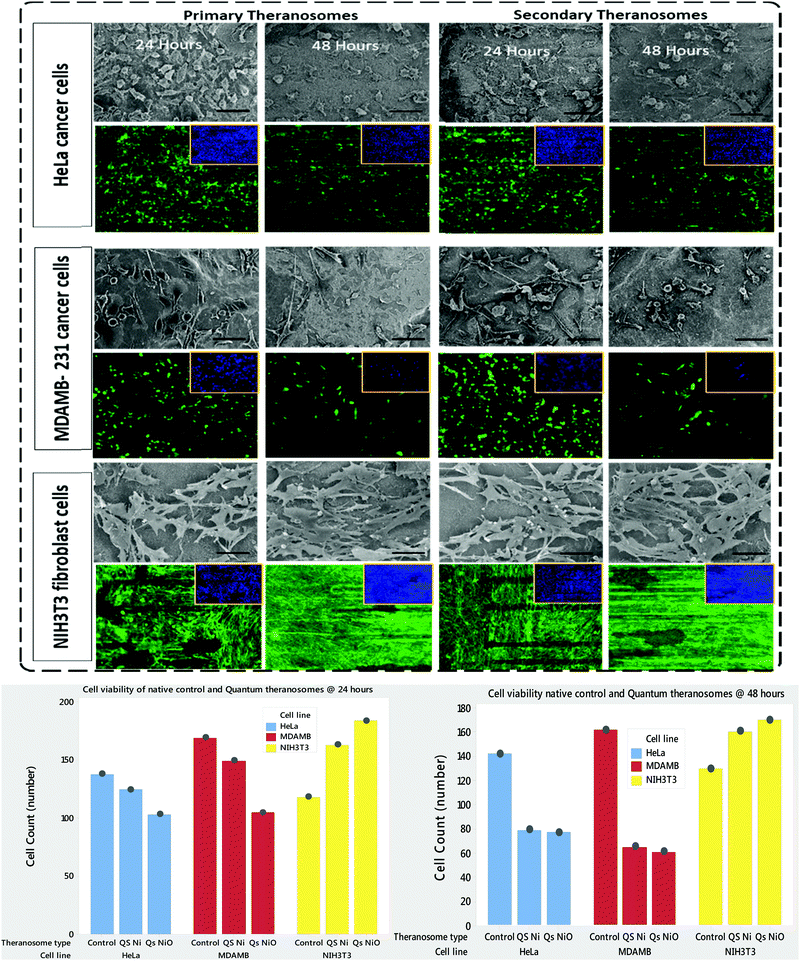 |
| Fig. 7 Cellular proliferation of NIH3T3 fibroblast, HeLa and MDAM-231 cancer cells on primary and secondary theranosomes. Representative SEM and fluorescence images showing the proliferation of three different cell lines, metastatic MDA-MB-231, and HeLa cancer cells, as well as the noncancerous NIH3T3 fibroblast cells. | |
In vitro therapeutic efficacy of theranosomes
NIH3T3 fibroblasts, MDAMB-231 and HeLa cancer cells are cultured both on native nickel and synthesized quantum theranosomes to investigate and evaluate their adhesion and proliferation response. The SEM results presented in the insets of Fig. 7 compare the HeLa, MDAMB-231 and NIH3T3 fibroblast cell population number and morphology response on native and synthesized quantum theranosomes. All the cells attached and spread over well and evenly within the 24 hours duration without the need for any special treatment with ECM molecules prior to cell incubation, making the synthesized quantum theranosomes act like and mimic ECM-like behavior irrespective of the cell type. The NIH3T3, MDAMB-231 and HeLa cells proliferating on native nickel were observed to be smaller in size and were randomly distributed throughout the substrate. However, the number, morphology and organization of cancerous (HeLa & MDAMB-231) and NIH3T3 cell adhesions on quantum theranosomes were different from those of the cells that were attached to the native nickel substrates. In addition, the interacting cells systematically proliferated and grew in an organized manner with stretched filopodia and lamellipodia, as can be seen from the insets of Fig. 8. The cells adhering to the quantum theranosomes were larger in size than the cells proliferating upon the native nickel substrate. The NIH3T3 fibroblast cells present on quantum theranosomes were closely self-organized and almost covered in a one monolayer thickness, making it hard to distinguish one cell from another.
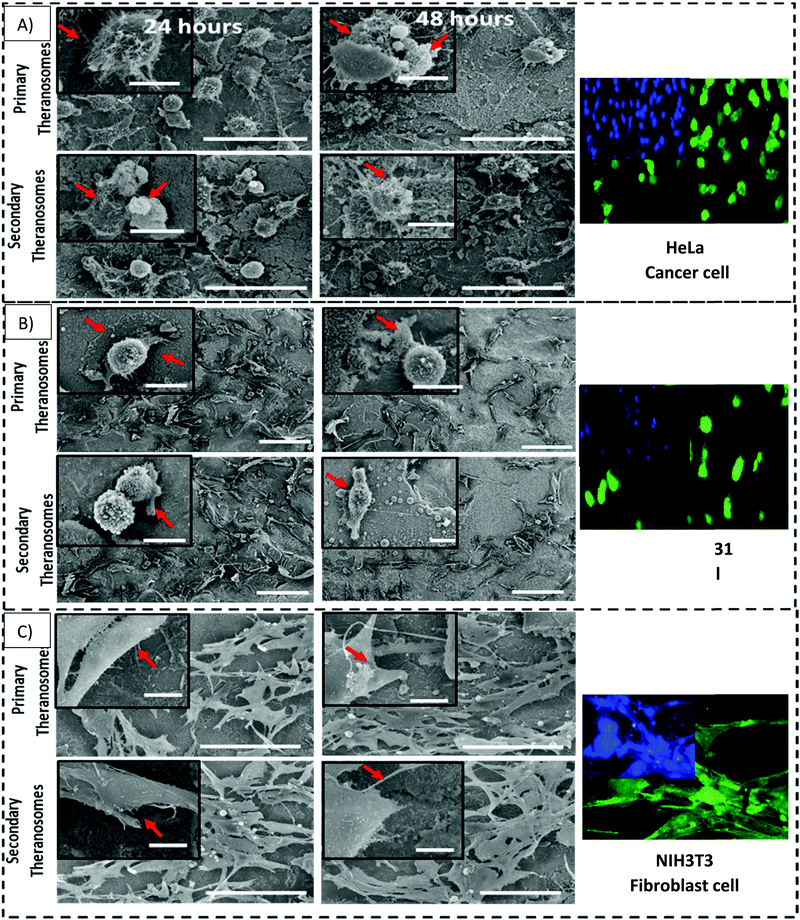 |
| Fig. 8 Influence of primary and secondary theranosomes on the adhesion (24 hours) and proliferation phases (48 hours). (A) Comparison between the quantum theranosomes over HeLa cell adhesion. (B) Comparison between the quantum theranosomes over MDAMB cell adhesion. Adhesion (C) of quantum theranosomes over NIH3T3 cell adhesion. Uniform scale bars of 10 and 3 μm were followed. The red indicators are the filopodial presence and its interaction on theranosomes. | |
A pronounced self-organized cell coverage was observed during the first 24 hour incubation on both primary and secondary theranosomes. This trend continued even with a prolonged incubation time of 48 hours on both the native nickel and theranosomes, as can be seen in the insets of Fig. 7 and 8. The quantum theranosomes, however, altered fibroblast cell behaviour after 48 hours duration with more evidence of elongated cell morphology present on quantum theranosomes, suggesting cell migration had taken place. Nonetheless, the in vitro response of MDAMB-231 and HeLa cells on the quantum theranosomes revealed remarkable cell-governing properties.
First, we investigated the influence of MDAMB-231 and HeLa cells on both the 3D quantum theranosomes, which played a key role in controlling cancer cell behaviour compared with fibroblast cells, as seen from Fig. 7. They demonstrated an increased fibroblast cell adhesion compared with cancer cells. The statistical results of the same cells after 24 hour incubation presented in the insets show that the total cancer cell count was reduced significantly on both quantum theranosomes compared with native nickel control samples from 24 to 48 hours. A complete reversal of cell attachment was noted with respect to particle size, wherein the quantum web acted as a decoy by turning off its proliferative behaviour during the 48 hour incubation period. We can certainly establish a grade of cancer killing efficiency in an increasing order based on the observed results, with secondary and primary theranosomes having low and high degrees of killing efficiency. The fluorescence microscopy analysis also suggests the same trend, as can be seen from the insets in Fig. 7 and 8. On the other hand, the fibroblast cell adhesiveness on quantum theranosomes does not seem to alter even with an increased incubation time of 48 hours. This unique and specific property of quantum nickel oxide to excite only interaction is observed from the results presented herein that NIH3T3 cells are less susceptible to theranosomes compared to MDAMB-231 and HeLa cells. Earlier studies on a tumor microenvironment demonstrated its vital role in regulating cell adhesion, sensing and signalling aspects. Emerging studies in a 3D tumor environment have now pointed out that the current need for incorporating ECM proteins and specific biomolecules is to alter the cell morphologies, adhesion, proliferation and migration properties.62 The 3D porous structures due to its heterogeneous nature have shown attributable traits in cellular adhesion and migration compared with its one and two-dimensional counterparts. Unlike conventional anti-cancer agents that straight away attack cancer cells right from the beginning, these quantum theranosomes are acting more as targeted agents. They presented favourable cues to cancer cells initially, thereby promoting adhesion and proliferation. But apoptosis was then induced after a prolonged incubation time of 48 hours through autophagy. Moreover, this apoptosis was induced solely by the particles present in the theranosome architecture, making it a quantum medicine rather than a mere drug carrier. Distinct biochemical and physical changes involving cell cytoplasm, nucleus and plasma membrane are often associated with apoptotic cell death.63 In a tumor microenvironment, during an early stage of apoptosis, cells predominantly cluster to become spherical, thereby losing contact with their surrounding cells and then subsequently starting to shrink. The filopodia of interacting HeLa, MDAMB-231 and NIH3T3 cells observed via SEM can be seen from the insets of Fig. 8(A)–(C) were employed to study the adhesion and proliferation properties of cells upon synthesized theranosomes. Filopodial development on native nickel was quite random in nature. In contrast, cells interacting on quantum theranosomes showed a drastic change in the number of filopodia during the first 24 hour incubation period. The cells that proliferated had on an average fewer filopodia with an altered morphology compared with the basic native nickel substrate. It is a well-known aspect that cells need to spread so as to stratify the various phases of cell growth. Thus, filopodia that adhere to substrates typically originated from random locations within the cell body and at a certain distance above the surface. These filopodia thus come into contact with the underlying substrate under a broad range of angles, as can be seen from the insets of Fig. 8(A). It could also be seen that, apart from the filopodia interacting with the theranosomes structure, so were the retraction fibers of the cells, as can be seen from the insets of Fig. 8(A). The presence of filopodia and retraction fibers at the end points demonstrate the presence of a high degree of incidence of cellular and synthesized quantum theranosomes interaction having already taken place.
Intracellular reactive oxygen species (ROS) assessment of quantum theranosomes
Reactive oxygen species (ROS) play a critical role in the early stages of cell apoptosis. Mitochondria were considered both as a source and also as a target of ROS exhibited by these cells. In general, when excess ROS was generated, it persuaded mitochondria membrane potential (MMP) depolarization to release cytochrome c, which stimulates caspase activation. The ROS generation can also induce DNA damage, which then promotes apoptosis.64 Wang et al. demonstrated that nickel refining fumes induced DNA damage and apoptosis on NIH3T3 fibroblast cells through oxidative stress.65 In addition, Pan et al. showed nickel sub sulfide (Ni3S2) induced ROS-mediated apoptosis in human bronchial epithelial BEAS-2B cells through the p38 signalling pathway.66 Furthermore, Ahamed et al. suggested that nickel nanoparticles induce oxidative damage, which decreases glutathione (GSH) and induces ROS and lipid peroxidation (LPO) in human lung epithelial A549 cells. Hence excess generation of ROS will eventually result in oxidative stress that mediates apoptosis.67 Although several previous studies had shown induction of ROS induced cell death in various cancer and fibroblast cells by different nanoparticles, they were all limited to colloidal nanoparticle suspensions whose dimensions were greater than 20 nm. Hence, we were quite interested to investigate the effect of 3D quantum theranosomes synthesized here, focussed more towards ROS generation in MDAMB-231 cancer cells and NIH3T3 fibroblast cells. For this study, MDAMB-231 and NIH3T3 cells were loaded with the ROS measuring probe 2′,7′-dichlorodihydro fluorescein diacetate (H2DCFDA) and studied using flow cytometry. As shown in Fig. 9, both primary and secondary quantum theranosomes induced ROS generation in a time-dependent manner, as indicated by a noted increase in fluorescence intensity. The ROS generated was at its peak after 48 hours of exposure to both quantum theranosomes in MDAMB-231 compared with untreated MDAMB-231 controls, as can be seen from ESI,† Fig. S3. The primary (quantum-Ni) theranosomes observed an increased ROS generation (39.69%) after 24 hours incubation period, compared with secondary (quantum-NiO) theranosomes (30.5%). However, with an increased incubation period of 48 hours the ROS intensity increased significantly for primary theranosomes (45.68%) compared with secondary theranosomes (74.11%) depicting a cell-selective physiochemical dependent ROS mediated apoptosis upon MDAMB-231 cancer cells. Thus, a substantial increase in MDAMB-231 cell ROS was observed in a comparison between the primary and secondary theranosomes. Concurrently, the same quantum theranosomes induced a very negligible ROS generation in NIH3T3 cells. A representative figure showing the difference in the fluorescence intensity is highlighted in Fig. 9, with normal proliferating cells showing a decreased level of fluorescence compared with an apoptotic cell.
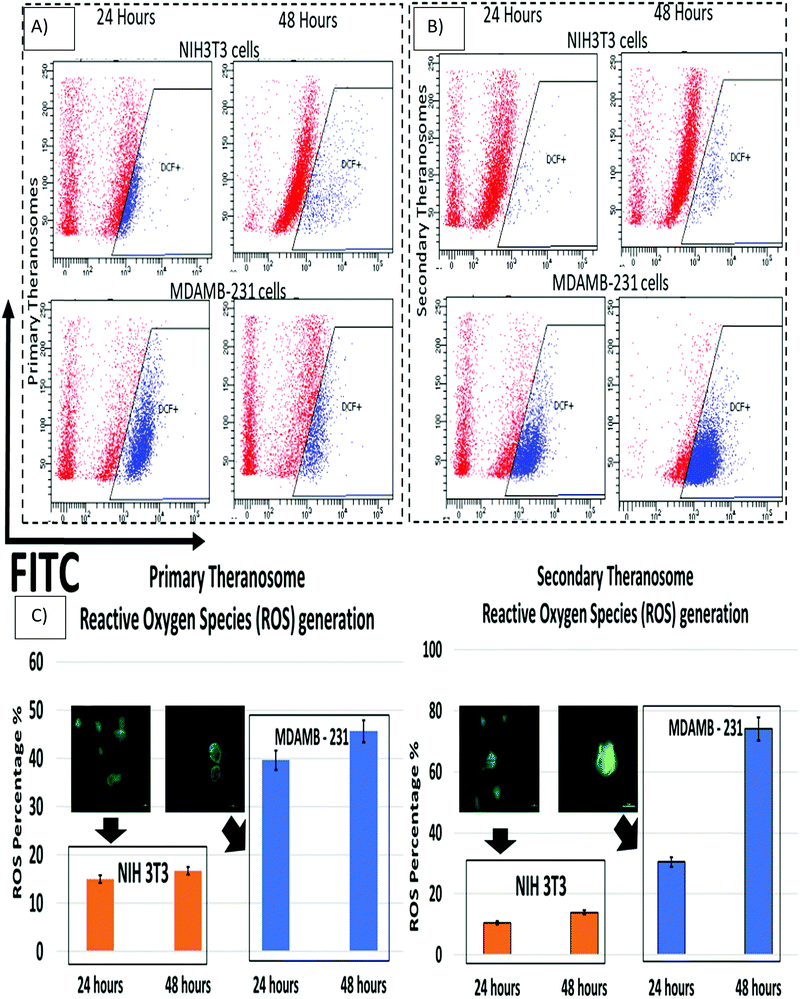 |
| Fig. 9 ROS generation – DCFH-DA assay. The blue dot plots indicate the cells under ROS through increased fluorescence intensity with respect to the control. (A) NIH3T3 and MDAMB-231 cells treated with primary (B) and secondary theranosomes. (C) Graph showing the percentage of cells with DCF fluorescence in blue. The data represents mean ± SD of three independent experiments. | |
Cell death induction by 3D quantum theranosomes and assessment of necrosis and apoptosis
Since the production of ROS is a major mediator for the activation of apoptosis signalling pathways, the influence of 3D quantum theranosomes on cancer and fibroblast cell apoptosis was evaluated by the annexin V-FITC/PI conjugate staining assay at 24 and 48 hours incubation periods. The results obtained are presented in Fig. 10 and in ESI,† Fig. S3, along with respective statistical analyses. Quadrant 3 highlighted in the graphs in Fig. 10 represents the percentage of viable cells present during the analysis. Both the quantum theranosomes presented an increased percentage of NIH3T3 fibroblast cells compared with MDAMB-231 cancer cells, irrespective of the incubation period. The percentage of cells in quadrant 4 indicated an early-stage apoptotic cell death, suggesting that the cells present in that quadrant are beyond viability. The primary theranosomes presented a significant percentage increase in early-stage cancer cell apoptosis compared with fibroblast cells, with an increase in incubation time from 24 to 48 hours. In contrast, the secondary theranosomes presented an increase in percentage of early apoptosis in cancer cells only during the first 24 hour time period. Additionally, the percentage of cells in quadrant 2 highlighted the percentage of end-stage apoptotic cell events in both NIH3T3 and MDAMB-231 cells. Both the theranosomes recorded a lower level, less than 5% events (24 and 48 hours) of end-stage fibroblast cell apoptosis, but offered an increased end-stage apoptosis in MDAMB-231 cells. Furthermore, the percentage of cells stained positive for proprium iodide (PI) was observed from quadrant 1, indicating a loss of membrane integrity which was a clear indication of necrotic cell death. The quantum theranosomes irrespective of the incubation time induced cell-selective apoptosis and necrosis to MDAMB cancer cells alone. But the NIH3T3 cells showed some signs of necrosis on primary quantum theranosomes (6%) during the first 24 hours only, but after an increased incubation time showed a reversal of trend (<1%) wherein no signs of either apoptotic or necrotic cells were observed. The secondary theranosome results for NIH3T3 cells also followed an identical trend over the 24 and 48 hour time periods. This reversal of the necrosis trend from 24 to 48 hours is primarily due to the initial reaction and exposure of primary and secondary theranosome particles interacting with NIH3T3 cells. In addition, as for the NIH3T3, the significantly low percentage (6%) of NIH3T3 cell necrosis observed during the first 24 hours can also be attributed to the stray theranosome particles during the flow cytometry measurement. But the secondary theranosomes showcased almost ∼600% percent change in MDAMB-231 cancer cell necrosis between the 24 and 48 hour incubation periods. The above results very clearly demonstrated that the cell-selective apoptotic behaviour exhibited was induced only by these specifically engineered and fabricated 3D quantum theranosomes.
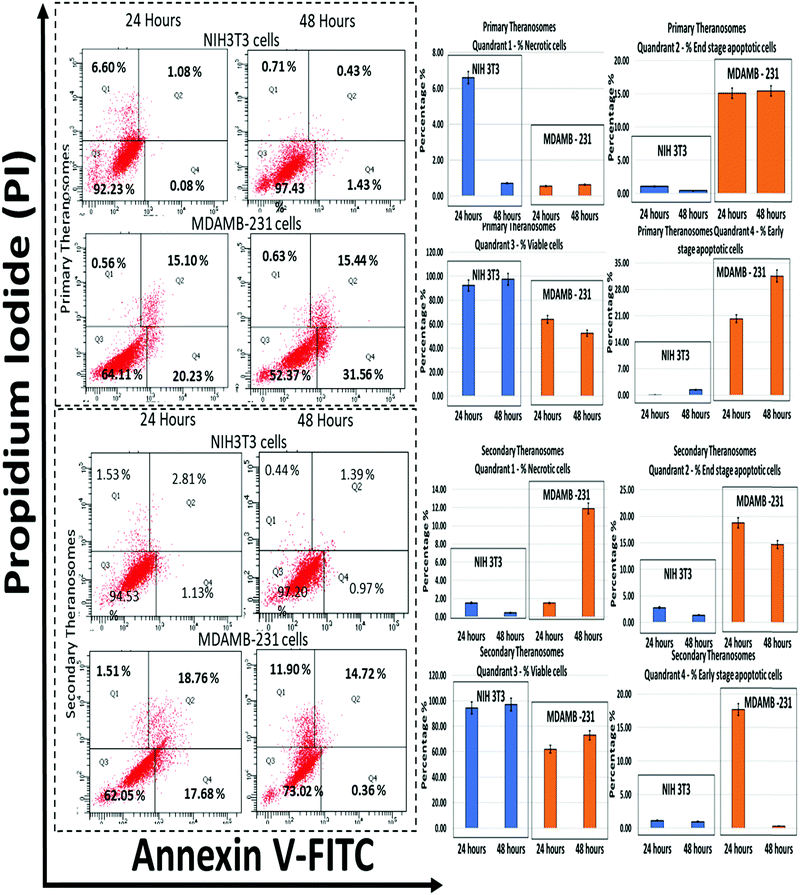 |
| Fig. 10 Cell apoptosis determined by annexin V-fluorescein isothiocyanate/propidium iodide staining. The results were determined after incubation with primary and secondary theranosomes in MDAMB cancer cells and NIH3T3 fibroblast cells for 24 and 48 hours (n = 3). A standard minimum of 10 000 events were recorded for each plot. | |
Conclusions
Theranosomes hold great promise for the future in the field of cancer treatment, as they can detect (imaging) and diagnose cancer and thereafter induce cancer apoptosis (theranostics) in a single-step process. The magnetic quantum theranosomes presented herein propose a new direction for cancer theranostics using label-free broadband fluorescence excitation and cancer therapeutics. The synthesized (primary and secondary) theranosomes provided both a magnetic and an optical response, allowing them to impart both label-free fluorescent imaging and cancer therapeutic functions through quantum confinement. The proposed primary (quantum-Ni) theranosome identified cancer (HeLa and MDAMB-231) cells from NIH3T3 fibroblast cells by enhancing the cancer cell fluorescence excitation, which in a way is a diagnostic process. The secondary (quantum-NiO) theranosome distinguished the MDAMB-231 cells from HeLa cells through its specific fluorescence excitation, thereby acting as a screening agent by selectively enhancing only the fluorescence signals of MDAMB-231 cancer cells through fluorescence imaging. In addition, the in vitro study conducted confirmed and demonstrated the biocompatibility of the proposed quantum theranosomes, wherein the 3D architecture empowered mammalian cells to exhibit selective adhesion and proliferation but later selectively induced (HeLa and MDAMB-231) cancer cell apoptosis, mimicking a tumor microenvironment. Although favourable biocompatibility is attributed to quantum theranosomes, the intracellular oxidative stress derived through reactive oxygen species and the apoptotic pathway assessment clearly demonstrated the innate therapeutic functionality exhibited by these synthesized theranosomes compared with those of the fibroblast cells. The theranosomes presented in this research are synthesized using a novel one-step multiphoton ionization mechanism arranged in a 3D fashion. The evidence recorded and presented in this research demonstrates the suitability of magnetic quantum theranosomes as a potential candidate for future cancer theranostics. This simplified approach will pave the way for next-generation cancer theranostics, which should be of interest to a broad spectrum of researchers in the field of theranostics.
Author contributions
Sivaprasad C. V. carried out all sample preparation, experimentation, material characterization and drafted the manuscript. Krishnan V. and Bo T. supported its design and co-ordination.
Funding
This research was funded by the Natural Science and Engineering Research Council of Canada (NSERC Discovery Grant 126042, 119087).
Conflicts of interest
There are no conflicts of interest to declare.
References
- Y. Wang, Y. Wang, G. Chen, Y. Li, W. Xu and S. Gong, ACS Appl. Mater. Interfaces, 2017, 9, 30297–30305 CrossRef CAS.
- A. B. Chinen, C. M. Guan, J. R. Ferrer, S. N. Barnaby, T. J. Merkel and C. A. Mirkin, Chem. Rev., 2015, 115, 10530–10574 CrossRef CAS PubMed.
- X. Wang, W. Cao, L. Qin, T. Lin, W. Chen, S. Lin, J. Yao, X. Zhao, M. Zhou, C. Hang and H. Wei, Theranostics, 2017, 7, 2277–2286 CrossRef CAS PubMed.
- L. Wu, A. Mendoza-Garcia, Q. Li and S. Sun, Chem. Rev., 2016, 116, 10473–10512 CrossRef CAS.
- A. Pramanik, S. Jones, F. Pedraza, A. Vangara, C. Sweet, M. S. Williams, V. Ruppa-Kasani, S. E. Risher, D. Sardar and P. C. Ray, ACS Omega, 2017, 2, 554–562 CrossRef CAS PubMed.
- T. D. Nguyen, Colloids Surf., B, 2013, 103, 326–344 CrossRef CAS PubMed.
- Y. Jun, J. Seo and J. Cheon, Acc. Chem. Res., 2008, 41, 179–189 CrossRef CAS.
- C. Alexiou, R. Tietze, E. Schreiber, R. Jurgons, H. Richter, L. Trahms, H. Rahn, S. Odenbach and S. Lyer, J. Magn. Magn. Mater., 2011, 323, 1404–1407 CrossRef CAS.
- Z. A. Vegerhof, E. A. Barnoy, M. Motiei, D. Malka, Y. Dannan, Z. Zalevsky and R. Popovtzer, Materials, 2016, 9, 943 CrossRef PubMed.
- K. D. Wegner and N. Hildebrandt, Chem. Soc. Rev., 2015, 44, 4792–4834 RSC.
- D. N. Basov, R. D. Averitt and D. Hsieh, Nat. Mater., 2017, 16, 1077–1088 CrossRef CAS PubMed.
- J. M. Pérez-Donoso, J. P. Monrás, D. Bravo, A. Aguirre, A. F. Quest, I. O. Osorio-Román, R. F. Aroca, T. G. Chasteen and C. C. Vásquez, PLoS One, 2012, 7, e30741 CrossRef PubMed.
- A. P. Alivisatos, Science, 1996, 271, 933–937 CrossRef CAS.
- I. L. Medintz, H. T. Uyeda, E. R. Goldman and H. Mattoussi, Nat. Mater., 2005, 4, 435–446 CrossRef CAS PubMed.
- K. Huang, H. Ma, J. Liu, S. Huo, A. Kumar, T. Wei, X. Zhang, S. Jin, Y. Gan, P. C. Wang, S. He, X. Zhang and X. J. Liang, ACS Nano, 2012, 6, 4483–4493 CrossRef CAS.
- M. J. Ruedas-Rama, J. D. Walters, A. Orte and E. A. H. Hall, Anal. Chim. Acta, 2012, 751, 1–23 CrossRef CAS PubMed.
- P. Reineck and B. C. Gibson, Adv. Opt. Mater., 2017, 5, 1600446 CrossRef.
- N. Singh, S. Charan, K. Sanjiv, S.-H. Huang, Y.-C. Hsiao, C.-W. Kuo, F.-C. Chien, T.-C. Lee and P. Chen, Bioconjugate Chem., 2012, 23, 421–430 CrossRef CAS PubMed.
- X. Yu, M. Li, M. Xie, L. Chen, Y. Li and Q. Wang, Nano Res., 2010, 3, 51–60 CrossRef CAS.
- K. Das, S. Sarkar and P. K. Das, ACS Appl. Mater. Interfaces, 2016, 8, 25691–25701 CrossRef CAS.
- S. Chinnathambi, S. Chen, S. Ganesan and N. Hanagata, Adv. Healthcare Mater., 2014, 3, 10–29 CrossRef CAS.
- F. Tang, C. Wang, X. Wang and L. Li, ACS Appl. Mater. Interfaces, 2015, 7, 25077–25083 CrossRef CAS PubMed.
- G. Huang, X. Zhu, H. Li, L. Wang, X. Chi, J. Chen, X. Wang, Z. Chen and J. Gao, Nanoscale, 2015, 7, 2667–2675 RSC.
- Y. P. Sun, B. Zhou, Y. Lin, W. Wang, K. A. S. Fernando, P. Pathak, M. J. Meziani, B. A. Harruff, X. Wang, H. Wang, P. G. Luo, H. Yang, M. E. Kose, B. Chen, L. M. Veca and S. Y. Xie, J. Am. Chem. Soc., 2006, 128, 7756–7757 CrossRef CAS PubMed.
- R. Bardhan, S. Lal, A. Joshi and N. J. Halas, Acc. Chem. Res., 2011, 44, 936–946 CrossRef CAS PubMed.
- F. Zou, H. Zhou, T. Van Tan, J. Kim, K. Koh and J. Lee, ACS Appl. Mater. Interfaces, 2015, 7, 12168–12175 CrossRef CAS.
- S. Sudhasree, A. Shakila Banu, P. Brindha and G. A. Kurian, Toxicol. Environ. Chem., 2014, 96, 743–754 CrossRef CAS.
- M. Abudayyak, E. Guzel and G. Özhan, Neurochem. Int., 2017, 108, 7–14 CrossRef CAS PubMed.
- L. De Los Santos Valladares, A. Ionescu, S. Holmes, C. H. W. Barnes, A. Bustamante Domínguez, O. Avalos Quispe, J. C. González, S. Milana, M. Barbone, A. C. Ferrari, H. Ramos and Y. Majima, J. Vac. Sci. Technol., B: Nanotechnol. Microelectron.: Mater., Process., Meas., Phenom., 2014, 32, 051808 Search PubMed.
- M. Marciuš, M. Ristić, M. Ivanda and S. Musić, J. Alloys Compd., 2012, 541, 238–243 CrossRef.
- A. C. Gandhi, J. Pant, S. D. Pandit, S. K. Dalimbkar, T.-S. Chan, C.-L. Cheng, Y.-R. Ma and S. Y. Wu, J. Phys. Chem. C, 2013, 117, 18666–18674 CrossRef CAS.
- S. Chinnakkannu Vijayakumar, K. Venkatakrishnan and B. Tan, ACS Appl. Mater. Interfaces, 2017, 9, 5077–5091 CrossRef CAS PubMed.
- S. Liu, S. C. Lee, U. M. Patil, C. Ray, K. V. Sankar, K. Zhang, A. Kundu, S. Kang, J. H. Park and S. Chan Jun, J. Mater. Chem. A, 2017, 5, 4543–4549 RSC.
- W. Zhen, J. Ma and G. Lu, Appl. Catal., B, 2016, 190, 12–25 CrossRef CAS.
- S. Sarkar, A. K. Sinha, M. Pradhan, M. Basu, Y. Negishi and T. Pal, J. Phys. Chem. C, 2011, 115, 1659–1673 CrossRef CAS.
- F. T. Thema, E. Manikandan, A. Gurib-Fakim and M. Maaza, J. Alloys Compd., 2016, 657, 655–661 CrossRef CAS.
- A. Das, A. C. Mandal, S. Roy and P. M. G. Nambissan, J. Exp. Nanosci., 2015, 10, 622–639 CrossRef CAS.
- M. Patel, J. S. Kim, B. S. Kim, Y. H. Kim and J. Kim, Mater. Lett., 2018, 218, 123–126 CrossRef CAS.
- H. Weizhao, Z. Yansong, L. Chade, H. Zhonghui and C. Gang, ACS Sustainable Chem. Eng., 2018, 6, 889 CrossRef.
- M. El-Kemary, N. Nagy and I. El-Mehasseb, Mater. Sci. Semicond. Process., 2013, 16, 1747–1752 CrossRef CAS.
- N. Mironova-Ulmane, A. Kuzmin, I. Steins, J. Grabis, I. Sildos and M. Pärs, J. Phys.: Conf. Ser., 2007, 93, 012039 CrossRef.
- A. C. Gandhi, H.-Y. Cheng, Y.-M. Chang and J. G. Lin, Mater. Res. Express, 2016, 3, 035017 CrossRef.
- N. D. Klein, K. R. Hurley, Z. V. Feng and C. L. Haynes, Anal. Chem., 2015, 87, 4356–4362 CrossRef CAS PubMed.
- M. M. Yallapu, S. F. Othman, E. T. Curtis, N. A. Bauer, N. Chauhan, D. Kumar, M. Jaggi and S. C. Chauhan, Int. J. Nanomed., 2012, 7, 1761–1779 CAS.
- A. Albanese, C. D. Walkey, J. B. Olsen, H. Guo, A. Emili and W. C. W. Chan, ACS Nano, 2014, 8, 5515–5526 CrossRef CAS PubMed.
- B. D. Chithrani, A. A. Ghazani and W. C. W. Chan, Nano Lett., 2006, 6, 662–668 CrossRef CAS PubMed.
- S. Behzadi, V. Serpooshan, W. Tao, M. A. Hamaly, M. Y. Alkawareek, E. C. Dreaden, D. Brown, A. M. Alkilany, O. C. Farokhzad and M. Mahmoudi, Chem. Soc. Rev., 2017, 46, 4218–4244 RSC.
- F. X. Gu, R. Karnik, A. Z. Wang, F. Alexis, E. Levy-nissenbaum, S. Hong, R. S. Langer and O. C. Farokhzad, Nano Today, 2007, 2, 14–21 CrossRef.
- N. Oh and J. H. Park, Int. J. Nanomed., 2014, 9, 51–63 Search PubMed.
- S. R. Elkin, N. Bendris, C. R. Reis, Y. Zhou, Y. Xie, K. E. Huffman, J. D. Minna and S. L. Schmid, Cancer Res., 2015, 75, 4640–4650 CrossRef CAS.
- H. T. McMahon and E. Boucrot, Nat. Rev. Mol. Cell Biol., 2011, 12, 517–533 CrossRef CAS PubMed.
- B. D. Chithrani and W. C. W. Chan, Nano Lett., 2007, 7, 1542–1550 CrossRef CAS PubMed.
- M. M. Yallapu, S. F. Othman, E. T. Curtis, B. K. Gupta, M. Jaggi and S. C. Chauhan, Biomaterials, 2011, 32, 1890–1905 CrossRef CAS PubMed.
- K. Sugawa, T. Tamura, H. Tahara, D. Yamaguchi, T. Akiyama, J. Otsuki, Y. Kusaka, N. Fukuda and H. Ushijima, ACS Nano, 2013, 7, 9997–10010 CrossRef CAS PubMed.
- J. Chen, H. Jiang, H. Zhou, Z. Hu, N. Niu, S. A. Shahzad and C. Yu, Chem. Commun., 2017, 53, 2398–2401 RSC.
- P. Khandelwal and P. Poddar, J. Mater. Chem. B, 2017, 5, 9055–9084 RSC.
- B. Liu and J. Liu, ACS Appl. Mater. Interfaces, 2015, 7, 24833–24838 CrossRef CAS.
- A. Muñoz and M. Costa, Toxicol. Appl. Pharmacol., 2012, 260, 1–16 CrossRef PubMed.
- N. L. Chaves, I. Estrela-Lopis, J. Böttner, C. Lopes, B. C. Guido, A. R. De Sousa and S. N. Báo, Int. J. Nanomed., 2017, 12, 5511–5523 CrossRef CAS PubMed.
- T. Dos Santos, J. Varela, I. Lynch, A. Salvati and K. A. Dawson, Small, 2011, 7, 3341–3349 CrossRef CAS PubMed.
- J. Voigt, J. Christensen and V. P. Shastri, Proc. Natl. Acad. Sci. U. S. A., 2014, 111, 2942–2947 CrossRef CAS.
- L. Yaqi, X. Chaoran, Z. Xiangmei, L. Chenshi, Y. Xin, X. Xiaofei, Z. Li, Q. Chao, H. Xiaopeng, Y. Lei, H. Wei and Y. Lifang, ACS Nano, 2018, 12, 1519 CrossRef.
- A. A. Bhirde, V. Patel, J. Gavard, G. Zhang, A. A. Sousa, A. Masedunskas, R. D. Leapman, R. Weigert, J. S. Gutkind and J. F. Rusling, ACS Nano, 2009, 3, 307–316 CrossRef CAS.
- M. Z. Hossain and M. Kleve, Int. J. Nanomed., 2011, 6, 1475 CrossRef CAS.
- Y. Wang, S. Wang, L. Jia, L. Zhang, J. Ba, D. Han, C. Yu and Y. Wu, Int. J. Environ. Res. Public Health, 2016, 13, 629 CrossRef.
- J. Pan, Q. Chang, X. Wang, Y. Son, Z. Zhang, G. Chen, J. Luo, Y. Bi, F. Chen and X. Shi, Chem. Res. Toxicol., 2010, 23, 568–577 Search PubMed.
- M. Ahamed, D. Ali, H. A. Alhadlaq and M. J. Akhtar, Chemosphere, 2013, 93, 2514–2522 CrossRef CAS.
Footnote |
† Electronic supplementary information (ESI) available: Fig. S1: White light emission at primary and secondary theranosomes, along with a representative picture of broadband emission. Fig. S2: (A) Fluorescence intensity of NIH3T3, HeLa and MDAMB-231 cells upon native controls. All the images are set to a standard scale of 10 μm. (B) Cell viability of theranosomes is established using model cancerous (MDAMB-231) and non-cancerous (NIH3T3) cells. Fig. S3: Stained and unstained controls for ROS and apoptosis assessment. Fig. S4: SEM and fluorescence images of NIH3T3, HeLa and MDAMB-231 cells upon native controls. All the SEM images are set to a standard scale of 10 μm. See DOI: 10.1039/c8nh00287h |
|
This journal is © The Royal Society of Chemistry 2019 |